-
PDF
- Split View
-
Views
-
Cite
Cite
Eun Yu Kim, Ki Youl Park, Young Sam Seo, Woo Taek Kim, Arabidopsis Small Rubber Particle Protein Homolog SRPs Play Dual Roles as Positive Factors for Tissue Growth and Development and in Drought Stress Responses , Plant Physiology, Volume 170, Issue 4, April 2016, Pages 2494–2510, https://doi.org/10.1104/pp.16.00165
- Share Icon Share
Abstract
Lipid droplets (LDs) act as repositories for fatty acids and sterols, which are used for various cellular processes such as energy production and membrane and hormone synthesis. LD-associated proteins play important roles in seed development and germination, but their functions in postgermination growth are not well understood. Arabidopsis (Arabidopsis thaliana) contains three SRP homologs (SRP1, SRP2, and SRP3) that share sequence identities with small rubber particle proteins of the rubber tree (Hevea brasiliensis). In this report, the possible cellular roles of SRPs in postgermination growth and the drought tolerance response were investigated. Arabidopsis SRPs appeared to be LD-associated proteins and displayed polymerization properties in vivo and in vitro. SRP-overexpressing transgenic Arabidopsis plants (35S:SRP1, 35S:SRP2, and 35S:SRP3) exhibited higher vegetative and reproductive growth and markedly better tolerance to drought stress than wild-type Arabidopsis. In addition, constitutive over-expression of SRPs resulted in increased numbers of large LDs in postgermination seedlings. In contrast, single (srp1, 35S:SRP2-RNAi, and srp3) and triple (35S:SRP2-RNAi/srp1srp3) loss-of-function mutant lines exhibited the opposite phenotypes. Our results suggest that Arabidopsis SRPs play dual roles as positive factors in postgermination growth and the drought stress tolerance response. The possible relationships between LD-associated proteins and the drought stress response are discussed.
Environmental stresses, including drought, high salinity, oxidative stress, and unfavorable temperatures, profoundly affect the growth and development of higher plants. Because of their sessile life cycle, plants have developed self-protective mechanisms to increase their tolerance to short- and long-term stresses by triggering diverse sets of signal transduction pathways and activating stress-responsive genes. The genetic and cellular mechanisms in response to abiotic stress have been widely documented in higher plants (Shinozaki and Yamaguchi-Shinozaki, 1996; Bray, 1997; Ishitani et al., 1997; Zhu, 2002; Bohnert et al., 2006; Shinozaki and Yamaguchi-Shinozaki, 2007; Vij and Tyagi, 2007).
Lipid droplets (LDs) are dynamic subcellular organelles enclosed by a monolayer of phospholipid. LDs act as repositories for fatty acids and sterols, which are used for energy production and membrane and hormone synthesis. LDs are also involved in various cellular processes, including intracellular protein storage, stress responses, and lipid signaling (Bartz et al., 2007; Zehmer et al., 2009; Carman, 2012; Herker and Ott, 2012; Murphy, 2012; Sun et al., 2013; Kory et al., 2015). LDs bud from the endoplasmic reticulum (ER), where they become enriched with triacylglycerols and subsequently enlarged, until they pinch off to form an LD (Chapman et al., 2012; Chapman and Ohlrogge, 2012; Jacquier et al., 2013). Several reports suggest that LD-associated proteins, such as fat-specific protein 27 (FSP27), SEIPIN, and PERILIPIN1 (Plin1), are key regulators of LD formation in mammals, Drosophila, and yeasts (Farese and Walther, 2009; Xu et al., 2012; Yang et al., 2012). After budding from the ER, LDs fuse with each other and expand. In adipocytes, Plin1 functions as an enhancer of FSP27-mediated lipid transfer and LD growth, indicating that Plin1 and FSP27 participate in LD formation and fusion (Sun et al., 2013). Enlarged LDs provide surfaces to allow the attachment of numerous LD-associated proteins, which are later displaced during shrinkage of LDs by lipolysis (Kory et al., 2015).
Because LDs are mainly present in seeds, studies on LD-associated proteins in higher plants have focused on seed development and germination (Chapman et al., 2013; Gidda et al., 2013; Horn et al., 2013; Szymanski et al., 2014). For example, oleosins regulate LD size in Arabidopsis (Arabidopsis thaliana) seed development (Siloto et al., 2006). Arabidopsis SEIPINs modulate LD proliferation and neutral lipid accumulation in developing seeds (Cai et al., 2015). On the other hand, the cellular roles of LD-associated proteins in postgermination growth remain largely unraveled.
CaSRP1 (Capsicum annuum stress-related protein 1) was previously identified as a hot pepper small rubber particle protein (SRPPs) homolog (Hong and Kim, 2005). CaSRP1 was induced in response to water stress in hot pepper plants. Constitutive over-expression of CaSRP1 in transgenic Arabidopsis plants resulted in elevated growth and increased drought tolerance relative to wild-type Arabidopsis (Kim et al., 2010). CaSRP1 is evolutionarily related to SRPPs in rubber-producing plants (Wititsuwannakul et al., 2008). Rubber particles are single-membrane organelles that store rubber (cis-1,4-polyisoprene). Although rubber particles and LDs have different lipid compositions, their basic architectures are similar (Cornish et al., 1999). Thus, SRPP homologs may have common properties in the formation and biogenesis of rubber particles and/or LDs in rubber-producing and non-rubber-producing plants.
In this report, we identified and characterized three SRPP homologs, SRP1, SRP2, and SRP3, in Arabidopsis. The SRP genes were differentially expressed in various tissues and induced by abscisic acid (ABA) and a broad spectrum of abiotic stress, including drought, high salinity, and low temperature. SRP-overexpressing transgenic Arabidopsis plants (35S:SRP1, 35S:SRP2, and 35S:SRP3) exhibited higher vegetative and reproductive growth and markedly better tolerance to drought stress than wild-type Arabidopsis plants. In addition, ectopic expression of SRPs resulted in increased numbers of large LDs in postgermination seedlings. In contrast, single (srp1, 35S:SRP2-RNAi, and srp3) and triple (35S:SRP2-RNAi/srp1srp3) loss-of-function mutant lines showed the opposite phenotypes. Arabidopsis SRPs appeared to be LD-associated proteins and displayed polymerization properties in vivo and in vitro. These results are discussed in light of the suggestion that Arabidopsis SRPs play dual roles as positive factors in postgermination growth and drought stress response. The possible relationships between LD-associated proteins and stress tolerance response are also discussed.
RESULTS
Arabidopsis Contains Three SRP Homologs
In our prior study, CaSRP1 was identified as a hot pepper small rubber particle protein homolog. CaSRP1 was induced by dehydration in hot pepper plants, and CaSRP1-over-expressing transgenic Arabidopsis lines showed increased growth and enhanced drought tolerance relative to wild-type plants (Kim et al., 2010). In this study, we extended these findings to identify Arabidopsis SRP homologs. The Arabidopsis genome contains three SRP homologs: SRP1 (At1g67360), SRP2 (At2g47780), and SRP3 (At3g05500). Figure 1A represents the restriction map of the three SRP cDNAs. The predicted SRP1, SRP2, and SRP3 proteins are composed of 240 amino acids (Mr = 26.4 kD and pI value = 8.9), 235 amino acids (Mr = 26.6 kD and pI value = 4.4), and 246 amino acids (Mr = 27.2 kD and pI value = 8.3), respectively (Fig. 1A; Supplemental Fig. S1A).
