-
PDF
- Split View
-
Views
-
Cite
Cite
Anjil Kumar Srivastava, Cunzin Zhang, Gary Yates, Mark Bailey, Adrian Brown, Ari Sadanandom, SUMO Is a Critical Regulator of Salt Stress Responses in Rice , Plant Physiology, Volume 170, Issue 4, April 2016, Pages 2378–2391, https://doi.org/10.1104/pp.15.01530
- Share Icon Share
Abstract
SUMO (Small Ubiquitin-like Modifier) conjugation onto target proteins has emerged as a very influential class of protein modification systems. SUMO1/2 double mutant plants are nonviable, underlining the importance of SUMO conjugation to plant survival. Once covalently bound, SUMO can alter a conjugated protein’s stability and/or function. SUMO conjugation is a highly dynamic process that can be rapidly reversed by the action of SUMO proteases. The balance between the conjugated/deconjugated forms is a major determinant in the modulation of SUMO-target function. Despite the important mechanistic role of SUMO proteases in model plants, until now the identity or the function of these regulatory enzymes has not been defined in any crop plant. In this report, we reveal the ubiquitin-like protease class of SUMO protease gene family in rice (Oryza sativa) and demonstrate a critical role for OsOTS1 SUMO protease in salt stress. OsOTS-RNAi rice plants accumulate high levels of SUMO-conjugated proteins during salt stress and are highly salt sensitive; however, in non-salt conditions, they are developmentally indistinguishable from wild-type plants. Transgenic rice plants overexpressing OsOTS1 have increased salt tolerance and a concomitant reduction in the levels of SUMOylated proteins. We demonstrate that OsOTS1 confers salt tolerance in rice by increasing root biomass. High salinity triggers OsOTS1 degradation, indicating that increased SUMO conjugation in rice plants during salt stress is in part achieved by down-regulation of OTS1/2 activity. OsOTS1 is nuclear localized indicating a direct requirement of OsOTS1-dependent deSUMOylation activity in rice nuclei for salt tolerance.
Rice (Oryza spp.) is the principal food crop for more than half of the world’s population and also serves as an excellent model system to understand the impact of environmental stress on crop yield. Rice is also a salt-sensitive monocot; in particular, seedlings and the reproductive stages are very sensitive to salinity, while germination is relatively tolerant (Lutts et al., 1996; Zheng and Shannon, 2000; Zheng et al., 2001). Understanding the mechanisms by which plants perceive salt stress signals and convey them to the cellular machinery to activate adaptive responses is crucial for classical breeding and biotechnology programs aimed at improving tolerance. Recent studies on model plants and crops indicate that these adaptive responses are underpinned by complex perception and signaling mechanisms that operate to produce an integrated physiological response to environmental stresses (Hasegawa et al., 2000; Zhu, 2002). Therefore identifying molecular targets that act as coordinators that influence multiple stress signaling pathways and revealing how these improve whole-plant stress physiology will be crucial for increasing crop productivity.
Protein modifications act at the core of plant stress responses by either activating (positive factors) or deactivating (negative factors) stress sensors and downstream transcription factors that control the expression of hundreds of genes. Protein ubiquitination and phosphorylation are the two most understood protein modifications controlling stress signaling. In recent years, SUMO (Small Ubiquitin-like Modifier) conjugation onto target proteins has emerged as a very influential protein modification system (Elrouby and Coupland, 2010; Miller et al., 2010). Different SUMO isoforms are present in Arabidopsis (Arabidopsis thaliana), although only two nearly identical isoforms AtSUMO1 and AtSUMO2, have been shown to be conjugated onto target proteins upon various types of stress (Catala et al., 2007; Conti et al., 2008). It has been demonstrated in a number of studies that mutants that fail to promote SUMO1/2 attachment onto target proteins display characteristic phenotypes including reduced growth, altered response to phosphate starvation, deregulated salicylic acid-dependant signaling upon pathogen infection, reduced basal thermotolerance, and impaired drought, freezing, and salinity tolerance (Hotson and Mudgett, 2004; Mudgett, 2005; Yoo et al., 2006; Catala et al., 2007; Miura et al., 2007a). These data illustrate the importance of SUMOylation in diverse response pathways. Indeed, SUMO1/2 double mutant plants are nonviable underlining the importance of SUMO conjugation to plant survival.
