-
PDF
- Split View
-
Views
-
Cite
Cite
Hiroki Murakami, Takashi Nobusawa, Koichi Hori, Mie Shimojima, Hiroyuki Ohta, Betaine Lipid Is Crucial for Adapting to Low Temperature and Phosphate Deficiency in Nannochloropsis , Plant Physiology, Volume 177, Issue 1, May 2018, Pages 181–193, https://doi.org/10.1104/pp.17.01573
- Share Icon Share
Abstract
Diacylglyceryl-N,N,N-trimethylhomo-Ser (DGTS) is a nonphosphorous, polar glycerolipid that is regarded as analogous to the phosphatidylcholine in bacteria, fungi, algae, and basal land plants. In some species of algae, including the stramenopile microalga Nannochloropsis oceanica, DGTS contains an abundance of eicosapentaenoic acid (EPA), which is relatively scarce in phosphatidylcholine, implying that DGTS has a unique physiological role. In this study, we addressed the role of DGTS in N. oceanica. We identified two DGTS biosynthetic enzymes that have distinct domain configurations compared to previously identified DGTS synthases. Mutants lacking DGTS showed growth retardation under phosphate starvation, demonstrating a pivotal role for DGTS in the adaptation to this condition. Under normal conditions, DGTS deficiency led to an increase in the relative amount of monogalactosyldiacylglycerol, a major plastid membrane lipid with high EPA content, whereas excessive production of DGTS induced by gene overexpression led to a decrease in monogalactosyldiacylglycerol. Meanwhile, lipid analysis of partial phospholipid-deficient mutants revealed a role for phosphatidylcholine and phosphatidylethanolamine in EPA biosynthesis. These results suggest that DGTS and monogalactosyldiacylglycerol may constitute the two major pools of EPA in extraplastidic and plastidic membranes, partially competing to acquire EPA or its precursors derived from phospholipids. The mutant lacking DGTS also displayed impaired growth and a lower proportion of EPA in extraplastidic compartments at low temperatures. Our results indicate that DGTS is involved in the adaptation to low temperatures through a mechanism that is distinct from the DGTS-dependent adaptation to phosphate starvation in N. oceanica.
Betaine lipids (BLs) are a family of glycerolipids characterized by a nonphosphorous, zwitterionic, polar head group and an ether bond connecting the head group with a diacylglycerol (DAG) backbone. To date, three types of BL have been identified in various organisms: diacylglyceryl-N,N,N-trimethylhomo-Ser (DGTS), diacylglyceryl-hydroxymethyl-N,N,N-trimethyl-β-Ala, and diacylglyceryl-carboxyhydroxymethylcholine (Dembitsky, 1996; Cañavate et al., 2016). Although information about the occurrence of the latter two BLs is relatively scarce and has only been described in several taxonomic groups of algae, DGTS is widely distributed across multiple kingdoms such as Bacteria, Protozoa, Chromista, Fungi, and Plantae (Sato and Furuya, 1985; Kato et al., 1996; Künzler and Eichenberger, 1997; Rozentsvet et al., 2000; Shemi et al., 2016; Cañavate et al., 2016, 2017). The amount of BL in cellular membranes can vary both within a species and among species. In general, limited to no BLs are produced by various species under optimal culture conditions, whereas BLs accumulate in response to phosphate (Pi) starvation (Benning et al., 1995; Geiger et al., 1999; Khozin-Goldberg and Cohen, 2006; Van Mooy et al., 2009; Geske et al., 2013; Riekhof et al., 2014; Abida et al., 2015; Senik et al., 2015; Shemi et al., 2016). Because Pi starvation also triggers phospholipid degradation, it has been proposed that the increase in BLs may complement the reduction in phospholipids, thereby reallocating Pi use from membrane lipid synthesis to other metabolic pathways. One remarkable case involves the green alga Chlamydomonas reinhardtii, which intrinsically lacks phosphatidylcholine (PC), a major phospholipid class in most eukaryotes, and instead produces a substantial amount of DGTS regardless of Pi concentration (Giroud et al., 1988). In organisms like C. reinhardtii, DGTS seems to replace PC constitutively rather than facultatively. The complementary association between BLs and phospholipids has long been accepted as an adaptive strategy to cope with periodic Pi deficiency in the natural environment. In certain algae species, however, BLs seem to have a distinct role aside from substituting for phospholipids since the fatty acid profile of BLs is dissimilar to that of any of the phospholipids, since it contains higher unsaturated groups (Iwai et al., 2015; Cañavate et al., 2016; Dolch et al., 2017; Nobusawa et al., 2017). Moreover, the degree of change in cellular BLs and PC contents upon fluctuations in external Pi availability is quite different among species (Cañavate et al., 2017). These species-dependent differences in BLs fatty acid profiles and susceptibility to low-Pi stress imply metabolic diversity among algae, as well as versatility in BLs function and requirement under Pi-starved conditions, yet we lack a comprehensive understanding of BLs functions (Cañavate et al., 2016).
DGTS is the sole BLs for which the biosynthetic pathway, precursors, and enzymes have been identified. DGTS biosynthesis is achieved through two main steps: attachment of a C4 homo-Ser moiety to a DAG backbone followed by the consecutive addition of three methyl groups to its amino group (Moore et al., 2001; Hofmann and Eichenberger, 1996; Fig. 1A). In prokaryotes, these first- and second-step reactions are catalyzed by BtaA and BtaB, respectively, whereas in eukaryotes, betaine lipid synthase 1 (BTA1), which contains both BtaA- and BtaB-like domains, carries out the entire process of DGTS biosynthesis (Klug and Benning, 2001; Riekhof et al., 2005; Fig. 1, A and B). The gene encoding BTA1 was initially identified in C. reinhardtii, and subsequent work identified putative BTA1 orthologs in several DGTS-producing fungi (Riekhof et al., 2005, 2014; Senik et al., 2015).
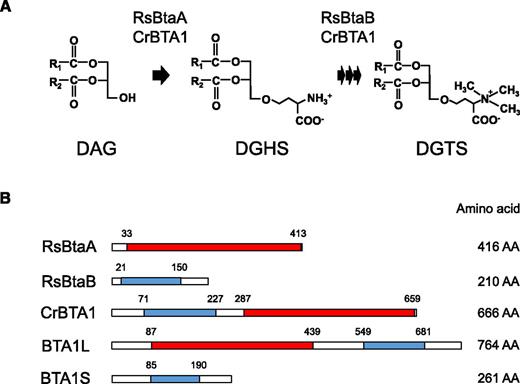
Biosynthetic pathway for DGTS. A, DGTS is synthesized from DAG via two step reactions. DGHS, Diacylglycerylhomo-Ser. B, Biosynthetic enzymes for DGTS with their catalytic domains. RsBtaA and the C-terminal domain of CrBTA1 catalyze the first step, and RsBtaB and the N-terminal domain of CrBTA1 catalyze the second step. Domains of 3-amino-3-carboxypropyltransferase and N-methyltransferase predicted with Pfam 30.0 are indicated by red and blue boxes, respectively. The start and end point of the domains are indicated above each construct, and the total number of amino acid residues (AA) is shown to the right. Rs, Rhodoobacter sphaeroides; Cr, Chlamydomonas reinhardtii.
