-
PDF
- Split View
-
Views
-
Cite
Cite
Xu Liu, Yuhua Yang, Yilong Hu, Limeng Zhou, Yuge Li, Xingliang Hou, Temporal-Specific Interaction of NF-YC and CURLY LEAF during the Floral Transition Regulates Flowering, Plant Physiology, Volume 177, Issue 1, May 2018, Pages 105–114, https://doi.org/10.1104/pp.18.00296
- Share Icon Share
Abstract
The flowering time of higher plants is controlled by environmental cues and intrinsic signals. In Arabidopsis (Arabidopsis thaliana), flowering is accelerated by exposure to long-day conditions via the key photoperiod-induced factor FLOWERING LOCUS T (FT). Nuclear Factor-Y subunit C (NF-YC) proteins function as important mediators of epigenetic marks in different plant developmental stages and play an important role in the regulation of FT transcription, but the mechanistic details of this remain unknown. In this study, we show that Arabidopsis NF-YC homologs temporally interact with the histone methyltransferase CURLY LEAF (CLF) during the flowering transition. The binding of NF-YC antagonizes the association of CLF with chromatin and the CLF-dependent deposition of H3 lysine-27 trimethylation, thus relieving the repression of FT transcription and facilitating flowering under long-day conditions. Our findings reveal a novel mechanism of NF-YC/CLF-mediated epigenetic regulation of FT activation in photoperiod-induced flowering and, consequently, contribute to our understanding of how plants control developmental events in a temporal-specific regulatory manner.
Flowering is precisely regulated by environmental and endogenous signals (Andrés and Coupland, 2012). In Arabidopsis (Arabidopsis thaliana), a facultative long-day (LD) plant, flowering signals are perceived and transmitted by different molecular pathways, including photoperiod, vernalization, thermosensory, aging, autonomous, and GA pathways, which mostly converge to regulate the key flowering integrator gene FLOWERING LOCUS T (FT). FT, the long-sought florigen, functions as a long-distance signal to the shoot apical meristem, where it diurnally binds to FD for transcriptional activation of floral meristem identity genes (Kardailsky et al., 1999; Kobayashi et al., 1999; Abe et al., 2005; Wigge et al., 2005; Corbesier et al., 2007). In LD conditions, the main photoperiod-responsive regulator CONSTANS (CO) promotes flowering by directly activating the FT and another floral integrator gene, SUPPRESSOR OF OVEREXPRESSION OF CO1 (SOC1; Putterill et al., 1995; Lee et al., 2000; Samach et al., 2000). In addition, FT also could be regulated by important regulators involved in other flowering pathways, such as FLOWERING LOCUS C (FLC), FLOWERING LOCUS M variants, and PHYTOCHROME INTERACTING FACTOR4, etc., to modulate flowering time (Michaels and Amasino, 1999; Kumar et al., 2012; Posé et al., 2013). So far, recent findings have suggested that FT exerts a central function in the flowering transition.
Flowering is genetically controlled by various epigenetic factors that activate or repress the transcription of flowering genes (He, 2012). In Arabidopsis, the Polycomb Repressive Complex2 (PRC2) specifically mediates the histone H3 Lys-27 trimethylation (H3K27me3) that plays extensive regulatory roles in various developmental stages, including flowering (Holec and Berger, 2012). CURLY LEAF (CLF) is a conserved component in PRC2, and loss of function of CLF causes an early-flowering phenotype with curled leaves (Goodrich et al., 1997). Previous extensive evidence supported that FT up-regulation is fundamentally required for the early flowering of clf, and CLF retains FT repression through promoting the H3K27me3 deposition in FT chromatin (Jiang et al., 2008; Adrian et al., 2010).
Nuclear Factor-Y subunit C (NF-YC), also termed Histone-Associated Protein5 (HAP5) and CCAAT Binding Factor C (CBF-C), interacts with NF-YB (HAP3/CBF-A) to form a heterodimer analogous to the core histone 2A/2B dimer with the highly conserved histone fold domain. The NF-YC/YB dimer, in turn, unites with NF-YA (HAP2/CBF-B) to constitute the NF-Y heterotrimer that canonically binds to the CCAAT box in eukaryotic promoters (Huber et al., 2012; Nardini et al., 2013). In plants, NF-YC subunits are encoded by a multigene family and function as important participants in flowering time control (Petroni et al., 2012; Swain et al., 2017; Zhao et al., 2017). Arabidopsis NF-YC homologs have redundant roles in the FT activation of photoperiod-dependent flowering by contributing to forming the canonical NF-YC-YB-YA and specific NF-YC-YB-CO complexes, which recognize the distal CCAAT box enhancers and the proximal CO-responsive elements, respectively, within the FT promoter region (Kumimoto et al., 2010; Cao et al., 2014; Gnesutta et al., 2017). Recent studies have demonstrated that NF-Y factors recruit different histone modifiers to regulate various developments in plants (Hou et al., 2014; Tang et al., 2017). However, how NF-Y mediates the epigenetic regulation of FT transcription remains unknown.