Like ubiquitination, SUMO conjugation in plants is facilitated by the sequential activity of three enzymes (E1, E2, and E3). In Arabidopsis, the E1 SUMO-activating enzymes AtSAE1 and AtSAE2 act as a heterodimer, responsible for adenylation-mediated ATP-dependent thiol-ester bond formation between SAE2 and SUMO. Transesterification results in the transfer of SUMO to the E2 SUMO-conjugating enzyme AtSCE1. AtSCE1 finally catalyzes SUMO isopeptide bond formation to target proteins, sometimes in conjunction with E3 SUMO ligases HIGH PLOIDY2 (AtHPY2/AtMMS21) or SAP and MIZ1 (AtSIZ1; Miura et al., 2005, 2007b; Saracco et al., 2007; Huang et al., 2009; Ishida et al., 2012; Novatchkova et al., 2012). Once covalently bound, SUMO can alter a conjugated protein stability and/or functionality. SUMO may facilitate new protein-protein interactions through SUMO-interacting motifs and compete with other posttranslational modifications, such as ubiquitination and acetylation (Müller et al., 2001; Kerscher, 2007; Elrouby and Coupland, 2010).
SUMO conjugation is a highly dynamic process that can be rapidly reversed by the action of SUMO proteases. There is strong evidence that SUMO proteases contribute to the regulation of flowering time, plant pathogen interactions, and adaptation to abiotic stress factors in Arabidopsis (Murtas et al., 2003; Xu et al., 2007; Conti et al., 2008; Kim et al., 2008). Thus, the balance between the conjugated/deconjugated forms is a major determinant in the modulation of SUMO-target function (Kurepa et al., 2003; Golebiowski et al., 2009). Despite the important mechanistic role for SUMO proteases, until now the identity or the function of these regulatory enzymes has not been defined in any crop plant. Previously we have shown that Arabidopsis mutant seedlings lacking the SUMO proteases OTS1 and OTS2 exhibit inhibition of root growth when exposed to 100 mm salt stress (Conti et al., 2008). We demonstrated that one of the ways OTS1 SUMO proteases regulate growth is to influence the abundance of growth restraining DELLA proteins via deSUMOylation. It is likely that the OTS class of ubiquitin-like protease (ULP) SUMO proteases may provide routes for boosting crop growth under stress.
In this article, we reveal the ULP-like SUMO protease gene family in rice and demonstrate a critical role for OsOTS1 SUMO protease in salt stress signaling. OsOTS-RNAi rice plants accumulate high levels of SUMO1-conjugated proteins during salt stress and are highly salt sensitive; however, in non-salt conditions, they are developmentally indistinguishable from wild-type plants. Transgenic rice plants overexpressing OsOTS1 have increased salt tolerance and a concomitant reduction in the levels of SUMOylated proteins. We demonstrate that OsOTS1 confers salt tolerance in rice by increasing root biomass. Intriguingly, high salinity triggers OsOTS1 degradation, indicating that increased SUMO conjugation in wild-type rice plants during salt stress is in part achieved by down-regulation of OTS1/2 activity. OsOTS1 is nuclear localized, indicating a direct requirement of OsOTS1-dependant deSUMOylation activity in rice nuclei for salt tolerance. Our data indicate that OsOTS SUMO proteases are likely to target proteins that act to promote root growth under salt stress.
RESULTS
Identification of SUMO Proteases in Rice
Recent reports showed that Arabidopsis SUMO proteases form a gene family comprising of at least eight members (Kurepa et al., 2003; Johnson, 2004). A characteristic feature of this gene family is the C terminus core protease domain (Li and Hochstrasser, 2003). Using this domain sequence, we performed a homology-based search against the rice genome to identify 12 genes encoding proteins with similar catalytic domains. A protein sequence alignment using Web-PRANK software (Fig. 1A) indicates that the similarity between these SUMO proteases is highest in the C-terminal protease domain. All of these putative SUMO proteases contain three highly conserved amino acids (Supplemental Fig. S1, highlighted in gray) previously reported to form the catalytic triad that includes His, Asp, and the critical Cys (Fig. 1A), the putative active site for these SUMO proteases. The phylogeny shown in Figure 1B shows two main groups for SUMO proteases. It appears that there is one group that is ULP and ULP-diverged, and the other includes ESD4. These two main groups of SUMO proteases can be divided again into two, making four recognizable groups within the 12 rice proteins. These four groups could represent the four classes identified in the dicot Arabidopsis as discussed by Novatchkova et al. (2012), which include, an ULP-like class, an OTS-like class, and an EDS-like class. Our data here suggest that the OTS and OTS-like proteins have diverged from the ULP/ULP-like group.