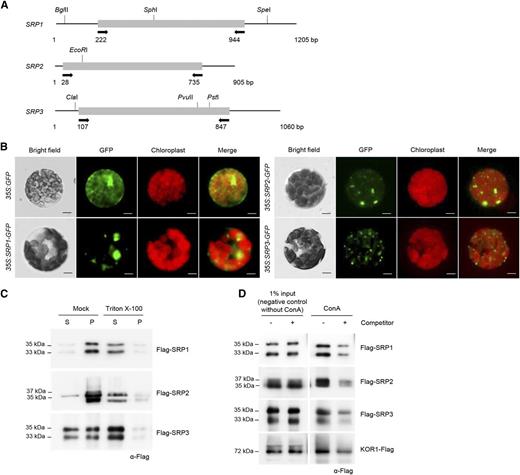
Characterization of Arabidopsis SRPs. A, Schematic structures of SRPs. Solid bars represent the coding regions. Solid lines depict the 5′-and 3′-untranslated regions. Arrows indicate the primers used for genotyping PCR and RT-PCR. Nucleotide sequences of the primers are listed in Supplemental Table S1. B, Subcellular localizations of SRPs in tobacco protoplasts. Protoplasts were obtained from tobacco leaves that transiently expressed 35S:SRP-GFP fusion constructs. The GFP signals were detected by fluorescence microscopy under dark-field or light-field conditions. Scale bars = 10 μm. C, Membrane-association assay of SRPs. The 35S:Flag-SRP fusion genes were transiently expressed in tobacco leaves. Soluble (S) and pellet (P) fractions of total leaf protein extracts were treated with Triton X-100 and subjected to immuno-blot analysis using anti-Flag antibody. D, Lectin-binding assay of SRPs. Total protein extracts were prepared from Flag-SRP-expressing tobacco leaves and were incubated with Con A-sepharose resin in the presence or absence of methyl α-d-glucopyranoside. Proteins eluted from the sepharose resin were analyzed by protein gel-blotting using anti-Flag antibody. Methyl α-d-glucopyranoside was used as a competitor to bind to Con A. KOR1 was used as a positive glycoprotein marker.
The 35S:SRP-GFP constructs were transiently expressed in tobacco (Nicotiana benthamiana) leaf cells by Agrobacterium-mediated transfection, after which protoplasts were prepared. Figure 1B shows that the fluorescent signals of all three SRP-GFP fusion proteins were mostly confined to the cytosolic fraction with spot- or dot-like structures. Subsequently, the 35S:Flag-SRP fusion genes were transiently expressed in tobacco leaves, and the expressed proteins were characterized by immuno-blot analysis. The Flag-SRP proteins exhibited slightly different electrophoretic mobilities (33-35 kD and 35-37 kD), as detected by the anti-Flag antibody in tobacco total leaf extracts (Fig. 1C). These two shifted bands probably represented the truncated or modified forms of Flag-SRP fusion proteins due to unknown reasons. Flag-SRP1 and Flag-SRP2 proteins were exclusively found in the pellet fraction of the leaf extracts, while Flag-SRP3 was equally distributed in both the pellet and soluble fractions (Fig. 1C). When the Flag-SRP protein extracts were treated with 1% Triton X-100, most SRPs were detected in the soluble fractions, suggesting that SRPs are membrane associated.
SRPs share amino acid identities (34%-54%) with rubber tree (Hevea brasiliensis) SRPP (Supplemental Fig. S1, A and B). Rubber tree SRPP is a glycoprotein (Wititsuwannakul et al., 2008). To test whether Arabidopsis SRPs are glycosylated, a lectin-binding assay was performed using concanavalin A (Con A) column. As shown in Figure 1D, transiently expressed Flag-SRPs and endo-1,4-β-glucanase korrigan 1 (KOR1), a positive glycoprotein marker, bound to the Con A column. These lectin-binding activities were inhibited by methyl α-d-glucopyranoside, which has an affinity for Con A. Together, these results indicate that Arabidopsis SRPs contain lectin binding activities, suggesting that they are glycoproteins.
Arabidopsis SRPs Are Upregulated by ABA and Various Abiotic Stress Treatments
To analyze the spatial expression patterns of SRPs in various tissues of Arabidopsis, reverse transcription (RT)-PCR (Supplemental Fig. S2) and real-time quantitative (qRT)-PCR (Fig. 2A) were performed. The results showed that SRP3 is a major transcript in all tissues examined, including seedlings, roots, leaves, stems, flowers, siliques, and developing seeds (Fig. 2A). SRP1 and SRP2 exhibited low levels of expression in Arabidopsis plants. One exception was that a high amount of SRP2 transcript was detected in developing seeds (Fig. 2A). The histochemical promoter-GUS assay also revealed that SRP3 is highly expressed throughout the Arabidopsis plants (Fig. 2B). Low levels of SRP1 promoter activity were detected in the hydathodes, main veins of cotyledons, and newly dividing tissues, such as immature leaves, lateral root primordia, and root tips, whereas GUS activity of the SRP2 promoter was barely detectable in the immature leaves of 7-d-old seedlings (Fig. 2B).

Expression of SRPs. A, Spatial expression patterns of SRPs in Arabidopsis. Transcript levels of SRP1, SRP2, and SRP3 were analyzed in seedlings, roots, leaves, stems, flowers, siliques, and seeds by real-time qRT-PCR. The expression fold of SRPs was normalized to that of glyceraldehyde-3-phosphate dehydrogenase C subunit, which was used as a reference gene. Results are presented as means ± sd from three independent biological replicates. B, Promoter activities of SRP1, SRP2, and SRP3 in developing Arabidopsis tissues. Histochemical GUS expression profiles in T3 pSRP-GUS transgenic plants were visualized by cyclohexylammonium salt staining for 16 h. Arrow indicates low level of pSRP2-GUS activity in the immature leaves of 7-d-old seedlings. DAF, day after flowering. Scale bars = 0.25 cm. C, qRT-PCR analysis of SRPs. Light-grown, 7-d-old, wild-type Arabidopsis seedlings were subjected to drought (0, 1.5, and 3 h), low temperature (4°C for 0, 1.5, and 3 h), high salinity (300 mm NaCl for 0 and 4.5 h), and 100 μm ABA (0, 1.5, and 3 h). Total RNA was extracted from the treated tissues and analyzed by qRT-PCR. Glyceraldehyde-3-phosphate dehydrogenase C subunit mRNA was used as an internal control for normalization. Error bars indicate means ± sd from three independent experiments.
Further transcription analysis of SRPs was carried out using 7-d-old whole seedlings in response to ABA and various abiotic stress treatments. All three SRP transcripts were induced by 100 μm ABA treatment (0, 1.5, and 3 h; Fig. 2C). SRP1 and SRP3 were induced by different abiotic stresses, including drought (0, 1.5, and 3 h), low temperature (4°C for 0, 1.5, and 3 h), and high salinity (300 mm NaCl for 0 and 4.5 h), while expression of SRP2 was enhanced selectively by dehydration (Fig. 2C).
Analysis (http://arabidopsis.med.ohio-state.edu) of upstream regions of three SRP genes indicated that they share similar cis-acting elements in their promoters, including Dc3 Promoter-Binding Factor-1 (DPBF1) and -2 (DPBF2; Kim et al., 1997), MYB (Sablowski et al., 1994), related to ABI3/VP1-A (Kagaya et al., 1999), LEAFY consensus (Lamb et al., 2002), and ABA-responsive elements (ABRE)-like (Choi et al., 2000) binding sites (Supplemental Table S2). In addition, SRP2 has additional regulatory elements in its promoter region, such as MYC2 (Abe et al., 1997), G-box binding factor 1/2/3 (de Vetten and Ferl, 1995), ABRE binding factors (Choi et al., 2000), auxin response factors (Ulmasov et al., 1999), and C-repeat/dehydration responsive element binding factor 2 (Pla et al., 1993) binding motifs. These putative upstream cis-acting sequences in SRPs are in agreement with qRT-PCR and histochemical GUS data, suggesting that the expression of Arabidopsis SRPs is subject to control by developmental processes and abiotic stresses.