In this study, we demonstrate a CLF-dependent epigenetic mechanism in the NF-YC-mediated FT transcription regulation in Arabidopsis. NF-YC homologs counteract the H3K27me3 deposition in FT chromatin by temporally interacting with CLF to attenuate the association of CLF with the FT locus, thus derepressing FT transcription under LD conditions. Collectively, our findings support that NF-YC proteins function as important modulators of epigenetic marks controlling flowering, promoting the understanding of how plants precisely control developmental events in a temporal-specific regulatory manner.
RESULTS
NF-YC Interacts with CLF
To further investigate the molecular roles of NF-YC proteins, we performed a yeast two-hybrid screening to identify the potential NF-YC-interacting epigenetic partners. Interestingly, CLF, the conserved histone methyltransferase of PRC2, was found to interact strongly with NF-YC3 and NF-YC9, and weakly with NF-YC4, in yeast (Fig. 1A). Then, the glutathione S-transferase (GST) pull-down assay showed that the recombinant proteins of His-NF-YC3, His-NF-YC4, and His-NF-YC9 were coprecipitated by GST-CLF fusion proteins but not by the GST control (Fig. 1B), indicating the physical interaction between CLF and NF-YC proteins in vitro. Since NF-YC9 could genetically replace the function of the other two homologs (Liu et al., 2016b; Tang et al., 2017), we chose NF-YC9 as the representative NF-YC gene for further investigation.
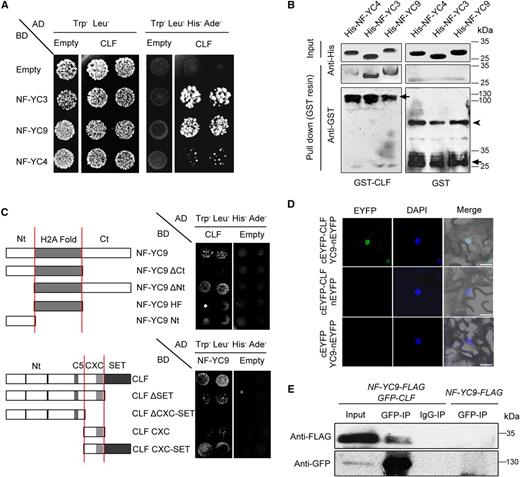
NF-YCs interact physically with CLF in vitro and in vivo. A, Yeast two-hybrid assays show the interactions between CLF and NF-YC proteins. Transformed yeast cells were grown on SD-Trp/-Leu/-His/-Ade or SD-Trp/-Leu medium. AD, Activation domain; BD, binding domain. B, Pull-down assays show the physical interaction between GST-CLF and His-NF-YC fusion proteins in vitro. His-NF-YC proteins were incubated with immobilized GST or GST-CLF, and immunoprecipitated fractions were detected by anti-His and anti-GST antibodies, respectively. Arrows indicate the specific bands of GST-CLF or GST; the arrowhead indicates the nonspecific bands. C, Domains required for the interaction of CLF and NF-YC proteins. Sketches show the domains of NF-YC9 and CLF and their various deletions. Yeast two-hybrid assays show the interactions between CLF, NF-YC9, and their derivatives. Transformed yeast cells were grown on SD-Trp/-Leu/-His/-Ade or SD-Trp/-Leu medium. D, BiFC analysis of the interaction between NF-YC9-nEYFP and cEYFP-CLF in tobacco leaf epidermal cells. The 4′,6-diamino-2-phenylindol (DAPI) staining indicates the nucleus. Bars = 20 μm. E, In vivo interaction of NF-YC9 and CLF in Arabidopsis. Plant nuclear extracts from 9-d-old clf nf-yc9 35S:GFP-CLF pNF-YC9:NF-YC9-FLAG seedlings under LD conditions were immunoprecipitated by either anti-GFP trap or preimmune serum (IgG). The coimmunoprecipitated proteins were detected by anti-FLAG and anti-GFP antibodies.
According to the conserved regulatory domains in NF-YC and CLF (Holec and Berger, 2012; Liu et al., 2016b), we designed various truncated versions of NF-YC9 and CLF to identify the necessary domains required for the protein interaction. This result indicates that the highly conserved CXC-SET domain of CLF and the C-terminal end of NF-YC9 contribute to the protein interaction (Fig. 1C). Bimolecular fluorescence complementation (BiFC) analysis was conducted in tobacco (Nicotiana tabacum) leaf epidermal cells to examine the interaction between NF-YC9 and CLF in vivo. The fluorescence by NF-YC9-nEYFP together with cEYFP-CLF was localized in the cell nuclei (Fig. 1D). Coimmunoprecipitation (Co-IP) analysis using the nuclear extracts of 9-d-old seedlings (clf-28 nf-yc9-1 35S:GFP-CLF pNF-YC9:NF-YC9-FLAG) further confirmed the binding of NF-YC9 to CLF in Arabidopsis (Fig. 1E). Taken together, these data support the direct interaction between NF-YC and CLF proteins in plants.