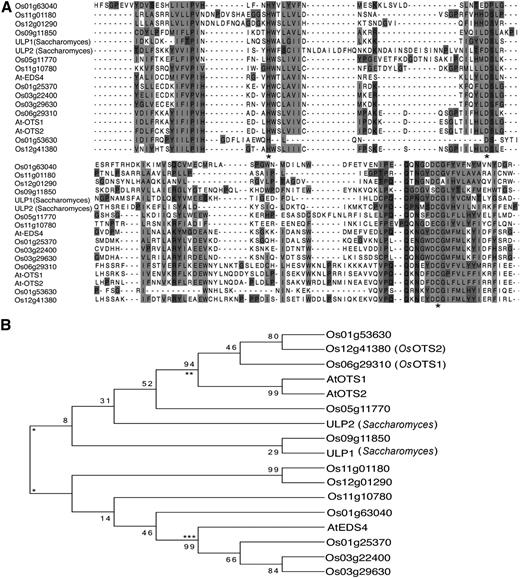
Alignment and phylogeny of rice SUMO protease proteins. Potential SUMO protease proteins were identified by ascertaining the presence of a SUMO protease catalytic domain (H-X-D-X-C). A, Alignment of 12 proteins from rice that contain this catalytic triad along with three Arabidopsis proteins, AtOTS1 (AT1G60220), AtOTS2 (AT1G10570), and AtESD4 (AT4G15880), and two proteins from Saccharomyces cerevisiae, ULP1 (YPL020C) and ULP2 (YIL031W). The catalytic triad is indicated by an asterisk. B, Phylogenetic tree analysis of the aligned proteins. There are two main branches into which all the proteases fall, marked by a single asterisk. The upper section is ULP and ULP-like, which can then be subdivided into two groups: (1) OTS/OTS-like (marked by **) and (2) ULP-like. Interestingly, the ULP1 from S. cerevisiae (used as an outgroup) has strongest homology to Os09g11850, which could imply that this protein may be one of the ancestral forms of SUMO protease. The lower section is again divided into two sections, the first (marked ***) is EDS-like, leaving four proteins that make up the final group. Taken together, it appears there are four groups represented by the 12 rice SUMO proteases. The percentage of replicate trees in which the associated taxa clustered together in the bootstrap test (1,000 replicates) is shown next to the branches.
Previously, we demonstrated that in Arabidopsis, OTS1 SUMO protease is important for salt tolerance. Construction of phylogenetic tree (Fig. 1B) based on the highly variable N terminus of AtOTS1 indicated that the closest homolog of AtOTS1 in O. sativa cv japonica is the protein encoded by the gene at locus LOC_Os06g29310; we designated this gene OsOTS1 (Fig. 1B). We also designated the second closest homolog as OsOTS2 (LOC_Os12g41380; Fig. 1B) based on sequence similarity to AtOTS2. We decided to exploit this knowledge to reveal the role of SUMO proteases in regulating stress tolerance in rice.
OsOTS1 Encodes a Bona Fide SUMO Protease That Is Expressed in Most Tissues in Rice
To test for OsOTS1 SUMO protease activity, we produced a recombinant His-tagged OsOTS1 (HIS:OsOTS1) in Escherichia coli. Affinity-purified HIS:OsOTS1 protein was incubated with proSUMO substrate consisting of recombinant His-tagged AtSUMO1 fused at their C terminus to the transcription factor FLC (HIS:AtSUMO1:FLC; Conti et al., 2008). After overnight incubation of recombinant protein in SUMO assay buffer, the products of the reaction were resolved by SDS-PAGE and analyzed by immunoblotting with anti-His antibodies. Incubation of proSUMO substrates (∼45 kD) with HIS:OsOTS1 or the positive control AtOTS1 resulted in the appearance of a single novel band representing His-AtSUMO1, the cleavage products of HIS:AtSUMO1:FLC, which migrated at the expected M r of ∼17 kD (Fig. 2A). These data clearly show that OTS1 has bona fide SUMO protease activity as its able to generate mature AtSUMO1 from its artificial precursor HIS:AtSUMO1:FLC.