SRPs Play Positive Roles in Tissue Growth and Development
To investigate the cellular roles of SRPs, transgenic Arabidopsis plants (35S:SRP1, 35S:SRP2, and 35S:SRP3) that over-expressed full-length SRPs under the control of the 35S CaMV promoter were constructed (Supplemental Fig. S3A). In addition, T-DNA-inserted knock-out mutants of SRP1 (srp1) and SRP3 (srp3) were obtained (Supplemental Fig. S3, B and C). Because a loss-of-function mutant of SRP2 was unavailable, RNAi-mediated knock-down transgenic lines of SRP2 (35S:SRP2-RNAi) were generated (Supplemental Fig. S3D). The triple mutant line (35S:SRP2-RNAi/srp1srp3) was subsequently constructed by introducing the 35S:SRP2-RNAi construct into the srp1srp3 double knock-out mutant (Supplemental Figure S3E).
The phenotypic properties of the gain-of-function and loss-of-function progeny of SRPs were first investigated under normal growth conditions. As indicated in Figure 3A, the germination rates of the T4 over-expressing and mutant lines were indistinguishable at 1 to 5 d after imbibition, suggesting that SRPs may not be involved in the germination process under normal growth conditions. However, the postgermination growth rates of over-expressing and mutant lines were clearly different. We found that 4-d-old SRP-overexpressing seedlings displayed increased (1.4- to 1.7-fold) root growth; in contrast, the single (srp1 and srp3) and triple (35S:SRP2-RNAi/srp1srp3) mutants exhibited shorter (0.5- to 0.7-fold) roots than the wild-type plants (Fig. 3B, left). The root growths of these plants were similar at 1 to 2 d after germination but became increasingly different at 3 to 8 d after germination (Fig. 3B, right). We noticed that the phenotypic difference of 35S:SRP2-RNAi was not as clear as those of the srp1 and srp3 mutant lines. This was probably due to incomplete silencing of SRP2 (Supplemental Fig. S3D). Alternatively, the expression level of SRP2 is very low in most vegetative tissues (Fig. 2, A and B), and suppression of SRP2, in contrast to its over-expression, may not affect Arabidopsis growth under normal growth conditions.
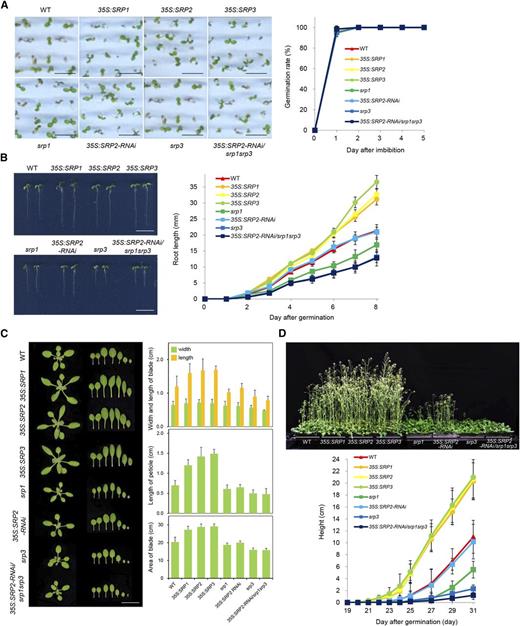
Phenotypic characterization of SRP-overexpressing and loss-of-function srp mutant plants. A, Germination assay. Left: wild-type (WT), SRP-over-expressing, and srp mutant seeds were germinated on half-strength Murashige and Skoog medium. Scale bars = 0.5 cm. Right: germination rates with respect to radicle emergency were determined 1 to 5 d after imbibition. Error bars indicate means ± sd (n = 80). B, Root growth. Left: morphological comparisons of wild-type, SRP-over-expressing, and srp mutant seedlings 4 d after germination. Scale bars = 2.0 cm. Right: growth profiles of wild-type, SRP-over-expressing, and srp mutant early roots 1 to 8 d after germination. Error bars indicate means ± sd (n = 40). C, Leaf growth. Left: morphological comparisons of 2-week-old rosettes from wild-type, SRP-over-expressing, and srp mutant plants. Scale bars = 2.0 cm. Right: quantification of the dimensional parameters of the second leaf. Detached second leaves were analyzed using the SCIONIMAGE program. Error bars indicate means ± sd (n = 40). D, Bolting. Top: bolting phenotypes of wild-type, SRP-over-expressing, and srp mutant plants. Bottom: growth rates of the inflorescence stems of wild-type, SRP-overexpressing, and srp mutant plants were monitored 19 to 31 d after germination. Error bars indicate means ± sd (n = 80).
Figure 3C shows that 2-week-old 35S:SRP transgenic leaves were longer (1.3- to 1.4-fold) than the wild-type leaves, resulting in an approximately 1.5-fold increase in leaf surface area. The petiole length was also increased by 1.7 to 2.1 times in the SRP-over-expressing lines. In contrast, the loss-of-function srp1, srp3, and 35S:SRP2-RNAi/srp1srp3 mutant plants displayed, in general, the opposite phenotypes; they developed shorter leaves and petioles and consequently, their leaf area was reduced compared with wild-type plants (Fig. 3C). Again, the 35S:SRP2-RNAi knock-down plants showed wild-type-like phenotypes. Overall, growth retardation was most severe in srp3 and 35S:SRP2-RNAi/srp1srp3 (Fig. 3, B and C), suggesting that SRP3 is critical for leaf and root development.
The most striking phenotype of the SRP plants was that the SRP over-expressors grew more rapidly and bolted approximately 2 to 3 d earlier than the wild-type Arabidopsis plants did (Fig. 3D, top). Under our growth conditions, the majority of the wild-type plants bolted at 23 d after germination. However, the 35S:SRP transgenic lines began bolting at 20 to 21 d after germination. At 25 d after germination, the inflorescence length of SRP-over-expressing plants was approximately 5 times longer than that of the control plants (Fig. 3D, bottom). By contrast, the single (srp1 and srp3) and triple (35S:SRP2-RNAi/srp1srp3) mutant plants bolted 24 d after germination, indicating that suppression of SRPs resulted in delayed bolting phenotypes (Fig. 3D). Thus, these phenotypic analyses suggested that SRPs play positive roles in vegetative and reproductive growth in Arabidopsis.
The Tissue-Growth Phenotypes of the Over-expression and Loss-of-Function Mutants of SRPs Were Correlated with Cell Proliferation
To test whether the changes in root and leaf growth in the over-expression and loss-of-function mutants of SRPs were caused by alteration in cell division or elongation, the root tips and leaf cell layers were examined microscopically. The SRP1-, SRP2-, and SRP3-over-expressing plants exhibited an expanded proximal meristem (PM) zone in their root tips (Fig. 4A). Meristem cell number in the root tip PM zones of wild-type and over-expressing plants was 29.0 ± 0.5 and 35.0 ± 1.3, respectively, indicating that the three SRPs had similar positive impacts on root tip growth. In contrast, the srp1, srp3, and 35S:SRP2-RNAi/srp1srp3 mutant plants displayed a reduced number of meristem cells (22.0 ± 1.0, 19.0 ± 0.7, and 18.0 ± 0.3, respectively) in their root tip PM zones. 35S:SRP2-RNAi knock-down root tips showed wild-type-like phenotypes. In addition, the 35S:SRP transgenic leaves possessed smaller and more populated cells in both the epidermal and mesophyll layers relative to wild-type leaves (Fig. 4B). Figure 4B also shows that the srp1, srp3, and 35S:SRP2-RNAi/srp1srp3 mutant plants possessed lager and less dense cells in their leaves. These results indicate that the SRP over-expressors contained an increased number of cells in both the root and leaf tissues, while loss-of-function of SRP1 and SRP3 resulted in decreased cell number. Thus, it is likely that the enhanced growth that resulted from SRP over-expression was correlated with cell proliferation rather than cell elongation.