CLF Is Epistatic to NF-YC
As both CLF and NF-YC are involved in flowering control (Goodrich et al., 1997; Kumimoto et al., 2010; Hou et al., 2014), but with little mutual regulation regarding transcriptional levels (Supplemental Fig. S1), the interaction between CLF and NF-YC proteins led us to investigate whether these two proteins associate functionally to regulate flowering. We examined the genetic connection between NF-Y and CLF by introducing the nf-yc3-2 nf-yc4-1 nf-yc9-1 (nf-ycT) mutations into the clf-28 (clf) mutant background. Under LD conditions, the clf nf-ycT mutants exhibited similar early flowering and upward-curled leaves to clf mutants, whereas nf-ycT showed extreme late flowering, as reported previously (Fig. 2, A and B; Kumimoto et al., 2010). Meanwhile, mutation of CLF also rescued the late-flowering phenotype in loss-of-function mutants of NF-YBs (nf-yb2-1 nf-yb3-1), another subunit of NF-Y involved in flowering control (Supplemental Fig. S2). These observations indicate that the genetic function of CLF in flowering control is epistatic to NF-YC/B genes. To test this further, we examined the temporal expression of several flowering genes in various genetic backgrounds. Both FT and SOC1 were significantly up-regulated in the Col wild type during the floral transition under LD conditions. Remarkably, the daily expression of FT was down-regulated in nf-ycT but highly up-regulated in clf and clf nf-ycT compared with that in the wild type (Fig. 2C; Supplemental Fig. S3), supporting the epistatic role of CLF to NF-YC in FT expression. By contrast, SOC1 transcripts were decreased by nf-ycT in either the clf or CLF background, indicating that CLF does not mediate NF-YC-regulated SOC1 expression (Fig. 2C). In addition, CO, encoding the main regulator of FT transcription, was hardly regulated by NF-YC and CLF (Fig. 2C). FLC, SEP3, and AG genes are known as the direct downstream targets of CLF and are repressed by CLF in flowering (Schubert et al., 2006; Lopez-Vernaza et al., 2012). Our analysis showed that, compared with that in the wild type, the expression of these genes was increased remarkably in clf and clf nf-ycT but changed slightly (FLC) or not affected (SEP3 and AG) in the nf-ycT background (Supplemental Fig. S4), indicating that it is unlikely that NF-YC acts as the main regulator of the CLF targets FLC, SEP3, and AG. Therefore, these results suggest that CLF and NF-YC mediate flowering time primarily by regulating FT.
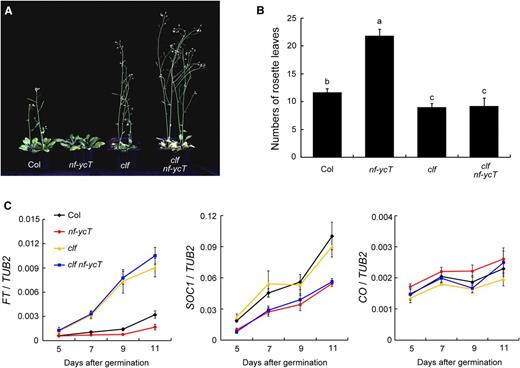
Genetic analysis of the interaction of CLF and NF-YC. A, Flowering phenotypes of Columbia (Col), clf, nf-ycT, and clf nf-ycT plants. The seedlings were grown under LD conditions at 22°C for 28 d and selected for photography. B, Flowering times of Col, clf, nf-ycT, and clf nf-ycT plants under LD conditions at 22°C. The number of rosette leaves in at least 10 plants at bolting was used as an indicator of flowering time. Statistically significant differences are indicated by different lowercase letters (one-way ANOVA, P < 0.05). C, Quantitative real-time PCR (qPCR) analysis of FT, SOC1, and CO temporal expression in developing Col, clf, nf-ycT, and clf nf-ycT seedlings. The seedlings were grown under LD conditions at 22°C and collected for RNA extraction at the 12th h in a 24-h period (16 h of light/8 h of dark). The TUBULIN2 gene (TUB2) was used as an internal control. Data represent means ± sd of biological triplicates.
NF-YC Mediates CLF-Dependent H3K27me3 Deposition
CLF functions in the trimethylation of H3K27 at chromatin of flowering genes (Goodrich et al., 1997; Schubert et al., 2006; Jiang et al., 2008). To learn whether NF-YC affects the epigenetic function of CLF, we examined the H3K27me3 levels at several selected FT loci in Col, nf-ycT, clf, and clf nf-ycT plants by chromatin immunoprecipitation (ChIP) assays (Fig. 3A). Notably, H3K27me3 marks in the FT locus were increased in nf-ycT, whereas the loss of CLF significantly reduced the levels of H3K27me3 in both the nf-ycT and wild-type backgrounds (Fig. 3B). Furthermore, we examined the deposition of H3K27me3 at the selected FLC, AG, and SOC1 loci. Consistent with the expression analysis, H3K27me3 levels in FLC and AG were attenuated in clf and clf nf-ycT (Figs. 2C and 3B). By contrast, loss of NF-YC had only a slight effect or no effect on H3K27me3 deposition in FLC and AG, respectively, but enhanced that in SOC1 (Fig. 3B). These results indicate that NF-YC may repress CLF-dependent H3K27me3 deposition to specifically regulate FT transcription.