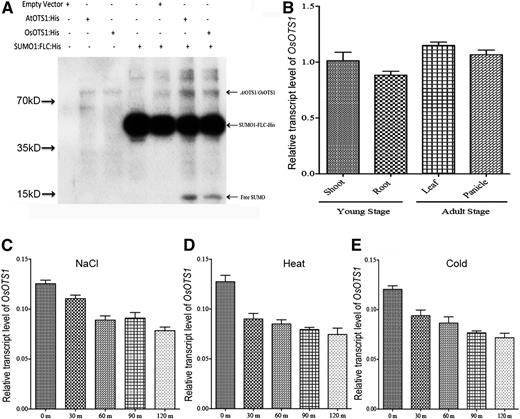
OsOTS1 is a bona fide SUMO protease expressed in different tissues. A, Immunoblot analysis of OsOTS1 in vitro protease activity. Each protein (AtOTS1, OsOTS1, and SUMO1:FLC::His) was expressed in E. coli and affinity purified. Proteins were incubated in different combination for 16 h, and the resulting mixture was resolved by 12% SDS-PAGE and probed with anti-His antibody. B, Expression profile of the OsOTS1 gene in various tissues. Total RNA was extracted from various tissues at different stages of rice plants under growth conditions. C to E, Expression analysis of OsOTS1 gene under 150 mm NaCl, heat (42°C), and cold (4°C) treatments. Total RNA was isolated from leaves of three independently treated plants, and real-time qPCR analysis of OsOTS1 was performed. OsOTS1 gene expression was normalized to that of rice actin, and the relative expression level was compared with that of control untreated plants. Mean values were obtained from three independent PCR amplifications. Error bars indicate sd.
To determine the gene expression pattern of OsOTS1 in rice, we performed quantitative real-time PCR (qRT-PCR), which indicated that OsOTS1 is expressed in all tissues tested with higher expression in the shoot in 10-d-old seedlings (Fig. 2B). However, gene expression was also observed at later stages of development in the adult leaves and in the panicles, indicating a role for SUMO deconjugation throughout rice plant development (Fig. 2B). Interestingly, we observed that OsOTS1 expression was suppressed in rice plants exposed to high salt (150 mm NaCl), heat (42°C), and cold stress (4°C) but did not show any significant difference after polyethylene glycol (20%) or abscisic acid (100 μm) treatment (Fig. 2, C–E; Supplemental Fig. S2). These data suggest that although OsOTS1 is expressed in multiple organs, the levels are responsive to different stresses. Significantly, the differential gene expression response of OsOTS1 to stresses is in contrast to AtOTS1, where we observed no alteration of gene expression under stress (Conti et al., 2008).
OsOTS1 Is Critical for Salt Tolerance in Rice
To ascertain the function of OsOTS SUMO proteases in rice, we generated independent transgenic lines, which efficiently cosilenced OsOTS1 and 2 expression by RNAi methods. In Arabidopsis, overexpressing OTS1 alone was sufficient for salt tolerance (Conti et al., 2008), and we wanted to ascertain if this was true in a evolutionarily divergent crop plant such as rice. Therefore, we also generated HA-tagged OsOTS1 overexpressing lines under the control of a maize (Zea mays) ubiquitin promoter in Nipponbare. qRT-PCR analysis confirmed that at least two independent single-copy OsOTS-RNAi or OsOTS1-overexpressing lines were produced (Supplemental Fig. S3). Two independent homozygous OsOTS-RNAi (1-2; 5-2) and OsOTS1-OX (3-2; 4-2) transgenic lines were selected for further analyses after confirming knockdown and up-regulation of the transcript level of OsOTS1 and 2 in the respective genetic background (Supplemental Fig. S4). To rule out any off target silencing, we performed qRT-PCR to analyze the expression of the gene encoding the most similar SUMO protease (Loc1g53630) to OTS1 and 2. The qRT-PCR data indicated that Loc1g53630 gene expression was not affected in the OTS RNAi lines (Supplemental Fig. S5), demonstrating that the RNAi-mediated gene silencing was specific to OTS SUMO proteases.