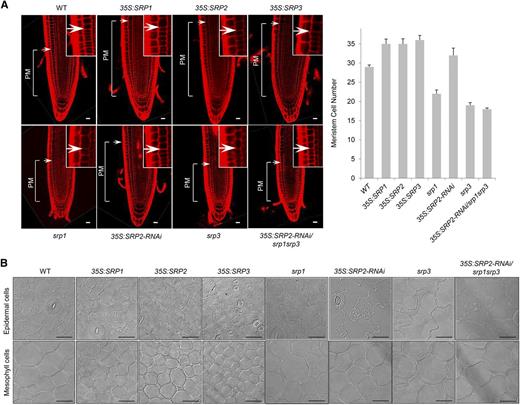
Cellular phenotypes of 35S:SRP transgenic and srp mutant plants. A, Left: longitudinal sections of 3-d-old roots from wild-type, SRP-over-expressing, and srp mutant plants. Vertical lines indicate the PM. Arrows represent the transition zone between the PM and elongation-differentiation zones. The insets show a magnified transition zone. Root sections were stained with propidium iodide and visualized by confocal microscopy. Right: measurement of meristem cell number. Error bars indicate means ± sd (n = 30). B, Different cell sizes in wild-type, SRP-over-expressing, and srp mutant leaves. The epidermal and mesophyll cell layers of 5-d-old leaves were treated with chloral hydrate, a tissue-clearing reagent. The image was visualized by light microscopy. Scale bars = 20 μm.
SRPs Participate in the Positive Regulation of Drought Stress Responses
On the basis of the stress-induced expression profiles of SRPs (Fig. 2C), we anticipated that SRPs participate in stress tolerance responses in Arabidopsis. In particular, all three SRPs are upregulated in response to dehydration (Fig. 2C). Therefore, the survival ratios of SRP-over-expressing and mutant plants were monitored after drought treatment. Light-grown, 2-week-old wild-type and SRP over-expressors were subjected to dehydration by withholding water for 10 d, which resulted in complete drying of the potted soil. These water-stressed plants were irrigated, and their survival rates were analyzed 3 d after reirrigation. The results indicated that the SRP over-expressors were more tolerant to water stress than the wild-type plants. The survival ratios of 35S:SRP1, 35S:SRP2, and 35S:SRP3 were 65% (59 of 91), 66% (59 of 89), and 58% (47 of 81), respectively, which are 2.2- to 2.5-fold higher than that (27%, 24 of 89) of the wild-type plants (Fig. 5A, left). In contrast, the loss-of-function progeny were highly sensitive to mild dehydration treatment. Three-week-old wild-type and mutant plants were exposed to water stress for 8 d, and the recovery rates were measured after 3 d of rewatering. After this mild drought treatment, 100% (96 of 96) of wild-type plants resumed growth, while the survival rates of the srp1, 35S:SRP2-RNAi, srp3, and 35S:SRP2-RNAi/srp1srp3 plants were 40% (35 of 88), 7% (6 of 92), 24% (23 of 94), and 0% (0 of 91), respectively (Fig. 5A, right). Triple 35S:SRP2-RNAi/srp1srp3 mutant plants were extremely sensitive to dehydration, suggesting that the three SRP homologs play interconnected roles to cope with conditions of water deficit. It should be noted that the 35S:SRP2-RNAi knock-down plants were markedly more susceptible to water stress than the srp1 and srp3 lines, suggesting a critical role for SRP2 in drought stress response. This view is consistent with the result that SRP2 is specifically induced by dehydration stress (Fig. 2C).

Drought sensitivity of wild-type, SRP-over-expressing, and srp mutant plants. A, Left: Light-grown, 2-week-old wild-type (WT) and SRP over-expressors (35S:SRP1, 35S:SRP2, and 35S:SRP3) were further grown for 10 d without irrigation. Plants were rewatered, and the survival rates were determined 3 d after rewatering. Right: wild-type and srp mutant (srp1, 35S:SRP2-RNAi, srp3, and 35S:SRP2-RNAi/srp1srp3) plants were grown for 3 weeks under normal growth conditions. After 8 d of desiccation, the plants were recovered by rewatering, and the number of surviving plants was counted 3 d after irrigation. B, Total chlorophyll amounts (mg/g DW) in wild-type, SRP-over-expressing, and srp mutant leaves after drought stress. Error bars indicate means ± sd (n = 20). C, Pro content of wild-type, SRP-over-expressing, and srp mutant leaves before (gray bars) and after (dark bars) water stress. Error bars indicate means ± sd (n = 15). D, Measurement of the rate of water loss in cut rosettes from wild-type, SRP-over-expressing, and srp mutant plants. Detached rosette leaves were subjected to desiccation for 0 to 5 h at room temperature. Water loss is represented as the percentage of the initial fresh weight of the detached rosette leaves. Error bars indicate means ± sd (n = 8).
In agreement with the survival rates, SRP-over-expressing leaves contained higher amounts of chlorophyll a + chlorophyll b (18.9 ± 0.5 mg/g dry weight [DW] to 19.3 ± 1.1 mg/g DW) than the wild-type leaves (12.9 ± 0.5 mg/g DW) after drought stress (Fig. 5B). As expected, the chlorophyll levels were decreased in the mutant leaves, with that of the triple 35S:SRP2-RNAi/srp1srp3 mutant leaves being most severely reduced (3.3 ± 0.5 mg/g DW). In addition, the Pro content of the 35S:SRP leaves was up to 3.3 times higher than that of the wild-type leaves before and after drought treatment, while the Pro content of the mutant leaves was lower (Fig. 5C). These results indicate that the expression levels of SRPs are positively correlated with photosynthetic activity and the accumulation of osmoprotectants.
Finally, the cut rosette water loss assay indicated that SRP-over-expressing plants retained their leaf water more efficiently than the wild-type plants. When 3-week-old detached rosette leaves from wild-type and over-expressing plants were incubated at room temperature for 5 h under dim light, the wild-type leaves lost approximately 72% of their fresh weight relative to the starting weight, whereas the SRP-over-expressing leaves lost only 49% to 52% of their fresh weight (Fig. 5D). The loss-of-function mutant leaves displayed the opposite phenotypes. After 5 h of incubation, detached mutant leaves lost up to 71% to 80% of their fresh weights, indicating that more rapid water loss occurred in the mutant leaves than in the wild-type leaves (Fig. 5D). Collectively, the phenotypic analyses presented in Figure 5 strongly support the notion that the three SRP homologs play combinatory roles in the positive regulation of dehydration stress responses in Arabidopsis.
SRPs Are Related with Cell Wall Organization
The aforementioned results indicate that Arabidopsis SRPs are involved in tissue growth and development (Figs. 3 and 4) and in the tolerance to drought stress (Fig. 5), which indicates dual roles for SRPs in growth and stress responses. Therefore, more detailed phenotypic properties of SRP over-expressors and mutants were investigated. Because SRP-over-expressing tissues displayed an elevated cell proliferation rate and more rapidly growing phenotypes, and vice versa for mutant tissues (Figs. 3 and 4), we considered the possibility that the cell wall structures of these plants are affected by the expression levels of SRPs. Seed coat is often used to analyze cell wall structure (Stork et al., 2010). Thus, the seed coat structures of over-expressors and mutants of SRPs were examined by SEM. In general, the columella, which is the epidermal layer of the Arabidopsis seed coat, exhibited a hexagonal and volcano-shaped structure (Fig. 6A). Regular but enlarged columellae were observed in SRP-overexpressing seeds, resulting in less dense cell wall structures relative to the wild-type seeds (Supplemental Fig. S4). The columella of the 35S:SRP2-RNAi knock-down plants showed wild-type-like structures. However, the typical hexagonal and volcano structures of the columellae were attenuated in the other loss-of-function mutants (srp1, srp3, and 35S:SRP2-RNAi/srp1srp3). Furthermore, their epidermal cells displayed aberrant and irregular shapes, although their sizes were somewhat similar to those of wild-type seeds (Fig. 6A; Supplemental Fig. S4). Wall-associated kinase 1 (WAK1), FEI2, and pectin methylesterase (PME3) are cell wall marker genes (Micheli, 2001; Xu et al., 2008; Steinwand and Kieber, 2010). WAK1 and FEI2 are receptor-like kinases that are required for cell wall expansion and synthesis of cell wall components, respectively. PME3 catalyzes the demethylesterification of pectin, which contributes to cell wall growth (Micheli, 2001). As shown in Figure 6B, all three cell wall marker genes were upregulated in the SRP over-expressors (1.4- to 14.8-fold), and in contrast, they were downregulated in the triple mutant plant (0.7-fold), relative to the expression levels in the wild-type plant (Fig. 6B). Overall, these results support the view that SRPs are involved in cell wall organization in Arabidopsis.