Nannochloropsis is a genus of microalgae that belongs to the stramenopiles (also referred to as heterokonta) and possesses a secondary plastid derived from a red alga. Nannochloropsis species are capable of accumulating a large amounts of neutral lipids (i.e. triacylglycerol) and eicosapentaenoic acid (or 20:5, number of carbons:number of double bonds) and thus have received much interest as a biological source for these compounds (Mühlroth et al., 2013; Slocombe et al., 2015; Ma et al., 2016). These Nannochloropsis species traits could be applied to the production of specialty fatty acids that are not synthesized by native strains but are valuable for industrial or pharmaceutical use. To exploit the ability of Nannochloropsis species to generate specialty lipids, a great deal of effort has been devoted toward omics studies such as genome sequencing, as well as transcriptomic and lipidomic analyses (Radakovits et al., 2012; Vieler et al., 2012; Wang et al., 2014; Li et al., 2014; Poliner et al., 2015; Alboresi et al., 2016). Moreover, the development of molecular biology tools that enable the generation of Nannochloropsis transformants is increasing (Kilian et al., 2011; Wang et al., 2016; Wei et al., 2017). The coordination of omics data and functional analyses of key genes related to lipid biosynthesis would yield important information on algal lipid metabolism that is expected to form the basis for future metabolic engineering techniques (Dolch et al., 2017; Nobusawa et al., 2017; Zienkiewicz et al., 2017).
Our previous study revealed that Pi starvation increases the DGTS content of the Nannochloropsis species Nannochloropsis oceanica (strain NIES-2145; Iwai et al., 2015). Additionally, several recent reports demonstrated that DGTS is highly enriched in 20:5 fatty acids, which are minor components of PC in Nannochloropsis (Iwai et al., 2015; Cañavate et al., 2016; Dolch et al., 2017; Nobusawa et al., 2017). To investigate the physiological role of DGTS in N. oceanica, we characterized two DGTS biosynthetic enzymes and generated DGTS-lacking mutants, DGTS-overproducing lines, and phospholipid-reducing mutants. Our results suggest that DGTS is required not only for normal cell proliferation under Pi-starved conditions, but also for adaptation to low temperatures.
RESULTS
BTA1 Homologs in N. oceanica, Other Algae, and Land Plants
First, we searched our RNA sequencing data set (Nobusawa et al., 2017) for DGTS biosynthetic enzymes in N. oceanica strain NIES-2145. Two proteins had high amino acid sequence similarity to C. reinhardtii BTA1 (Riekhof et al., 2005) and were designated BTA1L (764 amino acid residues) and BTA1S (261 residues; Fig. 1B). The domain configurations of these proteins were distinct from that of CrBTA1. BTA1L has a reverse domain order, with a BtaA-like domain at the N terminus and a BtaB-like domain at the C terminus, and BTA1S contains only a domain homologous to the N terminus of CrBTA1 and thus is similar to the bacterial BtaB (Fig. 1B). Previous work identified binding sites for the substrate S-adenosyl-Met in both the BtaA-like and BtaB-like domains of CrBTA1 and shows that these binding sites are necessary for enzyme activity (Riekhof et al., 2005). We therefore investigated whether the Val-Asp motif in the substrate binding site is present in N. oceanica BTA1L and BTA1S and found that BTA1L has this motif in both the N- and C-terminal domains, whereas the Val in the motif is replaced with Leu in BTA1S (Supplemental Fig. S1, A and B). Given that BtaB from Rhodoobacter sphaeroides, which can synthesize DGTS together with BtaA during Pi deficiency, encodes Leu at this locus (Supplemental Fig. S1B), BTA1S may also play a role in DGTS biosynthesis. Thus, N. oceanica harbors two proteins, one of which has a complete set of putative catalytic domains that could possibly mediate DGTS biosynthesis, whereas the other only has a BtaB-like N-methyltransferase domain (Fig. 1B).
Having observed a domain-reversed type of BTA1 in N. oceanica, we sought BTA1L homologs in other algae and land plants using available databases. We found BTA1L-like proteins in prasinophyte green algae, chlorarachniophyte algae, and several species of Chromista and Alveolata; the latter two are clades possessing a red alga-derived secondary plastid (Supplemental Fig. S1C). On the other hand, putative orthologs of CrBTA1 were detected in viridiplantae (a clade including green algae and land plants) except for prasinophyceae and seed plants (Supplemental Fig. S1C). Phylogenetic analysis of the BTA1L-like and CrBTA1-like proteins revealed that these algal and plant putative BTA1 proteins could be separated into two subgroups (types A and B) according to their domain arrangements (Supplemental Fig. S2). In addition, we found BTA1S-like proteins in some species of streptophyta, such as Klebsormidium nitens (formerly identified as Klebsormidium flaccidum; Hori et al., 2014; Ohtaka et al., 2017), Marchantia polymorpha, Physcomitrella patens, and Selaginella moellendorffii, all of which have type A BTA1, but not in the majority of the stramenopile algae, which have type B BTA1 proteins (Supplemental Fig. S1C).
Pi Starvation Upregulates BTA1L and BTA1S Expression and Enhances DGTS Accumulation
Our previous study revealed that the DGTS level increases in N. oceanica in response to Pi starvation (Iwai et al., 2015). Under Pi-starved conditions in Gram-negative bacteria and fungi, DGTS biosynthesis is tightly controlled by a transcription factor (Geiger et al., 1999; Riekhof et al., 2014). To determine whether Pi starvation-induced DGTS accumulation in N. oceanica is also transcriptionally regulated, we quantified expression of BTA1L and BTA1S in N. oceanica grown under Pi-depleted, Pi-replete, or other stress conditions (nitrogen starvation and low temperature). First, we confirmed that the Pi depletion conditions indeed induced DGTS accumulation (Fig. 2A; Supplemental Fig. S3). As we describe below in detail, cold treatment also increased the relative proportion of DGTS, albeit to a lower extent than Pi starvation (Fig. 2A). Reverse transcription quantitative PCR (RT-qPCR) revealed that BTA1L and BTA1S were significantly upregulated by a factor of 2 during Pi starvation compared with normal Pi-replete conditions (Fig. 2, B and C). This result indicated that the increase in DGTS level observed in Pi-starved N. oceanica is also transcriptionally regulated. Because we could not identify an apparent ortholog of Pho4p, a known transcriptional activator of BTA1 in fungi, the factor(s) responsible for induction of these genes remains unknown.