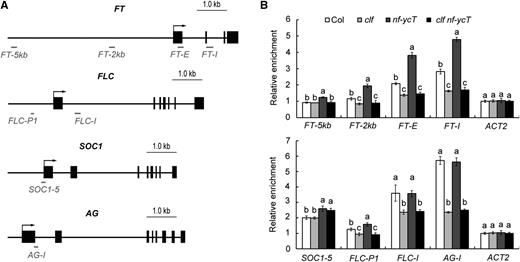
ChIP analysis of H3K27me3 levels in the relative flowering genes in various mutants. A, Schematic representation of the specific primer positions in the FT, FLC, SOC1, and AG loci used in B as well as in Figures 4B and 5A. Exons are represented by black rectangles and upstream regions and introns by black lines; the primer fragments used for amplification are indicated by gray lines. B, ChIP analysis of H3K27me3 levels of the relative flowering genes in Col, clf, nf-ycT, and clf nf-ycT plants. Plant nuclear proteins were extracted from 9-d-old seedlings under LD conditions. Relative fold enrichment values were calculated by normalizing the amount of a target DNA fragment against that of a genomic fragment of a reference gene, Cinful-like, and then against the respective input DNA samples. The enrichment of an ACTIN2 (ACT2) genomic fragment was used as the negative control. Data represent means ± sd of biological triplicates. Statistically significant differences between Col and mutants are indicated by different lowercase letters (one-way ANOVA, P < 0.05).
Loss of NF-YC Function Elevates CLF Enrichment and H3K27me3 Deposition on FT Chromatin
We created nf-ycT clf GFP-CLF by introducing the nf-ycT mutations into clf 35S:GFP-CLF plants. Under LD conditions, GFP-CLF could rescue the early-flowering phenotype of clf and nf-ycT clf GFP-CLF exhibited a late-flowering phenotype similar to that in nf-ycT (Fig. 4A), suggesting that CLF activity is required for late flowering caused by NF-YC loss of function. We next conducted ChIP assays to examine the association of GFP-CLF with target chromatin in clf GFP-CLF and nf-ycT clf GFP-CLF plants. NF-YC loss of function significantly promoted the binding of GFP-CLF to FT, which was concomitant with higher H3K27me3 levels of FT chromatin and lower FT expression in nf-ycT clf GFP-CLF compared with that in clf GFP-CLF plants (Fig. 4, B–D). By contrast, no difference was observed regarding GFP-CLF binding to SOC1 between clf GFP-CLF and nf-ycT clf GFP-CLF plants (Fig. 4, C and D). These results confirm the repressive role of NF-YC on CLF binding to FT chromatin.
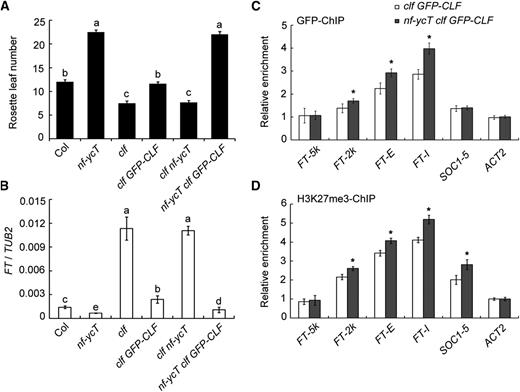
Analysis of NF-YC’s effect on the CLF enrichment and H3K27me3 deposition at FT loci. A, Late flowering caused by the loss of NF-YC genes requires CLF activity. At least 10 seedlings of Col, clf, nf-ycT, clf GFP-CLF, clf nf-ycT, and nf-ycT clf GFP-CLF plants grown under LD conditions at 22°C were investigated for flowering time determination. B, qPCR analysis of FT gene expression. Nine-day-old seedlings were grown under LD conditions at 22°C and collected for RNA extraction at the 12th h in a 24-h period (16 h of light/8 h of dark). C, ChIP analysis of CLF binding to the FT locus (amplicons defined in Fig. 3A). D, Analysis of H3K27me3 levels in FT chromatin. Nine-day-old clf 35S:GFP-CLF and nf-ycT clf 35S:GFP-CLF seedlings were grown under LD conditions at 22°C and collected for ChIP assay with anti-GFP and anti-H3K27me3 antibodies, respectively. Data represent means ± sd of biological triplicates. Statistically significant differences are indicated by different lowercase letters (one-way ANOVA, P < 0.05). Asterisks indicate significant changes of enrichment fold between two genetic backgrounds (Student’s t test, P < 0.05).