The salt tolerance of the different transgenics was compared using the salt-induced (150 mm NaCl) growth inhibition assay of fast-growing young seedlings as a parameter (Chen et al., 2013). At the 10-d-old seedling stage, no significant morphological differences were observed between the OsOTS1-OX and OsOTS-RNAi lines and control empty vector only under unstressed conditions (Fig. 3A). However, the same lines grown in 150 mm NaCl showed significant differences in sensitivity to salt (Fig. 3B) in terms of seedling growth. In particular, shoot growth of OsOTS-RNAi lines was significantly inhibited as measured by seedling height (64%) and fresh weight (45%) compared to control, wild-type Nipponbare transformed with empty vector (vector only; Fig. 3C). However, even though there was no clear difference in shoot lengths between OsOTS1-OX and empty vector only transgenics, OsOTS1-OX lines had significantly greater fresh weight compared to vector-only (16%) or OsOTS-RNAi lines (56%; Fig. 3D). These results indicate that OsOTS1 has a critical role in ameliorating rice seedling growth inhibition in high salinity. Significantly, overexpression of OsOTS1 confers salt tolerance by attenuating the shoot growth inhibition during salt stress in rice.
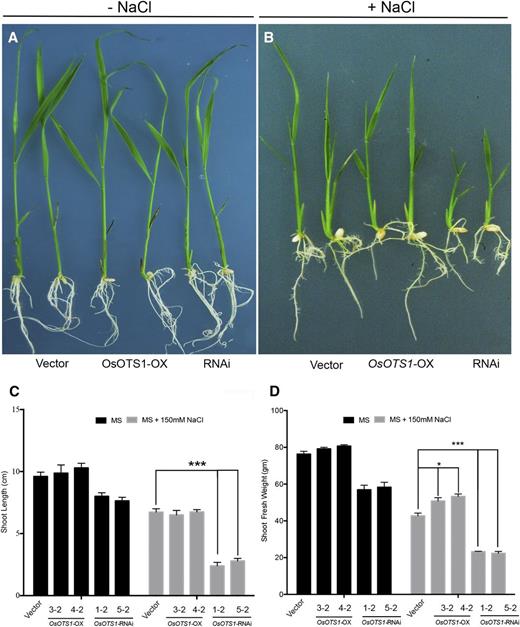
Phenotypic analysis of OsOTS-RNAi and OsOTS1-overexpressing lines under salt stress. Images of 10-d-old rice seedlings of various genotype in MS medium without salt (−NaCl; A) and MS medium with 150 mm NaCl (+NaCl; B). Shoot length (C) and shoot fresh weight (D) of 10-d-old plants grown in liquid MS medium (with or without 150 mm NaCl). Each column represents an average of three individual biological replicates with 20 seedlings in each experiment. Error bars indicate sd. P values for differences between empty vector only and the transgenic lines: *P < 0.05 and ***P < 0.0001 (two-way ANOVA test).
Another clear indicator of sensitivity in high salinity conditions in rice is the inactivation of photosynthesis, and this is correlated with the ability to retain leaf chlorophyll content (Kura-Hotta et al., 1987). We examined the chlorophyll content of floating leaf discs of the transgenic lines exposed for 72 h to varying concentrations of NaCl. When leaf discs were floated separately on different concentrations of NaCl for 72 h, the damage caused by salt stress was evident from the degree of bleaching observed in the leaf tissue (Fig. 4A). Chlorophyll content estimation was performed to confirm the leaf disc assay results. Only OsOTS1-OX transgenic leaf segments retained higher levels of chlorophyll (∼32–40%) compared to the vector-only plants in salt. OsOTS-RNAi lines showed the greatest loss of chlorophyll content, indicating rapid inhibition of photosynthesis (Fig. 4B).