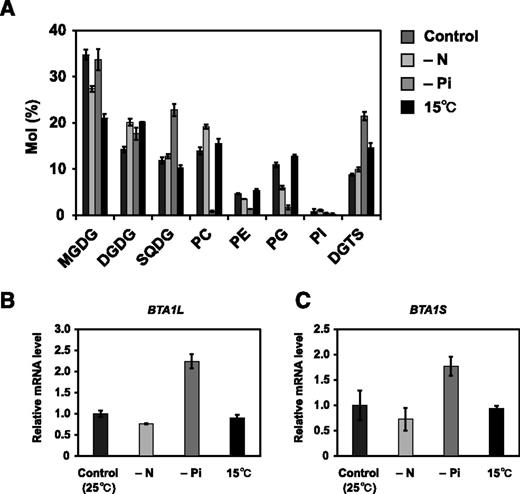
Changes in membrane lipid composition relative expression level of BTA1L and BTA1S genes under stress. A, Membrane lipid composition of N. oceanica wild-type strain grown under normal, nitrogen-starved, Pi-starved, or 15°C conditions for 3 d. B and C, Relative expression of BTA1L (B) and BTA1S (C) under the four conditions. Expression was normalized to that of the gene encoding NADH dehydrogenase subunit 11. Data represent the means ± sd of three biologically independent experiments. PG, Phosphatidylglycerol; PI, phosphatidylinositol; SQDG, sulfoquinovosyldiacylglycerol.
BTA1L and BTA1S Are DGTS Synthases
To obtain evidence that BTA1L and BTA1S are bona fide DGTS biosynthetic enzymes, we generated N. oceanica knockout mutants of BTA1L and BTA1S by homologous recombination and assessed their capacity to synthesize DGTS (Supplemental Fig. S4). One-dimensional thin-layer chromatography (TLC) was used for isolating DGTS from the total lipid fraction. With our TLC solvent system, DGTS separated into three spots probably because of differences in the content of 20:5 fatty acids (Supplemental Fig. S5). These three spots were absent in the total lipid fraction of the bta1l mutants, demonstrating that BTA1L is essential for DGTS biosynthesis (Figure 3). Conversely, the bta1s mutants did not show any defect in DGTS content even when grown under Pi-deficient conditions, which has the effect of up-regulating BTA1S expression and cellular DGTS levels (Supplemental Fig. S6). This result suggested that BTA1S either has no relevance to DGTS biosynthesis or has a similar function to that of the C-terminal domain of BTA1L, which compensates for the absence of BTA1S. To determine whether BTA1S is actually involved in DGTS biosynthesis in vivo, we constructed a mutant lacking only the C-terminal domain of BTA1L by incorporating a C-terminal domain-truncated BTA1L (BTA1LƊC) into bta1l and subsequently examining the ability of the complemented mutant to synthesize DGTS (Supplemental Fig. S7). Because we could not knockout BTA1S from bta1l;BTA1LƊC, BTA1LƊC was also introduced into the bta1s;bta1l double mutant as a negative control. bta1s;bta1l was generated by BTA1L knockout of the bta1s mutant background. As a positive control, the bta1s;bta1l mutant was complemented with full-length BTA1L. As shown in Figure 3, DGTS was detected in bta1l;BTA1LƊC and bta1s;bta1l;BTA1L but not in bta1s;bta1l and bta1s;bta1l;BTA1LƊC. To exclude the possibility that inadequate induction of BTA1LƊC in bta1s;bta1l;BTA1LƊC caused the lack of DGTS production, we compared the gene expression level of transformed BTA1LƊC or BTA1L between bta1l;BTA1LƊC, bta1s;bta1l;BTA1LƊC, and bta1s;bta1l;BTA1L. There was no apparent reduction in the BTA1LƊC transcript level in bta1s;bta1l;BTA1LƊC (Supplemental Fig. S8). These data indicated that either BTA1S or the C-terminal domain of BTA1L is necessary for DGTS biosynthesis; thus, BTA1S can function in DGTS synthesis in N. oceanica.
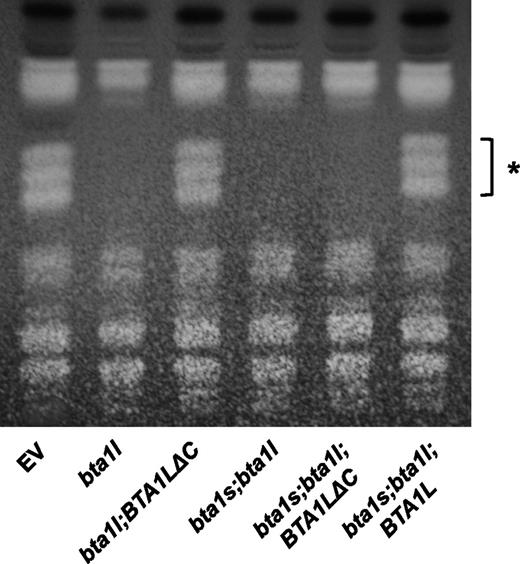
DGTS synthesis in bta1l and the BTA1L- or BTA1LƊC-complemented mutant as assessed with TLC. Total lipid of EV, bta1l, bta1l;BTA1LƊC, bta1s;bta1l, bta1s;bta1l;BTA1LƊC, and bta1s;bta1l;BTA1L cultivated under Pi-starved conditions for 4 d were separated by one-dimensional TLC using solvent system chloroform/methanol/acetic acid/water (170:30:15:3, v/v/v/v). The asterisk denotes spots of DGTS.
Under Pi Starvation, Proliferation of bta1l Mutants Is Retarded and the MGDG Level Is Elevated
Next, we cultured the bta1l, bta1s, and bta1s;bta1l mutants in either Pi-sufficient or Pi-deficient medium to examine whether the lack of DGTS affects cell proliferation and the membrane lipid profile. As shown in Figure 4, A and B, the proliferation of bta1l cells was comparable to that of the empty-vector (EV) control strain during exponential growth under Pi-sufficient conditions, whereas proliferation was impaired under Pi-deficient conditions. This result demonstrated the importance of DGTS for proper growth under Pi starvation. Unexpectedly, the cell density of bta1s;bta1l was reduced under normal conditions (Fig. 4A). We then analyzed the membrane lipid composition of bta1l and bta1s;bta1l grown under Pi-replete conditions. DGTS was not detected in bta1l or bta1s;bta1l (Fig. 4C), as was shown in Figure 3. Mutant bta1l exhibited an increase in the relative proportion of monogalactosyldiacylglycerol (MGDG; a major lipid of the chloroplast membrane) and phosphatidylethanolamine (PE), whereas bta1s;bta1l had an increased proportion of digalactosyldiacylglycerol (DGDG), PC, and PE compared with EV (Fig. 4C). Fatty acid composition of each lipid class was also altered in these mutants (Supplemental Fig. S9): in bta1s;bta1l, the proportion of 20:5 decreased in MGDG but increased in DGDG (Supplemental Fig. S10, A and B). Both bta1l and bta1s;bta1l had more 18:1, 18:2, and 20:4 but less 16:1 and 16:2 in PC, whereas they had more 20:4 but less 16:0, 16:1, 16:2, and 18:2 in PE (Supplemental Fig. S9, E and F). These observations indicated that defects in the DGTS biosynthetic pathway affected the profiles of both plastidic and extraplastidic membrane lipids, even under conditions that did not impair cell proliferation.