Temporal-Specific Interaction of NF-YC and CLF Regulates CLF-Dependent H3K27me3 Deposition in FT Chromatin
Generally, plants do not immediately enter the flowering transition without undergoing a period of vegetative development. Hence, we wondered how NF-YC represses CLF function via protein-protein interaction to facilitate flowering at the right time. To address this, the temporal pattern of the NF-YC-CLF interaction was investigated by a time-course ChIP analysis using developing clf GFP-CLF and nf-ycT clf GFP-CLF seedlings grown under LD conditions. As plant growth progressed, both CLF binding and H3K27me3 deposition in FT decreased gradually during the flowering transition stage (9–11 d after germination; Fig. 5A; Supplemental Fig. S5). It is worth noting that NF-YC loss of function significantly attenuated this decline (Fig. 5A). Furthermore, ChIP assay with a transient expression system showed that the binding of CLF to the FT region was decreased dramatically by the full-length NF-YC9 but not by the C-terminal-deleted NF-YC9, which fails to interact with CLF (Fig. 1C; Supplemental Fig. S6, A and B). These observations support that CLF function may be impaired by the enhanced interaction of CLF and NF-YC during the flowering transition stage. One possibility is that the varied interaction pattern could be triggered by the increasing abundance of NF-YC proteins during plant growth. However, we did not observe obvious increased NF-YC accumulation during the flowering transition stage by immunoblot analysis of clf nf-yc9 GFP-CLF NF-YC9-FLAG seedlings (Fig. 5B). In contrast to the decreased CLF binding and H3K27me3 levels (Fig. 5A), time-course Co-IP analysis showed an increasing interaction between CLF and NF-YC9 during the flowering transition (Fig. 5B), suggesting that other unknown factors determined by flowering signals might be involved in the temporal regulation of the NF-YC-CLF interaction.
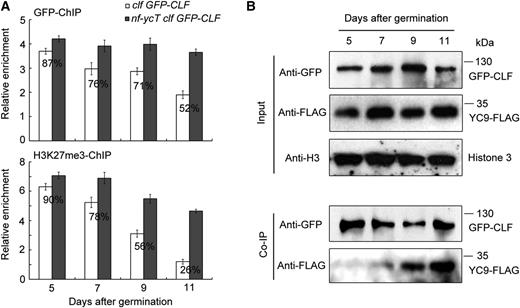
Temporal pattern analysis of NF-YC and CLF regulating CLF-dependent H3K27me3 at the FT locus. A, Time-course ChIP analysis of CLF binding (top) and H3K27me3 levels (bottom) in FT chromatin (amplicons defined in Fig. 3A). Seedlings of clf 35S:GFP-CLF and nf-ycT clf 35S:GFP-CLF were grown under LD conditions at 22°C and harvested for nuclear protein extraction at the indicated days. The percentages indicate the relative enrichment of the FT-I fragment in clf 35S:GFP-CLF against that in nf-ycT clf 35S:GFP-CLF (designated as 100%). B, Co-IP analysis of the temporal interaction between NF-YC9 and CLF in developing seedlings. clf nf-yc9 GFP-CLF NF-YC9-FLAG seedlings grown under LD conditions were harvested for nuclear protein extraction at the indicated days and then immunoprecipitated by anti-GFP trap. The coimmunoprecipitated proteins were detected by anti-FLAG and anti-GFP antibodies.
DISCUSSION
In yeast and mammals, NF-Y factors are deemed to make a functional link between chromatin and transcription (Dolfini et al., 2012). Our recent studies have revealed that NF-YC regulates plant developmental stages via mediating epigenetic modifications on target genes (Hou et al., 2014; Tang et al., 2017), implying an important function of NF-YC proteins in the epigenetic regulation in plants. In the photoperiod flowering pathway, NF-Y factors are crucial for the chromatin activation of FT, which is simultaneously controlled by various epigenetic regulations (Gu et al., 2013; Bu et al., 2014; Cao et al., 2014). However, whether and how NF-YC mediates the epigenetic regulation of FT transcription remain unknown. Here, we reveal a temporal interaction of NF-YC with the histone methyltransferase CLF that specifically counteracts CLF-dependent H3K27me3 deposition to derepress FT transcription, thus promoting flowering under LD conditions. These results illustrate a novel epigenetic regulatory model in the photoperiod flowering pathway and expand our understanding of how plants precisely control developmental events in a temporal-specific manner.
In eukaryotes, the PRC2 members are structurally and evolutionarily conserved, in which CLF orthologs function as the core component to catalyze H3K27 methylation. The conserved CXC and SET domains of CLF are necessary for H3K27 methyltransferase activity and sequence-specific binding (Cao et al., 2002; Müller et al., 2002; Ketel et al., 2005). For example, the gain-of-function allele clf-59, harboring a Pro-to-Ser amino acid mutation in the CXC domain, results in vernalization-independent early flowering in Arabidopsis (Doyle and Amasino, 2009). Like CLF, NF-YC, with a histone fold domain close to the core histone 2A, is widely conserved in eukaryotic species and functions as a subunit of NF-Y heterotrimers (Dolfini et al., 2012). In this study, the highly conserved CXC and SET domains of CLF and the C-terminal end of NF-YC9 contribute to their protein interaction (Fig. 1C), implying that the combination of CLF and NF-YC may frequently present and function in different eukaryotic species or may play distinct roles in various biological processes. Upon our observations, the binding of NF-YC to the CXC-SET domain of CLF impairs the DNA affinity of CLF, thus restricting CLF function to target chromatins. However, we cannot exclude the possibility that NF-YC might act as a potential catalyzed substrate of CLF to mediate unknown regulations.