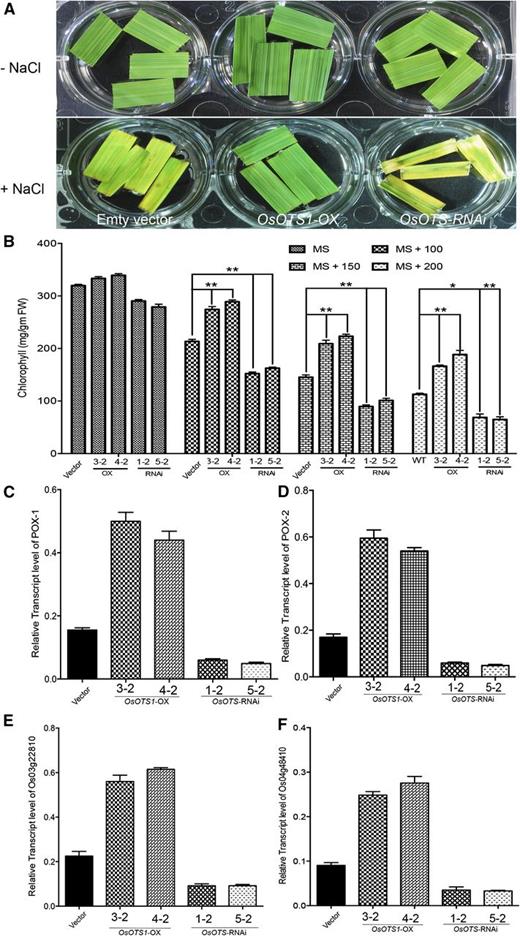
Increased chlorophyll retention and up-regulation of genes encoding antioxidants in OsOTS1-OX rice. A, Representative images indicate chlorophyll retention in leaf discs from unstressed and salt (NaCl)-stressed empty vector, OsOTS1-OX, and OsOTS-RNAi transgenic plants. B, Quantification of chlorophyll content at different concentrations of salt. Relative expression of LOC_Os04g59160 (C), LOC_Os07g47990 (D), LOC_Os03g22810 (E), and LOC_Os04g48410 (F). Total RNA was prepared from the 10-d-old seedlings of the vector-only and transgenic lines and then reverse transcribed. Transcript levels were measured by qPCR with cDNA. Actin was used as an internal control. Data represent the mean values of three independent biological replicates. Error bars indicate sd. P values for differences between empty vector only and the transgenic lines: *P < 0.05 and **P < 0.001 (two-way ANOVA test).
Since OsOTS1-OX plants were able to retain more chlorophyll under stress, we wanted to ascertain whether this was due to increased antioxidant activity, which provides protection against free radical damage. Peroxidases and superoxide dismutases are two principal indicators of plant antioxidant system (Mittler, 2002; Asano et al., 2012). Real-time PCR data indicated that in 2-week-old seedlings, leaf tissue treated with 150 mm NaCl for 12 h, rice peroxidase genes POX-1 (LOC_Os04g59160) and POX-2 (LOC_Os07g47990) as well as the superoxide dismutase genes (LOC_Os03g22810 and LOC_Os04g48410) were up-regulated in OsOTS1-OX but down-regulated in OsOTS-RNAi transgenic plants compared with empty vector only (Fig. 4, C–E). This evidence suggests that OsOTS1 increases rice salt tolerance at least in part by the activation of antioxidant defenses in the shoot.
OsOTS1 Promotes Root Growth under Salt Stress by Modulating the Expression of Root Growth-Promoting Genes
Although OsOTS1-OX rice shoots possess more antioxidant capability and more fresh weight, the shoot length was not significantly greater than vector-only controls under salt stress. We wanted to determine whether this phenotype was also reflected in the roots of OsOTS1 transgenics. Interestingly, under salt stress, both root length and fresh weight were significantly higher in OsOTS1-OX lines compared to controls. However, OsOTS-RNAi lines showed the greatest decrease in both root length (58%) and root fresh weight (58%), indicating significant seedling root growth inhibition in salt (Fig. 5, A–H). This is in contrast to what was observed in shoots. These data suggested that OsOTS1 had a greater role in root growth and development during high salinity.