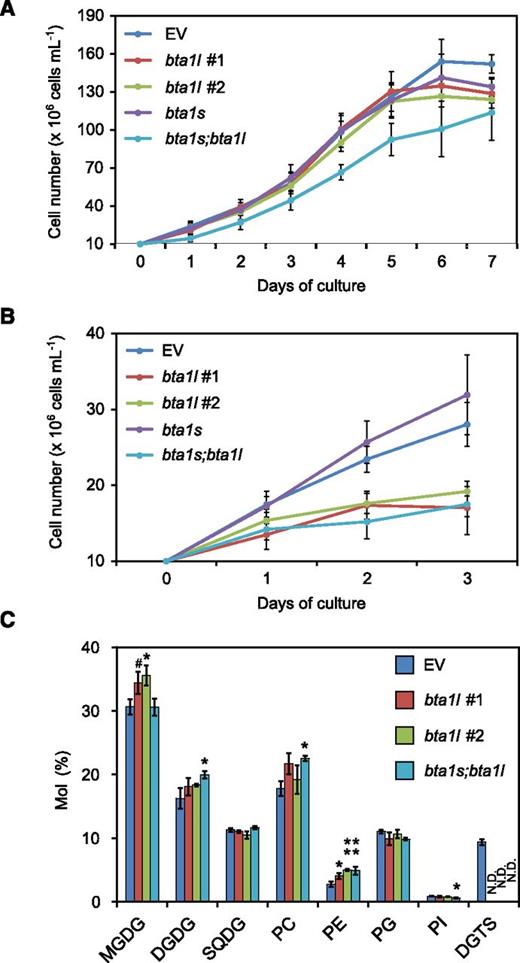
Phenotypes of mutants bta1l, bta1s, and bta1s;bta1l. A and B, Growth curve for EV, bta1l, bta1s, and bta1s;bta1l under Pi-sufficient (A) and Pi-deficient (B) conditions. Each cell culture was diluted with medium to an initial density of 107cells mL–1. Data represent the mean ± sd of four (A) and three (B) biologically independent experiments. C, Comparison of relative polar lipid composition. The EV line (control) and mutant lines were cultivated under normal conditions for 3 d and then harvested for lipid analysis. Data represent the mean ± sd of three biologically independent experiments. Statistical significance was determined with Dunnett’s test (compared with EV): #, P < 0.1; *, P < 0.05; **, P < 0.01. SQDG, Sulfoquinovosyldiacylglycerol; PG, phosphatidylglycerol; PI, phosphatidylinositol; N.D., not detected.
Overexpression of BTA1L Leads to an Increase in DGTS and a Decrease in MGDG
We overexpressed BTA1L in N. oceanica to overproduce DGTS under normal culture conditions and analyzed the effect this had on membrane lipid composition (Supplemental Fig. S10). BTA1L was expressed under the control of the violaxanthin-chlorophyll a binding protein 1 (VCP1) promoter, which facilitates potent expression under normal growth conditions (Kilian et al., 2011). We introduced the gene construct into N. oceanica and obtained an overexpression line (OEBTA1L) that had ∼10-fold higher expression than the control strain (Supplemental Fig. S10). Consistent with this, the proportion of DGTS in the polar lipid fraction increased by ∼40% in OEBTA1L compared with EV (Fig. 5A). Conversely, OEBTA1L had a smaller proportion of MGDG and phosphatidylglycerol but exhibited no significant change in the proportion of PC (Fig. 4A; Klug and Benning, 2001; Riekhof et al., 2014). The distribution of 20:5 within the major classes of membrane lipid was also altered in OEBTA1L: this ratio was higher in DGTS but lower in MGDG (Fig. 5E). Because DGTS is enriched in 20:5 (Supplemental Fig. S9H), we initially expected that the overproduction of DGTS led to an overall increase in 20:5 content. However, the total 20:5 content was not greater in OEBTA1L (EV, 19.4 ± 1.78 µg L–1; OEBTA1L, 20.1 ± 0.979 µg L–1; n = 3), probably owing to the reduction in the relative amount of 20:5 in DGTS (Fig. 5B). Thus, although DGTS has an abundance of 20:5, enhancing DGTS biosynthesis alone may not be useful for increasing the 20:5 content in N. oceanica.
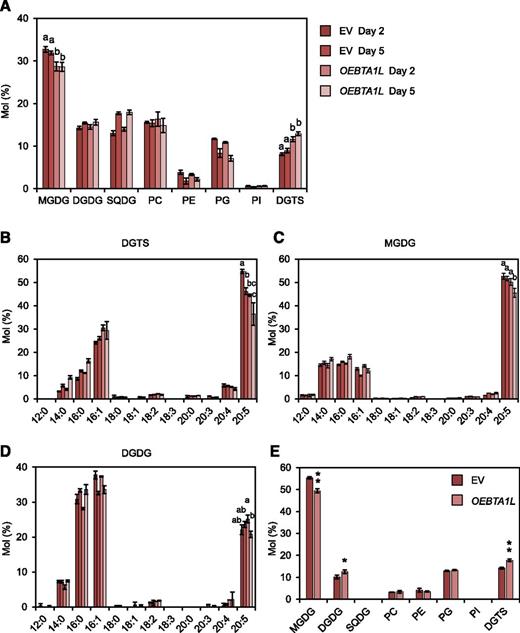
Membrane lipid analysis of the BTA1L overexpression line. A, Comparison of membrane lipid composition between EV and OEBTA1L. B to D, Fatty acid profiles for DGTS (B), MGDG (C), and DGDG (D). Data represent the mean ± sd of three biologically independent experiments. Statistical significance was determined with Tukey’s test and is indicated by letters at the top. E, Distribution of 20:5 within the eight classes of membrane lipid after 2 d of culture. Data represent the mean ± sd of three biologically independent experiments. Statistical significance was determined with the two-tailed Student’s t test: *, P < 0.05; **, P < 0.01. SQDG, Sulfoquinovosyldiacylglycerol; PG, phosphatidylglycerol; PI, phosphatidylinositol.