FT functions as a major output of the photoperiod pathway regulator CO when plants respond to LD conditions (Andrés and Coupland, 2012). Recent studies have demonstrated that NF-Y factors are involved in the CO-FT regulatory cascade via direct interaction with CO (Kumimoto et al., 2010; Gnesutta et al., 2017). The identification of NF-Y-mediated chromatin looping at the FT locus and the novel sequence-specific NF-YB/YC/CO complex further support that CO regulating FT is dependent primarily on NF-Y(C) functions (Cao et al., 2014; Gnesutta et al., 2017). It was shown that CLF indirectly controls FT expression by epigenetically regulating FLC in different aspects, such as the autonomous pathway (Doyle and Amasino, 2009; Lopez-Vernaza et al., 2012; Müller-Xing et al., 2014). However, CLF could bind directly to FT chromatin and mediate H3K27me3 deposition in FT under LD conditions (Fig. 5A; Jiang et al., 2008; Adrian et al., 2010). Similar to clf nf-ycT, clf co double mutants also presented a clf-like early-flowering phenotype (Pazhouhandeh et al., 2011). Considering the biological interaction between NF-YC and CLF presented in this study, it is quite possible that the CO-NF-Y module regulates FT transcription by preventing CLF-dependent H3K27me3 deposition in FT chromatin. Consistently, clf could rescue the late-flowering phenotype caused by the loss of NF-YB2/3 function (Supplemental Fig. S2); however, the question of whether NF-YC represses CLF together with NF-YA/B and CO to facilitate flowering requires further investigation.
Besides FT, SOC1 acts as another important integrator in flowering control (Lee et al., 2000; Samach et al., 2000). Our previous study showed that SOC1 is regulated directly by CO-NF-Y via the recruitment of an H3K27 demethylase, RELATIVE OF EARLY FLOWERING6 (REF6), under LD conditions (Hou et al., 2014). Although H3K27me3 levels in SOC1 decrease during the flowering transition, we did not observe that CLF affects SOC1 gene expression in this study (Fig. 2C), suggesting that NF-YC mediates H3K27me3 deposition in SOC1 chromatin primarily by recruiting REF6 rather than by impairing CLF activity. In addition, we detected gene expression and H3K27me3 levels of FLC, SEP3, and AG genes, which are known as CLF targets (Goodrich et al., 1997; Schubert et al., 2006; Lopez-Vernaza et al., 2012). These results showed that these genes were hardly regulated by NF-YC, implying that other factors, but not NF-YC, might be involved in their regulation by CLF. Furthermore, since the dynamics of the H3K27me3 mark regulated by CLF at many genomic loci may be detected only in specific tissues and at specific developmental stages (Liu et al., 2016a), it is possible that the NF-YC-CLF module also could contribute to the precise control of other developmental events.
In addition to H3K27me3, other histone modifications, such as H3K4me3 and histone acetylation, also are involved in the regulation of flowering (He et al., 2003; Engelhorn et al., 2017). Generally, repressive H3K27me3 and activation-related H3K4me3 oppositely regulate the spatial and temporal expression of genes during specific developmental processes. A recent genome-wide study revealed that the H3K4me3 deposition prevails over H3K27me3, whereas H3K27me3 reduction occurs later during early flower morphogenesis (Engelhorn et al., 2017). Since NF-YC functions with CO to promote FT transcription, whereas CO activates FT by recruiting MRG1/2 proteins, the H3K4me3/H3K36me3 readers (Kumimoto et al., 2010; Bu et al., 2014), it could be speculated that H3K4me3 modification might be involved in NF-YC-mediated flowering control. It was reported that several histone deacetylases (HDAs) regulate flowering by the transcriptional repression of flowering repressor genes such as FLC, MAF1, MAF4, and MAF5 (Wang et al., 2014). Although our previous work revealed that NF-YC regulates photomorphogenesis by HDA15-mediated histone acetylation (Tang et al., 2017), no evidence supports the idea that NF-YC-HDA15 is involved in flowering regulation. However, it is worthwhile investigating whether NF-YC regulates flowering via recruiting other HDAs related to the flowering response, such as HDA6/9/19 (Wang et al., 2014).