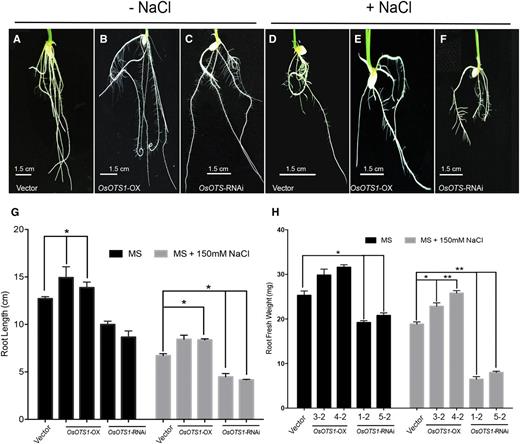
OsOTS-RNAi roots are highly sensitive to salt. Root images of vector-only control, OsOTS1-OX, and OsOTS-RNAi seedlings grown in liquid MS medium (in absence of NaCl; A–C) or in presence of 150 mm NaCl (D–F). OsOTS-RNAi showed dramatically reduced root growth. G, Quantification of root length and root fresh weight of 10-d-old plants in MS and MS with 150 mm NaCl. Each column represents an average of five independent biological replicates with 20 seeds in each experiment. Error bars indicate sd. P values for differences between empty vector only and the transgenic lines: *P < 0.05 and **P < 0.001 (two-way ANOVA test).
To obtain further insight into the function of OsOTS1 in adult rice plants, we grew the different transgenics in soil for 45 d without salt treatment. The robustness of the effects of OsOTS1-OX was confirmed by the increase in root length and fresh weight of these soil-grown rice plants (Fig. 6). These data suggest that deSUMOylation controlled by OsOTS1 may preferentially target the factors that affect root development in rice.
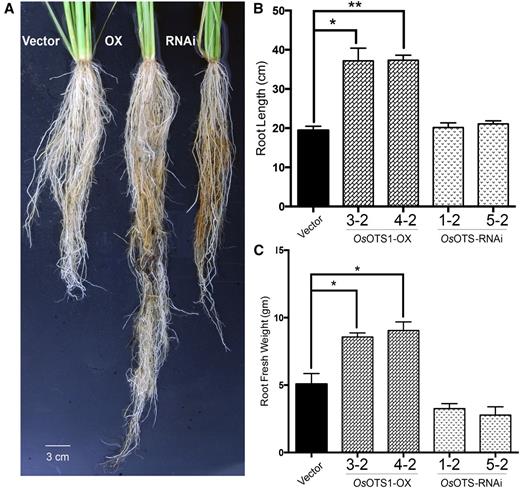
Enhanced root growth of soil grown adult OsOTS1-OX rice plants. A, Rice grown in sandy soil for 45 d and uprooted for root development analysis. Root images are representative from five independent plants of each genotype. Quantification of root length (B) and root fresh weight (C) in sandy soil-grown empty vector, OsOTS1-OX, and RNAi lines at 45 d. Error bars indicate sd values for differences between the different transgenic lines compared to empty vector only. *P < 0.05 and ***P < 0.0001 (two-way ANOVA test).
Since we have established that OsOTS1 SUMO protease may target the promotion of root growth as a probable mechanism for boosting salt tolerance in rice roots, we wanted to determine if this is mediated by the up-regulation of key genes that control root growth. RSS1, RSS3, IAA23, and DRO1 have recently been shown to be involved in affecting rice root development during stress (Jun et al., 2011; Ogawa et al., 2011; Toda et al., 2013; Uga et al., 2013). Indeed, qRT-PCR analysis performed after 6 h of salt treatment on the rice transgenics indicated that all of these genes were up-regulated in OsOTS1-OX lines but suppressed in the OsOTS-RNAi lines when compared to controls (Fig. 7). Our data demonstrate a novel mechanism whereby OsOTS1 SUMO protease affects root growth and development during salt stress in rice roots by inducing the expression of genes involved in promoting root growth.