The Proportion of 20:5 in Galactolipids Is Significantly Reduced in cct1 and pect1 Mutants
In Nannochloropsis, de novo fatty acid synthesis is considered to occur inside chloroplasts, whereas biosynthetic processes of 20:5, namely, desaturation and elongation of long-chain fatty acids, proceed at the endoplasmic reticulum (ER) membrane (Guschina and Harwood, 2006; Dolch et al., 2017). ER-localized PC and PE have been proposed to contribute to 20:5 biosynthesis because these two phospholipids harbor mainly desaturated fatty acids of lengths C18 (PC) and C20 (PE; Schneider and Roessler, 1994; Kaye et al., 2015). To gain further insight into the metabolic function of membrane lipids, we aimed to generate mutants that lack either PC or PE. To this end, we targeted CTP:phosphocholine cytidylyltransferase 1 (CCT1) and CTP:phosphoethanolamine cytidylyltransferase 1 (PECT1), which catalyze the respective rate-limiting step for PC and PE biosynthesis (Inatsugi et al., 2009; Mizoi et al., 2006). For N. oceanica sp NIES-2145, CCT1 and PECT1 were each predicted to be encoded by single genes based on a sequence similarity search, and a knockout strain was produced for each gene (cct1 and pect1; Supplemental Fig. S11). As shown in Figure 6A, under normal culture conditions, we observed a 44% reduction of PC in cct1 and a 43% reduction of PE in pect1, suggesting redundant function for these enzymes that precluded complete depletion of PC or PE in each single mutant. Under the same conditions, the cct1 mutant had a higher proportion of DGTS (+24%) and phosphatidylglycerol (+14%) compared with the EV control, whereas the membrane lipid profile was not significantly altered in pect1 (Fig. 6A). Moreover, cct1 had smaller relative amount of 14:0, 16:1, and 20:5 and a greater amount of 20:4 in PC, whereas pect1 had a significantly smaller proportion of 16:1 in PE (Fig. 6, B and C). In DGTS, cct1 had a greater proportion of 14:0, 16:0, 18:2, and 20:4 at the expense of 20:5 (Fig. 6F). Importantly, the proportion of 20:5 in MGDG and DGDG was reduced by ∼ 10% in both cct1 and pect1, indicating the importance of PC and PE in producing the 20:5 that is incorporated into the chloroplast membrane (Fig. 6, B and C).
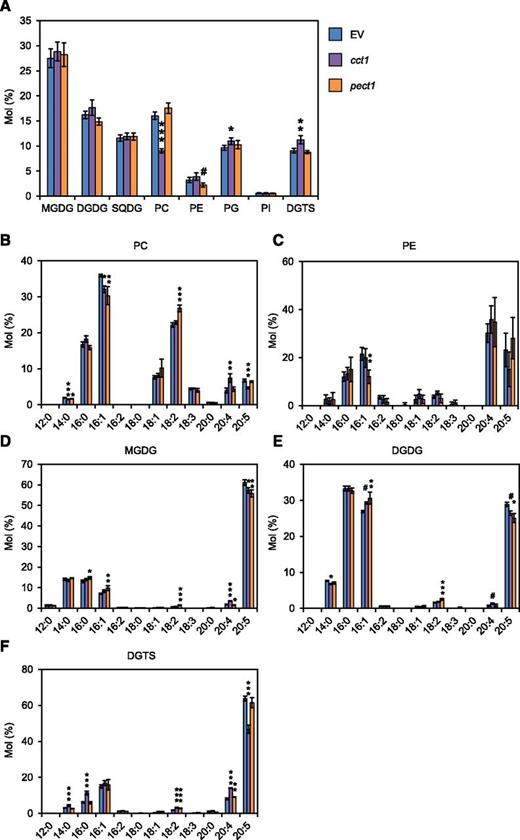
Membrane lipid composition of mutants cct1 and pect1 grown under normal conditions. A, Membrane lipid composition compared with EV (control). EV and mutant lines were cultured for 3 d under normal conditions before lipid analysis. B to F, Fatty acid profile of PC (B), PE (C), MGDG (D), DGDG (E), and DGTS (F) in EV, cct1, and pect1. Data represent the mean ± sd of four biologically independent experiments. Statistical significance was determined with Dunnett’s test (compared with EV): #, P < 0.1; *, P < 0.05; **, P < 0.01; ***, P < 0.001. SQDG, Sulfoquinovosyldiacylglycerol; PG, phosphatidylglycerol; PI, phosphatidylinositol.
Impaired Growth of bta1l at Low Temperatures
Growth at low temperatures has been shown to facilitate the accumulation of 20:5 in Nannochloropsis (Hu and Gao, 2006; Hoffmann et al., 2010), yet how polar lipid composition changes has not been described in detail. We therefore cultured the N. oceanica wild-type strain at 15°C and assessed its lipid composition. The proportions of DGDG, phosphatidylglycerol, and DGTS increased by up to 42, 17, and 66%, respectively, at the expense of MGDG (Fig. 2A, Supplemental Fig. S3). Unlike Pi starvation, the low temperature caused an increase in DGTS content without stimulating the expression of BTA1L and BTA1S (Fig. 2, B and C). Next, the bta1l, cct1, and pect1 mutants were exposed to a low temperature (15°C) to clarify which lipid is important for adaptation to low temperature and how membrane lipid composition changes when either of DGTS, PC, or PE is deficient. The results revealed that only bta1l had a significantly lower cell density (Fig. 7A). We then analyzed the lipid composition of these mutants and found that bta1l had no DGTS but had an increased proportion of MGDG, PC, and PE, compared with the EV control (Fig. 7B). With regards to fatty acid composition of each lipid class in bta1l, we observed a marked increase of 20:5 in PC and PE (Fig. 7, C and D). Nevertheless, the proportion of 20:5 in the bta1l extraplastidic membranes was significantly smaller compared with that measured for EV (Fig. 7F). We excluded phosphatidylglycerol from this evaluation because it is known to exist in both plastidic and extraplastidic compartments (Babiychuk et al., 2003). These bta1l phenotypes indicated that DGTS is required for maintaining both optimal growth and a large amount of 20:5 in extraplastidic membranes at low temperatures. The cct1 mutant produced less than half of the PC and had a larger proportion of DGTS, sulfoquinovosyldiacylglycerol, and PE than the EV control (Fig. 7B). Unexpectedly, the amount of PE in pect1 was comparable to that in the EV control (Fig. 7B), possibly owing to activation of CCT1 at low temperature, as reported in Arabidopsis (Arabidopsis thaliana; Inatsugi et al., 2009). Consistent with this result, pect1 did not show any significant difference in the proportion of other membrane lipid or fatty acid profiles of each lipid class compared with EV (Fig. 7, B–E).
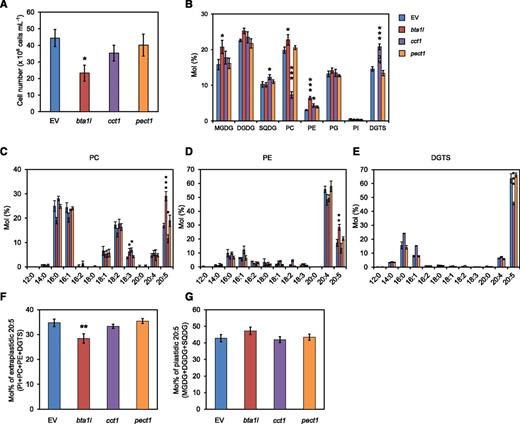
Phenotypes of the mutants bta1l, cct1, and pect1 grown at low temperature. A, Number of cells for EV and the indicated mutants after 3 d of culture. B to E, Comparison of relative polar lipid composition (B) and fatty acid profile of PC (C), PE (D), and DGTS (E) in EV, bta1l, cct1, and pect1. F and G, Molar ratio of 20:5 in extraplastidic (F) and plastidic (G) membrane lipids. Data represent the mean ± sd of three biologically independent experiments. Statistical significance was determined with Dunnett’s test (compared with EV): *, P < 0.05; **, P < 0.01; ***, P < 0.001. SQDG, Sulfoquinovosyldiacylglycerol; PG, phosphatidylglycerol; PI, phosphatidylinositol.