It is intriguing that a temporal-specific interaction of NF-YC and CLF was observed by time-course Co-IP assays (Fig. 5B). The increasing interaction during the flowering transition under LD conditions results in attenuated CLF binding and H3K27me3 deposition in FT chromatin, which convincingly explains the photoperiod-induced FT up-regulation. In the photoperiod pathway, the periodic change of CO protein abundance by light is well known to underlie the rhythmic expression of FT within a day. However, this does not fully explain FT transcripts increasing day by day under LD conditions, which was attributed previously to FT accumulation or other positive feedback regulations (Suárez-López et al., 2001). Here, the temporal-specific NF-YC-CLF module provides a plausible hypothesis for increasing FT transcript abundance. In this scenario, FT may be induced by an NF-YC-triggered decrease of H3K27me3 when seedlings are ready for flowering. Because we did not observe a significant increase of NF-YC protein level during the flowering transition, it raises the question of how the appropriate timing of the NF-YC interaction with CLF is regulated. We propose that other mediating factors or posttranslational modifications, which are induced by the flowering signals, may be included in this process. Previous work reported a functional interaction between the cullin-RING ubiquitin ligase (CUL4-DDB1) and the PRC2 complex (Pazhouhandeh et al., 2011). CO is known to interact with NF-YC, and turnover of the CO proteins is triggered by COP1-SPA complexes, a central repressor of light signaling, in the dark (Jang et al., 2008; Chen et al., 2010). In addition, it was reported that the CUL4-DDB1 complex is required for FT expression and associates with the COP1-SPA complex (Pazhouhandeh et al., 2011). The interaction of NF-YC and CLF may be mediated by CUL4-DDB1, COP1-SPA, or CO in a light-dependent manner, which is worthwhile for future investigation.
Taken together, we reveal the temporal-specific regulatory module NF-YC-CLF, through which NF-YC directly counteracts CLF activity to promote FT expression and flowering at an appropriate time. These findings provide novel insight into the molecular mechanisms of how plants precisely control specific development events by the temporal regulation of epigenetic factors.
MATERIALS AND METHODS
Plant Material and Growth Conditions
The Arabidopsis (Arabidopsis thaliana) plant materials nf-yc3-2 nf-yc4-1 nf-yc9-1 (nf-ycT) and nf-yc9 NF-YC9-FLAG were described previously (Hou et al., 2014; Liu et al., 2016b). clf-28 (SALK_139371) seeds were obtained from the Arabidopsis Biological Resource Center. clf GFP-CLF plants were generated by crossing 35S:GFP-CLF (Schubert et al., 2006) with clf-28 in the Col background. The nf-ycT clf GFP-CLF and clf nf-yc9 GFP-CLF NF-YC9-FLAG plants were generated by crossing clf GFP-CLF with nf-ycT and nf-yc9 NF-YC9-FLAG plants. Plant growth conditions were described previously (Hou et al., 2014), under which the flowering transition time of the Col wild type has been defined to occur during 7 to 11 d after germination (Liu et al., 2008). The average number of rosette leaves calculated from a minimum of 10 plants at bolting was used as an indicator of flowering time.
Yeast Two-Hybrid Assay
The coding regions of NF-YC3, NF-YC4, NF-YC9, CLF, and truncated versions of NF-YC9 and CLF were amplified and cloned into pGBKT7 (binding domain) and pGADT7 (activation domain) vectors (Clontech), respectively. The primers used are listed in Supplemental Table S1. Yeast two-hybrid assays were performed using the Yeastmaker Yeast Transformation System 2 (Clontech). Yeast AH109 cells were cotransformed with the selected binding domain and activation domain constructs. The yeast transformants were grown and selected on SD/-Trp/-Leu or SD/-Trp/-Leu/-His/-Ade medium.
BiFC Analysis
The coding regions of NF-YC9 and CLF were cloned into the pGreen binary vectors containing C- or N-terminal fusions of EYFP to generate 35S:NF-YC9-nEYFP and 35S:cEYFP-CLF constructs. Plasmids were cotransformed into tobacco (Nicotiana tabacum) leaf epidermal cells with Agrobacterium tumefaciens strain GV3101-psoup as described previously (Tang et al., 2017). The YFP signals were detected by an inverted fluorescence microscope (Leica) in tobacco leaf grown for 48 h after infiltration, while 4′,6-diamidino-2-phenylindole staining was used as the nuclear localization indicator.
Pull-Down Assay
Using the designed primers (Supplemental Table S1), the coding regions of NF-YC3, NF-YC4, NF-YC9, and CLF were amplified and cloned into the pQE30 (Qiagen) and pGEX-4T-1 (Pharmacia) vectors to produce His-NF-YC3, His-NF-YC4, His-NF-YC9, and GST-CLF constructs, respectively. These GST and His recombinant constructs and the empty pGEX-4T-1 plasmid were transformed into Escherichia coli Rosetta cells, and then recombinant protein expression was induced by isopropyl-β-d-thiogalactoside (Sigma). The purified His-NF-YC3, His-NF-YC4, and His-NF-YC9 proteins were incubated with immobilized GST or GST-CLF using glutathione-Sepharose beads (Amersham Biosciences) and analyzed using immunoblots as described previously (Liu et al., 2016b).