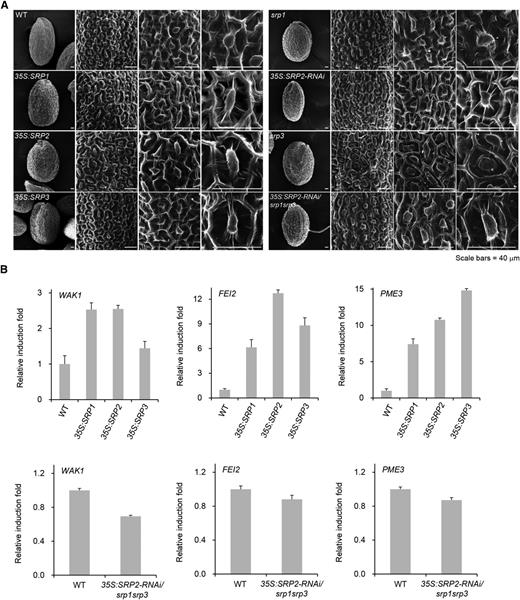
Surface structure of seed coats and the expression levels of cell wall-related genes. A, SEM analysis of dry seeds of wild-type (WT), 35S:SRP transgenic, and srp mutant plants. Scale bar = 40 μm. B, Transcriptional changes in cell wall-modifying genes (WAK1, FEI1, and PME3) in SRP-over-expressing and mutant progeny relative to wild-type plants. Data represent means ± sd from three independent experiments.
SRPs Are Colocalized to LDs in Tobacco Leaves
Horn et al. (2013) reported that LDAP1 and LDAP2 are LD-associated proteins in avocado (Persea Americana). Avocado LDAP1 and LDAP2 are homologs of SRP3. Like LDAP1 and LDAP2, SRP3 is localized at the surface of LDs in tobacco cells (Horn et al., 2013). The subcellular localization patterns of SRP1 and SRP2 were very similar to that of SRP3, suggesting that they are localized to LDs (Fig. 1B). To confirm these results, colocalization of SRPs and LDs were examined. As shown in Figure 7A, mature tobacco leaves contained few LDs detected by Nile red staining. LEAFY COTYLEDON 2 (LEC2) is a transcription regulator that increases number of LDs by promoting the expression of seed-specific genes and lipid accumulation (Cai et al., 2015). Consistent with previous results, 35S:LEC2-infiltrated tobacco leaves exhibited increased number of LDs (Fig. 7B). When 35S:LEC2 and 35S:SRPs-GFP constructs were coinfiltrated, the Nile red-stained LDs and SRPs-GFP fluorescent signals were well merged in tobacco leaves (Fig. 7C). Thus, it seems highly likely that SRPs and LDs are closely associated, suggesting that SRPs are localized to LDs.

Colocalization of Arabidopsis SRPs to LDs. The 35S:P19 (A), 35S:P19 + 35S:LEC2 (B), and 35S:P19 + 35S:LEC2 + 35S:SRPs-GFP (C) constructs were transiently expressed in tobacco leaves via Agrobacterium-mediated infiltration. P19, a viral suppressor of posttranscriptional gene-silencing, was used to increase infiltration efficiency. LEC2 was used to promote lipid accumulation and increase number of LDs. After 3 d of infiltration, leaves were stained using Nile red (2 μg/mL) and fluorescent signals were visualized via confocal microscopy. Red and green signals represented LDs and SRP-GFPs, respectively. Scale bars = 20 μm.
SRPs Play Positive Roles in the Biogenesis of LDs
Subcellular colocalization studies suggest that SRP1, SRP2, and SRP3 are localized to LDs (Fig. 7). In both plant and mammalian systems, the development of LDs is closely linked to the optimal functioning of LD-associated proteins (Guo et al., 2009; Wilfling et al., 2014). Therefore, we hypothesized that Arabidopsis SRPs are also involved in LD development. LDs are abundant in the dry-seed stage, and their numbers gradually reduce during germination and seedling establishment in Arabidopsis (Eastmond, 2006; Kim et al., 2011). We analyzed the size and number of cellular LDs in the 6-d-old seedlings of the SRP over-expressors and 35S:SRP2-RNAi/srp1srp3 triple mutant plants by transmission electron microscopy (TEM). Various sizes of LDs were observed in the wild-type seedlings: approximately 9.8% of LDs were >2.5 μm in diameter, 19.7% were 2.0 to 2.5 μm, 16.4% were 1.5 to 2.0 μm, 32.8% were 1.0 to 1.5 μm, 16.4% were 0.5 to 1.0 μm, and 5.2% were 0.0 to 0.5 μm (Fig. 8A). However, in the SRP-over-expressing seedlings, numerous enlarged LDs were routinely detected. For example, 13.1% to 17.2% of the LDs were larger than 2.5 μm in diameter, and 22.1% to 34.7% were 2.0 to 2.5 μm (Fig. 8A). In contrast, only 0.1% to 1.3% of the LDs were 0.0 to 0.5 μm in diameter, which suggests that SRP over-expression resulted in an increased number of large LDs in the early seedlings. The opposite phenotypes were observed in the 35S:SRP2-RNAi/srp1srp3 mutant seedlings. As indicated in Figure 8A, the number of small-sized LDs (0.0-0.5 μm in diameter) was increased by up to approximately 15.0% in these plants. In addition, LDs with diameters >2.5 μm were rarely detected in the triple mutant seedlings. Thus, it is likely that SRPs are positively involved in the biogenesis of LDs. Alternatively, SRPs may inhibit the lipolysis of LDs by blocking LD-lipase interaction, thereby influencing LD morphology. Fatty acids, which are formed by the lipolysis of LD, are transported into the mitochondria and used to generate ATP via β-oxidation (Aon et al., 2014). qRT-PCR analysis revealed that the mRNA levels of both cytochrome c oxidase 1 (COX1) and COX2, which encode the rate-limiting enzymes of the mitochondrial respiratory chain (Khalimonchuk and Rödel, 2005; Fontanesi et al., 2008), were correlated with SRP mRNA levels. COX1 and COX2 were up-regulated and down-regulated in the SRP over-expressors and 35S:SRP2-RNAi/srp1srp3 mutant seedlings, respectively (Fig. 8B). These data indicate that the biogenesis, rather than the lipolysis, of LDs was affected by the expression of SRPs. Collectively, these results suggest that SRPs play positive roles in determining the number and size of LDs.