DISCUSSION
Although genes for DGTS biosynthesis are widely found in many algae and basal land plants (Supplemental Figs. S1 and S2), previous studies on these DGTS-related genes have been limited in some species such as C. reinhardtii and R. sphaeroides (Klug and Benning, 2001; Riekhof et al., 2005). In this work, we investigated the functions of the DGTS biosynthetic enzymes and the DGTS product via identification of two BTA1 homologs in the oleaginous alga N. oceanica. BTA1L has a reversed domain structure compared with that of a previously reported BTA1 (Riekhof et al., 2005, 2014; Senik et al., 2015), with putative orthologs in several species of secondary algae and prasinophyte algae (Fig. 1B; Supplemental Figs. S1C and S2). The BTA1L-like proteins form a clade that is phylogenetically distinct from the conventional type BTA1 proteins that are mainly distributed in viridiplantae (Supplemental Fig. S2). These observations indicate the existence of two types of bifunctional BTA1-encoding genes that have been inherited through different lineages in algae and plants (types A and B; see Supplemental Fig. S2). We also discovered that BTA1S is a homolog of the bacterial BtaB enzyme, as determined by its involvement in DGTS biosynthesis in N. oceanica (Figs. 1B and 3; Supplemental Fig. S7). BTA1S-like proteins were also found in streptophyta (Supplemental Fig. S1C). Because these distant organisms harbor a common set of genes, the bifunctional BTA1-encoding gene and the gene encoding a BtaB-like N-methyltransferase, BTA1S and its homologs may play specific roles in DGTS biosynthesis and/or other unknown processes that could not be accomplished by the bifunctional BTA1 alone. Consistent with this speculation, bta1s;bta1l had retarded growth compared with bta1l under normal conditions (Fig. 4A), even though DGTS was equally deficient in both mutants (Fig. 3). We initially proposed that BTA1S may be implicated in betaine (Gly betaine) synthesis because BTA1 is a BL synthase. Supplementation with Gly betaine, however, could not complement the growth deficiency of bta1s;bta1l. The catalytic reaction mediated by BTA1S may be reminiscent of the biosynthetic pathways of PC, which comprise triple methylation of PE or phosphoethanolamine (Sato et al., 2016; Hirashima et al., 2017). However, because the PC content was not reduced in bta1s;bta1l (Fig. 4C), it is unlikely that BTA1S contributes to PC formation. Although this study could not reveal BTA1S function, future analyses will elucidate the complete functions of Nannochloropsis BTA1 homologs.
We generated DGTS-lacking mutants to obtain new insight into the physiological role of DGTS in N. oceanica. The growth of bta1l was not impaired under normal conditions, whereas it was strongly arrested under Pi starvation and also at low temperatures (Figs. 4A, 4B, and 7A). Given that the wild-type strain accumulated a larger amount of DGTS in response to Pi starvation and low temperature, DGTS appears to have an essential role in the adaptation to these stresses (Fig. 2A). As many previous studies have described, DGTS would be expected to be crucial for adaptation to Pi-starved conditions as an alternative polar lipid to phospholipids (Van Mooy et al., 2009; Riekhof et al., 2014; Cañavate et al., 2017). On the other hand, we propose two possibilities that explain why DGTS is required for optimal cell proliferation at low temperatures. First, DGTS may be important as a store for 20:5 in extraplastidic membranes. Polyunsaturated fatty acids that are esterified to membrane lipids contribute to the maintenance of membrane fluidity, especially at low temperature (Murata and Los, 1997). Recently, Zorin et al. (2017) suggested that arachidonic acid (20:4)-containing MGDG might contribute to sustaining chloroplast membrane fluidity at low temperatures in the green algae Lobosphaera incisa. Our results indicate that, at low temperature, DGTS is naturally enriched in 20:5 and that DGTS deficiency leads to a smaller proportion of 20:5 in extraplastidic compartments compared with the EV control (Supplemental Figs. S3 and S9; Fig. 7F). Moreover, no other lipid class located in extraplastidic membranes contains as much 20:5 as DGTS. We also analyzed fatty acid composition of triacylglycerol in the EV control and the bta1 mutant and found very low levels of 20:5 with no large difference between these two lines. Therefore, we have omitted the idea that the neutral lipid has a great influence on the metabolism related to 20:5. In N. oceanica, DGTS may have a role in retaining 20:5; therefore, its relative deficiency affects the amount of 20:5, and hence the functionality, of the plasma membrane and other extraplastidic membranes, leading to reduced proliferation at 15°C. Second, the polar head group of DGTS, which is relatively large and thus may help maintain membrane-bilayer homeostasis even at low temperature, may be important for adapting to temperature changes.
By characterizing compositional changes in membrane lipids in mutants lacking DGTS, mutants overproducing DGTS, and phospholipid-reducing mutants, we attempted to elucidate the mechanism of lipid metabolism in N. oceanica. Lipid analyses in bta1l and OEBTA1L suggested a negative correlation between the amount of DGTS and MGDG (Figs. 4C and 5A). This trend was also reported in Chlorella minutissima (Haigh et al., 1996): DGTS and MGDG levels fluctuated periodically with a reciprocal relationship, leading to the speculation that DGTS might be a donor of 20:5 for chloroplast MGDG, another major pool of 20:5. Likewise, a suite of experiments using chemical inhibitors and low-Pi stress suggested that DGTS provides 20:5 for the sn-1 positions of galactolipids in the eustigmatophyte Monodus subterraneus (Khozin-Goldberg et al., 2002; Khozin-Goldberg and Cohen, 2006). However, our results suggest that DGTS is not crucial for delivering 20:5 from the ER to chloroplast membranes because bta1l did not show a reduction in 20:5 of galactolipids, nor galactolipids themselves, and conversely OEBTA1L had a reduced level of MGDG (Figs. 4C and 5A; Supplemental Fig. S9, A and B). DGTS and MGDG may rather compete for the two major 20:5 destinations in extraplastidic and plastidic membranes, for which fatty acids are produced via the catabolism of phospholipids (Fig. 8). This hypothesis would also explain the mechanism by which DGTS levels increased during low temperatures compared with normal conditions in the wild type; since MGDG levels dropped under low temperature in the EV, and the DGTS-lacking mutant had more MGDG at low temperatures than the EV, the accumulation of DGTS may be caused by the repression of MGDG synthesis. In this scenario, it is not activation of the DGTS synthetic enzymes but oversupplementation of substrates that would lead to enhanced DGTS levels. Alternatively, because the subcellular compartment in which DGTS and MGDG exists has yet to be defined in secondary symbiotic algae, it may be that DGTS and MGDG localize to the same place, e.g. the chloroplast envelope membrane, and act complementarily to each other, as do DGTS and PC in extraplastidic membranes. Notably, in secondary symbiotic algae, the chloroplast is enclosed by three or four envelopes, and the outermost envelope is continuous with the ER membrane (Murakami and Hashimoto, 2009). This fact may underlie intermixed membrane lipid composition in the chloroplast envelope derived from these two organelles, and a more sophisticated fractionation method must be developed to unequivocally determine the membrane lipid profile at the subcellular level.