Co-IP Assay
The clf nf-yc9 GFP-CLF NF-YC9-FLAG seedlings grown in LD conditions were harvested at the indicated growth stages and ground in liquid nitrogen. Nuclear proteins were extracted as described previously (Hou et al., 2014) and then incubated with Protein G PLUS/Protein A-Agarose Suspension (IP10; Calbiochem) plus anti-GFP antibody (ab290; Abcam) or preimmune serum (IgG) in Co-IP buffer (50 mm HEPES, pH 7.5, 150 mm KCl, 10 mm ZnSO4, 5 mm MgCl2, and 1% [v/v] Triton X-100) at 4°C overnight. After immunoprecipitation, the beads were washed and eluted with SDS loading buffer. The precipitated proteins were resolved by SDS-PAGE and immunodetected by anti-GFP and anti-FLAG (F3165; Sigma) antibodies.
Gene Expression Analysis
Various seedlings grown in LD conditions were harvested at the indicated growth stages for total RNA extraction using the E.Z.N.A. Total RNA Kit I (Omega) and reverse transcribed to cDNA using MMLV-RTase (Promega). qPCR was performed using a LightCycler 480 thermal cycler system (Roche) with KAPA SYBR Fast qPCR Kit Master Mix (Kapa Bio). The difference between the cycle threshold of target genes and the cycle threshold of the control gene was calculated by the relative quantification method (2−△△Ct) and used to evaluate quantitative variation. The primers used for gene expression analysis are listed in Supplemental Table S1. All expression analyses were performed with at least three biological replicates (three replicates of samples were taken from the same batch of seedlings, and total RNA was extracted from the pooled three to five seedlings per independent replicate). The above experiments were independently performed at least three times, and representative results are shown. The P value in data statistics was calculated by two-tailed Student’s t test and one-way ANOVA.
ChIP Assay
ChIP assays were performed primarily as described previously (Hou et al., 2014). The clf GFP-CLF and nf-ycT clf GFP-CLF seedlings grown in LD conditions were collected at the indicated growth stages and fixed for 40 min in 1% formaldehyde. Then, the nuclear proteins were extracted, and chromatin was isolated and sonicated to create DNA fragments approximately 500 bp in length on average. After that, the sonicated chromatin was immunoprecipitated by GFP-Trap (ChromoTek) or Protein G PLUS Agarose (16-201; Millipore) plus H3K27me3 antibody (07-449; Millipore) at 4°C overnight, and the remaining chromatin was used as an input control. The precipitated DNA was recovered and quantified by qPCR with KAPA SYBR Fast qPCR Kit Master Mix (Kapa Bio). Relative ChIP enrichment was calculated by normalizing the amount of a target DNA fragment against that of a Cinful-like genomic fragment and then against the respective input DNA amount. The primers used for the ChIP assay are listed in Supplemental Table S1. All ChIP analyses were performed in three biological replicates (three replicates of samples were taken from the same batch of seedlings, and chromatins were extracted from the pooled 20 to 30 seedlings per replicate). The above experiments were independently performed at least three times, and representative results are shown. The P value in data statistics was calculated by two-tailed Student’s t test and one-way ANOVA.
Accession Numbers
Accession numbers are as follows: NF-YC3 (AT1G54830), NF-YC4 (AT5G63470), NF-YC9 (AT1G08970), CLF (AT2G23380), CO (AT5G15840), FT (AT1G65480), FLC (AT5G10140), AG (AT4G18960), SEP3 (AT1G24260), TUB2 (AT5G62690), Cinful-like (AT4G03770), and ACT2 (AT5G09810).
Supplemental Data
The following supplemental materials are available.
Supplemental Figure S1. Expression analysis of NF-YC3, NF-YC4, NF-YC9, and CLF genes in nf-ycT, clf, and Col plants.
Supplemental Figure S2. Loss of NF-YB has a minimal effect on the early flowering of clf mutants.
Supplemental Figure S3. Quantitative analysis of FT gene circadian expression in nf-ycT, clf, clf nf-ycT, and Col plants.
Supplemental Figure S4. Expression analysis of FLC, AG, and SEP3 genes in nf-ycT, clf, clf nf-ycT, and Col plants.
Supplemental Figure S5. Time-course ChIP analysis of GFP-CLF binding and H3K27me3 levels at the ACT2 locus.
Supplemental Figure S6. ChIP analysis of CLF binding to the FT locus in the presence of the C-terminal-deleted NF-YC9.
Supplemental Table S1. List of primers used in this study.
ACKNOWLEDGMENTS
We thank the Arabidopsis Biological Resource Center for providing the mutant seeds used in this study.
LITERATURE CITED
Author notes
This work was supported by grants from the National Natural Science Foundation of China (no. 31301055), the Guangdong Science and Technology Department of China (no. 2015B020231009), and the Natural Science Foundation of Guangdong Province (no. 2017A030313211).
These authors contributed equally to the article.
Address correspondence to [email protected].
The author responsible for distribution of materials integral to the findings presented in this article in accordance with the policy described in the Instructions for Authors (www.plantphysiol.org) is: Xingliang Hou ([email protected]).
X.L. and X.H. designed the research; X.L., Y.Y., Y.H., L.Z., and Y.L. performed the research; X.L., Y.Y., and X.H. analyzed the data; X.L. and X.H. wrote the article.
Articles can be viewed without a subscription.