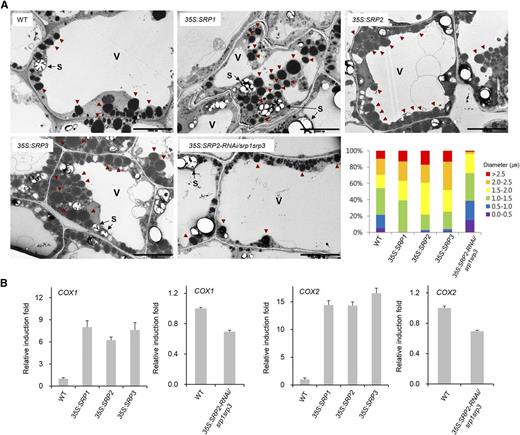
Distribution of LDs and the expression levels of mitochondrial respiratory genes. A, TEM analysis of 6-d-old hypocotyls from wild-type (WT), SRP over-expressors, and 35S:SRP2-RNAi/srp1srp3 triple mutant plants. LDs were divided into six groups (>2.5, 2.0-2.5, 1.5-2.0, 1.0-1.5, 0.5-1.0, and 0.0-0.5 μm) depending on their sizes. Red arrowheads indicate LDs. V, central vacuole; S, starch granule. Scale bars = 10 μm. B, Expression of COX1 and COX2, mitochondrial respiratory marker genes, in wild-type, 35S:SRP transgenic, and 35S:SRP2-RNAi/srp1srp3 mutant plants. Data represent means ± sd from three independent experiments.
SRPs Exhibit Polymerization Properties in Vivo and in Vitro
Rubber tree SRPP exhibits latex coagglutination activity (Wititsuwannakul et al., 2008). In addition, bacterially expressed recombinant SRPP displayed the self-assembly property (Berthelot et al., 2014a). Thus, we hypothesized that Arabidopsis SRPs play a role in the formation of LDs via their polymerization properties. To test this hypothesis, the 35S: Flag-SRP constructs were infiltrated into tobacco leaves. Total protein crude extracts were prepared and analyzed by immuno-gel blot assay. When nondenatured crude extract samples were subjected to native protein gel-blot analysis, high-molecular-weight smear bands of Flag-SRP proteins were detected by anti-Flag antibody (Figure 9A, top). This result was different from that of SDS denaturing PAGE gel that displayed two shifted protein bands (Fig. 1C; Fig. 9A, bottom). Similar results were obtained with bacterially expressed 6×His-2×Flag-SRP recombinant proteins. As shown in Figure 9B, purified 6×His-2×Flag-SRPs exhibited high-molecular-weight ladders in a native PAGE gel (Fig. 9B, lane 1, top). In contrast, these smear bands disappeared when the protein samples were denatured (Fig. 9B, lane 1, bottom). The 6×His-2×Flag-SRPs were denatured by heat in the presence of 0.2% SDS and 100 mm β-mercaptoethanol. The denatured 6×His-2×Flag-SRPs were then renatured by removal of the detergents through dialysis at 28°C for 2 h and subjected to immuno-blot analysis on a native or denaturing PAGE gel. The results showed that high-molecular-mass smear bands were apparent in a native PAGE gel (Fig. 9B, lane 2, top) and, in contrast, dimeric bands were detected in denaturing PAGE gel (Fig. 9B, lane 2, bottom). These results suggest that SRPs are polymerized in vivo and in vitro.
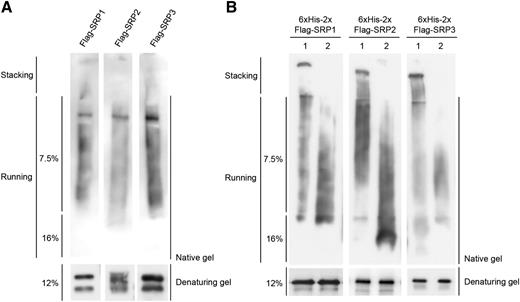
Polymerization properties of SRPs. A, In vivo polymerization of SRPs. Total protein extracts were prepared from 35S:Flag-SRPs-infiltrated tobacco leaves and subjected to immuno-blot analysis by native (7.5% and 16%; top) or denaturing (12%; bottom) PAGE gel with anti-Flag antibody. B, Lane 1: bacterially expressed 6×His-2×Flag-fused SRPs were purified and subjected to immuno-blot analysis by native (7.5% and 16%; top) or denaturing (12%; bottom) PAGE gel with anti-Flag antibody. Lane 2: 6×His-2×Flag-fused SRPs were boiled in the presence of 0.2% SDS and 100 mm β-mercaptoethanol. Denatured 6×His-2×Flag-SRPs were renatured for 2 h at 28°C and subjected to immuno-blotting by native (top) or denaturing (bottom) PAGE gel with anti-Flag antibody.
DISCUSSION
In this study, we characterized three SRP homologs in Arabidopsis. Similar to rubber tree SRPP, Arabidopsis SRPs appear to be membrane-associated glycoproteins (Fig. 1, C and D). SRP1, SRP2, and SRP3 are differentially expressed in various tissues and are induced by a broad spectrum of abiotic stimuli and ABA (Fig. 2). Consistent with their expression profiles, SRPs are positively involved in the growth of both vegetative and reproductive tissues (Figs. 3 and 4), as well as tolerance response to drought stress (Fig. 5). These results suggest that Arabidopsis SRPs play dual roles as positive factors in tissue growth and development and in drought stress response.
Rubber tree SRPP is localized to the surface of rubber particles, which have structural similarities to LDs in non-rubber-producing plants (Schmidt et al., 2010; Berthelot et al., 2014b). In addition, avocado SRP homologs (LDAP1 and LDAP2) and Arabidopsis SRP3 localize to the surface of LDs (Horn et al., 2013). Our current data show that the subcellular localization patterns of SRP1 and SRP2 are very similar to that of SRP3 and are reminiscent of those of LDAP1 and LDAP2 (Fig. 1B). Confocal microscopy with Nile red-stained tobacco leaves strongly indicates that three SRPs are colocalized to LDs (Fig. 7). Thus, SRP1 and SRP2, in addition to SRP3, are likely associated with LDs. SRPP in rubber tree participates in rubber particle aggregation and latex coagulation (Wititsuwannakul et al., 2008). Arabidopsis SRPs are related with the number and size of LDs, as evidenced by the results that over-expression of SRPs resulted in the accumulation of large-sized LDs in seedlings (Fig. 8) and that SRPs displayed in vivo and in vitro polymerization properties (Fig. 9). Thus, it seems that the cellular roles of SRP homologs are conserved, at least in part, in rubber-producing and non-rubber-producing plants.
Because LDs store neutral lipids, their biogenesis is closely related to cell proliferation in both mammalian and plant systems (Siloto et al., 2006; Yonezawa et al., 2011). Regulation of adipocytes, which are specialized tissues for lipid storage, is an important concern in human diseases such as diabetes and obesity (Gong et al., 2011). For example, fat-specific protein 27 (Fsp27) is a mammalian LD-associated protein that regulates LD biogenesis and affects cellular respiratory rate in the ob mouse (Yonezawa et al., 2011). In higher plants, LDs participate in cell proliferation in the seedling establishment stage by providing neutral lipids as carbon sources, while the cellular roles of LDs in postgermination growth are not well understood (Siloto et al., 2006). Studies on plant LD-associated proteins, including oleosins, caleosins, oil body-associated protein (OBAPs), and SEIPIN, have mainly focused on seed development and germination (López-Ribera et al., 2014; Cai et al., 2015). On the other hand, expression of Arabidopsis SRPs has been detected in postgermination tissues, including roots, leaves, and flowers (Fig. 2, A and B), and SRPs participate in postgermination growth and early bolting (Figs. 3 and 4). Furthermore, the germination profiles of SRP-over-expressing and mutant lines were undistinguishable from that of the wild-type (Fig. 3A). Thus, SRPs play a role in postgermination Arabidopsis growth. This view is further supported by the results that cell wall marker genes (WAK1, FEI2, and PME3) and mitochondrial respiratory genes (COX1 and COX2) were up-regulated in postgermination seedlings of SRP-over-expressing plants (Figs. 6B and 8B). The predicted amino acid sequence alignment revealed that the monocot model crop rice, which produces glutelin-rich seeds, contains two SRP homologs (Supplemental Fig. S1, A and B). Overall, we speculate that the LD-associated SRP homologs are involved in the broad aspects of tissue growth and development in non-rubber-producing plants.