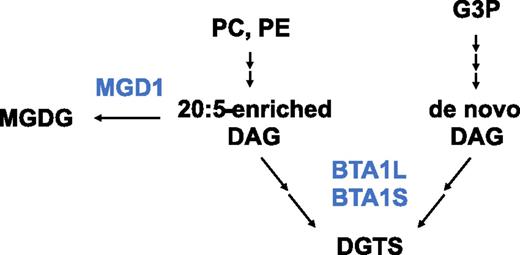
Schematic model for the DGTS biosynthesis in Nannochloropsis. We proposed that 20:5-containing DGTS and 20:5-free DGTS are formed by different pathways with different physiological relevance. The 20:5-enriched DAG derived from phospholipids would diverge to use for DGTS or MGDG biosynthesis catalyzed by BTA1L and BTA1S or MGD1, respectively. Blue represents enzymes and black represents metabolites.G3P, Glycerol 3-phosphate; MGD1, MGDG synthase 1.
In the case of secondary algae including N. oceanica, their genomes generally lack any TRIGALACTOSYLDIACYLGLYCEROL1-5 homologs, which mainly mediate the eukaryotic pathway in Arabidopsis (Li et al., 2016; Hori et al., 2016). This means that the principle for the DAG supplying pathway to galactolipids synthesis in higher plants would not be directly applied to their metabolism. However, elongation of 16:0 and desaturation of the elongated very-long-chain fatty acids, specifically formation of 20:5, mainly occurs in the ER not in plastids in Nannochloropsis (Schneider and Roessler, 1994; Dolch et al., 2017), suggesting that extensive lipid transport from the ER to the plastid must take place. This lipid transfer route is discerned from the eukaryotic pathway and is rather termed the omega pathway, while its detail is unresolved (Petroutsos et al., 2014). Our phenotypic analyses of cct1 and pect1 suggested that 20:5 are mainly produced through PC and PE and then transferred to plastid galactolipids, without being mediated by DGTS, and to DGTS and other lipids. We observed a lower occupancy of 20:5 in the galactolipids of cct1 and pect1 under normal conditions (Fig. 6, D and E). In cct1, DGTS also had a decreased proportion of 20:5, although this may be partly attributable to the relative increase in 20:5-free DGTS (Fig. 6F). These results suggest that PC and PE are important for producing the 20:5 used for the formation of the chloroplast membrane, and these roles cannot be fully compensated by excess DGTS. Because there was only a moderate reduction in 20:5 in cct1 and pect1 under normal conditions, the possibility remains that DGTS partly supports the metabolic roles of these phospholipids. We note that, at low temperatures, cct1 did not have a decreased level of plastidic 20:5 even though the amount of PC was less than half (Fig. 7B). This result suggests the possibility that DGTS metabolically compensated for the PC deficiency or that even a small amount of PC was sufficient for synthesizing a comparable amount of 20:5 in the wild-type strain, at least under these conditions.
Despite the widespread distribution of BLs among phytoplankton, understanding their defined roles in cellular physiology has been limited mostly to the condition of Pi starvation. This study reveals two distinct roles for DGTS in N. oceanica, namely, that DGTS is not only important as a surrogate lipid of phospholipids during Pi starvation but also required for adaptation to low temperatures. Furthermore, our results provide information about the mechanism of membrane lipid metabolism in N. oceanica. Although the microalgal lineage exhibits vast metabolic diversity, we speculate that the characteristics of DGTS may be applicable to other organisms, especially species that are taxonomically close to Nannochloropsis or algae that inhabit low Pi and/or low-temperature environments. Future detailed lipid analyses of algae and plants will help to clarify the diversity of roles played by BLs in algal homeostasis.
MATERIALS AND METHODS
Nannochloropsis oceanica Strains and Culture Conditions
The N. oceanica strain NIES-2145 was obtained from the National Institute for Environmental Studies. For the “normal” condition, cells were cultured in a 50-mL volume at 25°C in F2N medium (Kilian et al., 2011) with 36 g L–1 artificial seawater (Wako Pure Chemical Industries) in a NEG test tube (Nichiden-Rika Glass) with continuous bubbling of 2% CO2 and constant light (40 µmol photons m–2 s–1). Before each experiment, the cells were precultured for 3 d under normal conditions starting from a density of 107 cells mL–1. Cell density was measured using a bacteria counter (Sunlead Glass) with an optical microscope. To induce nitrogen or Pi starvation, cells were washed twice with nitrogen- or phosphorus-free F2N medium (cells were collected at 1,500g for 7 min and resuspended in the respective medium) as described by Iwai et al. (2015). A growth chamber (LH-80LED-DT; Nippon Medical & Chemical Instruments) was used for low-temperature experiments.
Phylogenetic Analysis
Amino acid sequences of putative BTA1 homologs from algae and plants were retrieved from available databases and were aligned using MAFFT v7.245 (Katoh and Standley, 2013). Regions of low sequence conservation were eliminated with the Gblocks server version 0.91b (Talavera and Castresana, 2007). The resultant alignment was subjected to phylogenetic analysis with MEGA 6.06 (Tamura et al., 2013) using the maximum-likelihood method with the amino acid substitution model of LG model + Gamma (eight categories).