Responsive to Dehydration 20 is a stress-inducible caleosin that is involved in the drought stress response (Aubert et al., 2010). Caleosin families were thus named because they possess calcium-binding sites comprised of a helix-loop-helix EF-hand motif (Laibach et al., 2015). Because calcium is a critical factor in the stress-signaling pathway, LD-associated caleosins participate in plant stress responses in addition to their structural role in LDs. These results may provide clues to the functions of LDs in stress responses (Purkrtova et al., 2008). Our data (Fig. 5) and previous results obtained by other groups show that LD-associated SRP homologs function as positive factors in abiotic stress responses (Kim et al., 2010; Seo et al., 2010; Balbuena et al., 2011; Fricke et al., 2013; Gidda et al., 2013). LDs serve as a reservoir for isoprenoids and steroid compounds, as well as neutral lipids, which act as hormones and/or antioxidant molecules. Thus, one cannot discount the possibility that LDs mediate stress responses by regulating these lipid compounds. This is consistent with the notion that SRPs confer enhanced drought tolerance by promoting the biogenesis of LDs (Fig. 8A).
An additional property of LDs as a protein-storage depot is that they store extra-nuclear histones and may provide histones to the nuclei for efficient chromatin assembly during times of high demand (Cermelli et al., 2006; Li et al., 2012). Spatiotemporal regulation of histones by LDs may control the expression of a subset of genes during cellular proliferation and in response to stress, which in turn, increases tissue growth and tolerance against stress such as drought. It should be noted that, because different kinds of proteins are associated with LDs, molecular crowding is a key factor in the determination of the composition of LD-associated proteins (Kory et al., 2015). Thus, it is plausible that various LD-associated proteins such as SRPs are involved in different but interconnected cellular aspects, including tissues growth and stress responses. Further experiments are required to identify additional LD-associated proteins and uncover their roles in the regulation LD biogenesis and drought stress tolerance in Arabidopsis.
It is noteworthy that, among the three SRP homologs, the expression level of SRP2 was very low in most tissues examined and was selectively induced by drought (Fig. 2). The 35S:SRP2-RNAi knock-down plants exhibited wild-type-like phenotypes in terms of tissue growth (Figs. 3 and 4), but they were extremely sensitive to dehydration stress. In addition, a high level of SRP2 transcript was exceptionally detected in developing seeds (Fig. 2A). SRP2 contains an acidic pI value of 4.4, whereas those of SRP1 and SRP3 are close to neutral (8.9 and 8.3, respectively). More diverse putative cis-acting regulatory elements exist in the upstream region of SRP2 compared to those of SRP1 and SRP3 (Supplemental Table S2). Thus, although SRP1, SRP2, and SRP3 play combinatory roles in diverse cellular aspects, there might be a functional specificity among the three SRPs in Arabidopsis.
Another interesting result is that Arabidopsis SRPs have polymerization properties (Fig. 9). Polymerization patterns of SRPs were indistinguishable in vivo and in vitro and, thus, it seems likely that glycosylation may not be essential for polymerization of these Arabidopsis proteins. Similarly, rubber tree SRPP exhibits latex coagglutination activity and self-assembly activity in vitro (Wititsuwannakul et al., 2008; Berthelot et al., 2014b). Other LD-associated proteins, including FSP27 and SEIPIN, act as oligomers to regulate size of LD (Chapman et al., 2012; Cai et al., 2015). These indicate that homo- or hetero-oligomerization of LD-associated proteins is involved in the biogenesis of LDs.
It should be noted that SRPs were not detected in Arabidopsis LD proteome data from mature leaves and seeds (Jolivet et al., 2004; Shimada et al., 2014). One possibility is that expression of SRPs are tightly regulated transcriptionally (Fig. 2) and/or posttranslationally in response to developmental process and environmental stress. SRPs are routinely solubilized from the membrane fraction in the presence of detergent (Fig. 1C). Thus, it is also possible that SRPs are associated with LDs transiently and/or reversibly under certain conditions (e.g. in response to drought stress and in mature leaves). Formation of LDs was induced by unfavorable growth conditions, such as nitrogen starvation, excess lipids, and ER stress, in animals, yeasts, and algae (Hapala et al., 2011; Siaut et al., 2011; Krahmer et al., 2013). Recently, Cai et al. (2015) reported that LD-associated protein SEIPIN promotes LD biogenesis in Arabidopsis. These results are consistent with our data that transgenic Arabidopsis seedlings, in which stress-induced SRPs are constitutively expressed, display markedly increased number of LDs as compared to wild-type seedlings (Fig. 8A). Thus, it would be intriguing to examine whether LDs may play as an intermediary host to shuttle SRPs to other organelles and/or cytosolic fractions in response to abiotic stress and developmental processes.
In conclusion, our data suggest that LD-associated SRPs play dual roles as positive factors in tissue growth and development and in drought stress responses in Arabidopsis. These results are consistent with the view that biogenesis and proper functioning of LDs are critical not only to seed development and germination but also to broad physiological aspects in non-rubber-producing Arabidopsis.
MATERIALS AND METHODS
Plant Materials and Stress Treatments
Arabidopsis (Arabidopsis thaliana) ecotype Columbia wild-type and T-DNA-inserted loss-of-function srp1 (GABI_309G05) and srp3 (Salk_103065) mutant seeds were obtained from the Ohio State University Arabidopsis Biological Resources Center (Columbus, OH). Homozygous srp1 and srp3 mutant lines were isolated by genotyping PCR using T-DNA left border and gene-specific primers (Supplemental Table S1). Full-length SRP1 (Arabidopsis Biological Resources Center stock no. U11034), SRP2 (U24879), and SRP3 (U137621) cDNAs were cloned into the binary vector pBI121. SRP-over-expressing transgenic lines were generated as described previously (Seo et al., 2008). The RNAi-mediated knock-down construct of SRP2 (35S:SRP2-RNAi) was introduced into the wild-type and srp1srp3 double mutant plants. The expression levels of SRPs in transgenic and mutant progeny were investigated by RT-PCR using gene-specific primer sets (Supplemental Table S1). Wild-type, mutant (srp1, 35S:SRP2-RNAi, srp3, and 35S:SRP2-RNAi/srp1srp3), and SRP-over-expressing plants were treated with ABA (100 μm for 0, 1.5, and 3 h), drought (0, 1.5, and 3 h), low temperature (4°C for 0, 1.5, and 3 h), or high salinity (300 mm NaCl for 0 and 4.5 h) as described by Ryu et al. (2010).
Protoplast Transient Assay
Full-length SRP cDNAs were fused with a soluble-modified GFP (smGFP), and the 35S:SRP-GFP constructs were transiently expressed in tobacco (Nicotiana benthamiana) leaves by the infiltration method (Kim and Kim, 2013). After 3 d of infiltration, protoplasts were prepared as described by Seo et al. (2008). Expression and subcellular localization of SRP-GFP were monitored with a cooled CCD camera and a BX51 fluorescence microscope (Olympus, Tokyo, Japan) as described previously (Son et al., 2009).
Protein Assays
The 35S:Flag-SRP fusion genes were constructed using gene-specific primers (Supplemental Table S1) and were transiently coexpressed with 35S:P19 in tobacco leaves via Agrobacterium-mediated infiltration. P19, a viral suppressor of posttranscriptional gene-silencing, was used to increase infiltration efficiency (Hellens et al., 2005). For the membrane-association assay, total proteins were extracted from tobacco leaves using a protein-extraction buffer (150 mm NaCl, 1 mm EDTA, and 50 mm Tris-HCl buffer, pH 7.4) with or without 1% Triton X-100 (Phan et al., 2008). After 15 min of incubation, samples were centrifuged at 16,000 g for 10 min at 4°C. The supernatant and pellet fractions were analyzed by immuno-blotting with anti-Flag antibody.