Plasmid Construction and Nuclear Transformation
The NT7 gene cassette (Kilian et al., 2011), which consists of the VCP2 promoter (ProVCP2), the Streptoalloteichus hindustanus bleomycin resistance gene (Sh ble), and the VCP1 terminator (terVCP1), was constructed between the PstI and KpnI sites of the pUC18 vector (reverse complement direction) as described by Nobusawa et al. (2017). Sh ble was replaced with the Streptomyces hygroscopicus aminoglycoside phosphotransferase gene (Aph7) or Streptomyces rimosus aminoglycoside 3-phosphotransferase gene (Aph8) to confer hygromycin B or paromomycin resistance, respectively. For making gene knockout constructs, ∼1 kb of the 5′ and 3′ flanking genomic regions of the relevant gene were amplified by PCR using N. oceanica genomic DNA as a template and were cloned into the PstI and KpnI sites of vector pUC18/NT7, respectively. The sequence of the flanking region was obtained from genome information of N. oceanica IMET1 (Wang et al., 2014). For gene overexpression and complementation, the promoter sequence (∼1 kb) of VCP1 (for overexpression) or lipid droplet surface protein gene (for complementation) with a NotI site were cloned between Sh ble (or Aph7 or Aph8) and terVCP1 of pUC18/NT7 by inverse PCR. BTA1L (2,295 bp) and BTA1LƊC (1,335 bp with a termination codon) coding sequences were PCR amplified using a cDNA template and were cloned into the NotI site (ProVCP2:sh ble:ProVCP1:gene:terVCP1). Information about primers used for plasmid construction is provided in Supplemental Table S1. Linearized DNA fragments amplified from each construct and M13 forward (5′-GTAAAACGACGGCCAGT-3′) and M13 reverse (5′-CAGGAAACAGCTATGAC-3′) primers were used for nuclear transformation. Genetic transformation experiments were conducted essentially according to Kilian et al. (2011). Cells at the exponential phase of growth were centrifuged (4,900g, 7 min) and washed twice with 375 mm sorbitol (Wako Pure Chemical Industries). The pellet was resuspended in a small amount of sorbitol, and 100 µL of the resultant cell suspension and 3 to 5 µg linearized DNA were added to a 2-mm cuvette (Bio-Rad) and then employed for electroporation performed with a Gene Pulser II (Bio-Rad). The electroporation conditions were 2.2 kV, 50 µF, and 600 Ohm. Electroporated cells were immediately transferred to a culture tube with 5 mL F2N medium and then were shaken for 48 h under continuous light. The cells were collected by centrifugation and resuspended in 5 mL melted top agar (f/2 medium with 0.4% Bacto agar [Difco] and 18 g L–1 seawater). Cell suspensions were sowed on f/2 agar plates (f/2 medium with 0.8% agar and 18 g L–1 seawater) containing 2 µg mL–1 zeocin (Thermo Fisher Scientific), 350 µg mL–1 hygromycin B (Wako Pure Chemical Industries), or 150 µg mL–1 paromomycin (Sigma-Aldrich), depending on the antibiotic resistance gene used for transformation. After single colonies became visible, each line was seeded into a 96-well plate filled with F2N medium. Genomic DNA was isolated as described by Nobusawa et al. (2017) and used for PCR-based screening with the primers shown in Supplemental Table S1.
RNA Preparation and RT-qPCR
Total RNA was prepared using the TRI reagent (Sigma-Aldrich). First-strand cDNA was synthesized using Superscript II reverse transcriptase (Invitrogen). PCR products were used to confirm gene knockouts and for quantification of relative mRNA levels. Semiquantitative PCR was carried out with the primers described in Supplemental Table S1. For quantitative PCR, cDNA was amplified with SYBR Premix Ex Taq II (Takara), and the signal was detected with the Thermal Cycler Dice Real-Time System (Takara). Delta Ct values were calculated based on the Ct value for the gene encoding NADH dehydrogenase subunit 11. RT-qPCR was carried out with the primers shown in Supplemental Table S1.
Lipid Analyses
Total cellular lipids were extracted according to Bligh and Dyer (1959). Polar lipids were separated by two-dimensional TLC using TLC silica plates (Merck), the solvent system chloroform/methanol/7 n ammonia water (120:80:8, v/v/v) as the first dimension, and chloroform/methanol/acetic acid/water (170:30:15:3, v/v/v/v) as the second dimension. To visualize each lipid class spot, each developed TLC plate was sprayed with 0.01% (w/v) primuline in 80% (v/v) acetone and then irradiated with black light (352 nm). Each lipid spot was scraped from the TLC plate, and 100 µL of 1 mm pentadecanoic acid was added as an internal standard. Each suspension was then incubated with 5% (v/v) HCl methanol solution (Supelco) at 85°C for 1 h for conversion into the corresponding fatty acid methyl ester. The methyl esters were then extracted with hexane and quantified via gas chromatography with a flame ionization detector (Shimadzu) with a HR-SS-10 capillary column (Shinwa Chemical Industries).
Accession Numbers
Sequence data of Nannochloropsis protein identified from this article can be found in the DNA Databank of Japan (http://www.ddbj.nig.ac.jp) with the following accession numbers: BTA1S (LC375791), BTA1L (LC375792), PECT1 (LC375793), and CCT1 (LC375794). The accession numbers used for the phylogenetic analysis were as follows: Selaginella moellendorffii (XP_002980648), Physcomitrella patens (XP_001757434), Marchantia polymorpha (OAE21146), Klebsormidium nitens (GAQ92949), Coccomyxa subellipsoidea (XP_005649296), Chlorella variabilis NC64A (XP_005844132), Volvox carteri (XP_002955110), Chlamydomonas reinhardtii (XP_001700879), Micromonas pusilla (XP_003063768), Ostreococcus lucimarinus (XP_001421536), Bigelowiella natans (JGI Protein ID 89413), Emiliania huxleyi (XP_005763111), Thalassiosira oceanica (EJK50635), Fragilariopsis cylindrus (OEU16585), Phaeodactylum tricornutum (XP_002176772), Guillardia theta (XP_005822595), and Vitrella brassicaformis (CEM35387).
Supplemental Data
The following supplemental materials are available.
Supplemental Figure S1. Predicted substrate-binding sites for BTA1L and BTA1S and putative BTA1 homologs found in algae and plants.
Supplemental Figure S2. Phylogenetic tree for the putative BTA1 homologs found in plants and algae.
Supplemental Figure S3. Fatty acid composition of each lipid class of the wild type grown under normal, -N, -Pi, and low-temperature conditions.
Supplemental Figure S4. Generation of knockout mutants of BTA1L and BTA1S via homologous recombination.
Supplemental Figure S5. Fatty acid composition of three TLC spots corresponding to molecular variants of DGTS.
Supplemental Figure S6. Membrane lipid composition of bta1s mutants grown under Pi-sufficient or -deficient conditions.
Supplemental Figure S7. Complementation with full length and C-terminally truncated BTA1L in mutants bta1l and bta1s;bta1l.
Supplemental Figure S8. Comparison of expression level of N-terminal domain of BTA1L.
Supplemental Figure S9. Relative fatty acid composition of each lipid class of bta1l and bta1s;bta1l.
Supplemental Figure S10. Relative expression of BTA1L in the overexpression mutant.
Supplemental Figure S11. Generation of mutants cct1 and pect1.
Supplemental Table S1. List of sequences of primers used in this study.
ACKNOWLEDGMENTS
N. oceanica strain NIES-2145 was obtained from the National Institute for Environmental Studies in Japan.
LITERATURE CITED
Author notes
This work was supported by CREST (Core Research for Evolutional Science and Technology) and OPERA (Program on Open Innovation Platform with Enterprises, Research Institute and Academia) of the Japan Science and Technology Agency.
Address correspondence to [email protected].
The author responsible for distribution of materials integral to the findings presented in this article in accordance with the policy described in the Instructions for Authors (www.plantphysiol.org) is: Hiroyuki Ohta ([email protected]).
H.M. and H.O. conceived the original research plans; H.M. performed the experiments and in silico analysis; T.N. provided technical assistance to H.M.; H.M., T.N., and H.O. analyzed the data; H.M. wrote the manuscript with contributions from all authors.
Articles can be viewed without a subscription.