For the lectin-binding assay, total proteins were prepared with the protein-extraction buffer containing 1% Triton X-100 and incubated with Con A-sepharose resin at 4°C overnight in the presence or absence of methyl α-d-glucopyranoside. Flag-fused KOR1-Flag was used as a positive marker for glycoproteins. After incubation, the sepharose resin was washed with the protein-extraction buffer, and bound proteins were eluted by boiling in SDS sample buffer. The eluted proteins were subjected to protein gel-blot analysis using the anti-Flag antibody (Park et al., 2010).
RNA Extraction, cDNA Synthesis, and qRT-PCR
Total RNA was isolated from developing tissues, including seedlings, roots, leaves, stems, flowers, siliques, and seeds, and from abiotic stress- or ABA-treated 7-d-old seedlings using the RNAiso RNA purification kit according to the manufacturer’s protocol (Takara, Shiga, Japan) with slight modifications as described by Xu et al. (2014). Total RNA was treated with DNase I (Promega, Madison, WI) for 30 min, and then, first-strand cDNA was synthesized as described by Kim et al. (2010). RT-PCR was performed using gene-specific primer sets (Supplemental Table S1). qRT-PCR was performed using an IQ5 light cycler (Bio-Rad, Hercules, CA), and the results were analyzed using the IQ5 optical system software (Bio-Rad), as described by Kim and Kim (2013).
Histochemical GUS Assay
The promoter regions of three SRPs were analyzed using the AGRIS program (Arabidopsis Gene Regulatory Information Server, http://arabidopsis.med.ohio-state.edu/AticsDB; promoter ID: SRP1, At1g67360; SRP2, At2g47780; and SRP3, At3g05500). The promoter-SRP-GUS constructs (pSRP1-GUS, pSRP2-GUS, and pSRP3-GUS) were introduced into Arabidopsis as described previously (Cho et al., 2011). For GUS staining, T3 pSRP-GUS transgenic plants were immersed in 50 mm sodium phosphate buffer (pH 7.2) containing 2 mm cyclohexylammonium salt (Duchefa Biochemie, Haarlem, Netherlands), 0.5 mm K3Fe(CN)6, and 0.5 mm K4Fe(CN)6, and incubated overnight at 37°C as described previously (Yoon et al., 2014). Chlorophyll was removed from the GUS-stained tissue by treatment with 70% ethanol for 6 h.
Phenotypic Analyses
For the germination assay, wild-type, SRP-overexpressing transgenic, and srp loss-of-function mutant seeds were collected at the same time and grown on half-strength Murashige and Skoog medium supplemented with vitamins and 1% Suc at 22°C with continuous light. The percentages of radicle emergence were calculated 1 to 5 d after sowing. Growth of root and leaf tissues was monitored with the image-analyzing program SCIONIMAGE (Scion Corp., Frederic, MD) as described by Seo et al. (2008). For the microscopic analysis, 3-d-old roots were stained with propidium iodide and visualized using confocal microscopy (LSM510 META; Carl Zeiss, Oberkochen, Germany) as described by Seo et al. (2008). For leaf cell layer observation, 5-d-old leaves were cleared with chloral-hydrate solution, and the images were analyzed using light-field microscopy (BX51 fluorescence microscope, Olympus).
Drought Stress Analysis
Light-grown, 2- to 3-week-old wild-type, 35S:SRP transgenic, and srp mutant plants were subjected to drought stress by withholding water for 8 to 10 d. The plants were then rewatered, and their survival rates were determined after 3 d of rewatering (Kim et al., 2010). Total chlorophyll (chlorophyll a + chlorophyll b) was extracted from the drought-treated leaves as described previously by Bae et al. (2009). The amount of Pro in normal and drought-treated leaves was determined as described by Claussen (2005).
SEM and TEM
The surface structures of wild-type, SRP-over-expressing, and srp-mutant seeds were examined by high-resolution SEM (model S-800, FESEM, Hitachi, Tokyo, Japan) at an accelerating voltage of 3 kV under high vacuum conditions (Penfield et al., 2001; Atia et al., 2009). For TEM, hypocotyl tissues of 6-d-old seedlings were fixed in 2.5% (v/v) glutaraldehyde, 4% (v/v) formaldehyde, and 100 mm phosphate buffer, pH 7.2, as described by Kim et al. (2011). Thin sections of tissues were prepared using an ultra-microtome (Leica, Vienna, Austria).
Colocalization assays of Arabidopsis SRPs to LDs
The 35S:P19, 35S:P19 + 35S:LEC2, and 35S:P19 + 35S:LEC2 + 35S:SRPs-GFP constructs were transiently expressed in tobacco leaves. P19 was used to increase infiltration efficiency (Hellens et al., 2005). LEC2 is a transcription regulator and was used to promote lipid accumulation and increase number of LDs (Cai et al., 2015). After 3 d of infiltration, tobacco leaves were stained with 2 μg/mL Nile red (Sigma-Aldrich, St. Louis, MO) in 50 mm PIPES buffer (pH 7.0). LDs were imaged using a LSM700 confocal laser scanning microscope (Carl Zeiss). To detect fluorescent signals of GFP and Nile red, samples were excited by 488-nm laser and the emission was acquired from 500 to 540 nm and 560 to 620 nm, respectively.
SRP Polymerization Assay
The 2×Flag-tagged SRP constructs were ligated into the pProEX vector (Invitrogen, Carlsbad, CA) and introduced into BL21 (DE3) E. coli strain. Expression of 6×His-2×Flag-SRPs was induced by the addition of IPTG (0.3 mM) into the growth medium and expressed recombinant proteins were purified using the Ni-NTA system according to the manufacturer’s protocol (QIAGEN, Hilden, Germany). The 35S:Flag-SRP constructs were infiltrated into tobacco leaves. The bacterially purified 6×His-2×Flag-SRPs and total crude protein extracts of Flag-SRPs-expressing leaves were boiled for 10 min in TBS containing 0.2% SDS and 100 mm β-mercaptoethanol for protein denaturation. The denatured SRPs were then renatured by removal of detergent through dialysis at 28°C for 2 h and subjected to native (7.5% and 16%) or denaturing (12%) PAGE followed by immuno-blot analysis using anti-Flag antibody.
Accession Numbers
Sequence data from this article can be found in the GenBank/EMBL data libraries under accession numbers SRP1 (NP_176904), SRP2 (NP_182299), and SRP3 (NP_187201).
Supplemental Data
The following supplemental materials are available.
Supplemental Figure S1. Sequence analysis of Arabidopsis SRPs.
Supplemental Figure S2. Expression profiles of SRPs.
Supplemental Figure S3. Generation and isolation of SRP-overexpressing and T-DNA-inserted srp mutant plants.
Supplemental Figure S4. Number of columella cells in seed coats of wild-type, SRPs-over-expressing, and mutant plants.
Supplemental Table S1. The sequence of primers used in this study.
Supplemental Table S2. Sequence analysis of upstream regions of three SRP genes.
Glossary
- ABA
abscisic acid
- Con A
concanavalin A
- DW
dry weight
- ER
endoplasmic reticulum
- LD
lipid droplet
- PM
proximal meristem
- qRT-PCR
real-time quantitative
- RT
reverse transcription
- SEM
scanning electron microscopy
- TEM
transmission electron microscopy
LITERATURE CITED
Author notes
This work was supported by grants from the Woo Jang Chun Special Project (PJ009106) funded by the Rural Development Administration, Republic of Korea, to W.T.K.
Present address: The Korean Ginseng Research Institute, Korea Ginseng Corp., Daejeon 305-805, Korea.
Address correspondence to [email protected]
The author responsible for distribution of materials integral to the findings presented in this article in accordance with the policy described in the Instructions for Authors (www.plantphysiol.org) is: Woo Taek Kim ([email protected]).
E.Y.K., K.Y.P., and Y.S.S. performed the experiments; E.Y.K., K.Y.P., and W.T.K. analyzed the data; E.Y.K., K.Y.P., and W.T.K. planned the project and wrote the article; W.T.K. supervised the project and complemented the writing.
Articles can be viewed without a subscription.