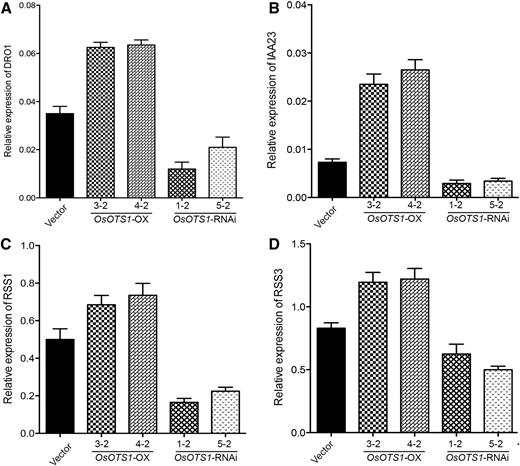
OsOTS1 affects the expression of root growth promoting genes. Ten-day-old rice seedlings were transferred to MS medium with 150 mm NaCl and harvested after 6 h of salt treatment to extract total RNA. The expression of different genes was determined by qPCR. Gene expressions of target genes were normalized to that of rice actin. The relative expression was compared with that of control plants. Mean values were obtained from three biologically independent experiments. Error bars indicate sd.
OsOTS1 Is a Nuclear Protein That Is Rapidly Degraded during Salt Stress in Rice
Previously, we showed that AtOTS1 and AtOTS2 are functionally redundant in Arabidopsis with respect to salt stress responses. Although both AtOTS1 and 2 are localized to the nucleus, the near identical partners differ in that AtOTS1 nucleoplasm localized but the near identical AtOTS2 was predominantly present in the nucleus as speckles (Conti et al., 2008). In rice, we wanted to ascertain the subcellular localization of OsOTS1 to determine its site of action in salt stress responses in rice. We transiently expressed YFP-OsOTS1 in Nicotiana benthamiana leaves and examined cellular YFP fluorescence by confocal laser scanning microscopy. Fluorescence of YFP-OsOTS1 was detected only in the nuclei of all cells examined but not in any speckle structures (Fig. 8A), while the DNA constructs expressing only YFP showed fluorescence in the nucleus and cytoplasm (Fig. 8A). Immunoblotting experiments with anti-GFP antibodies that cross-react with YFP confirmed the presence of YFP-OsOTS1 fusion proteins (Supplemental Fig. S6). These data confirmed that OsOTS1 like AtOTS1 is present throughout the nucleoplasm.
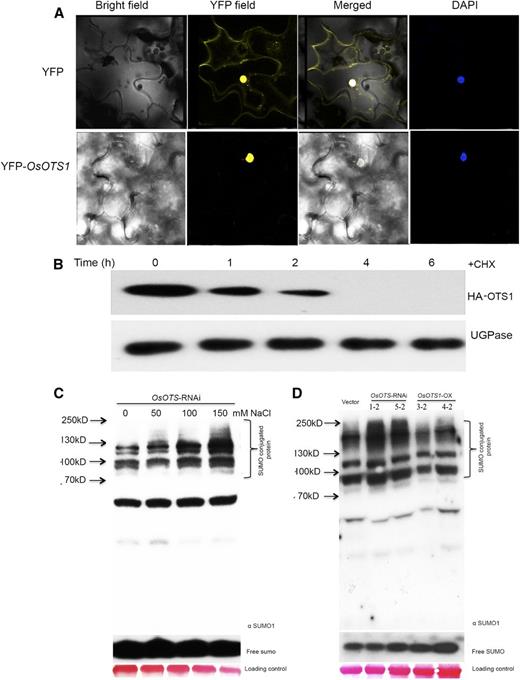
Subcellular localization of OsOTS1 and its stability under salt stress affects the accumulation of SUMO conjugates. A, OsOTS1-YFP was transiently expressed via agroinfiltration of N. benthamiana. B, Stability of OsOTS1 in the presence of 150 mm NaCl with 100 µm cyclohexamide (CHX) treatments as indicated. Ten-day-old rice seedlings were used for the assays. UGPase level was used as a loading control. C, Accumulation of SUMO conjugates in OsOTS-RNAi lines with different salt concentrations as detected with SUMO1 antibody. D, Accumulation of SUMO conjugates in vector-only, OsOTS1-OX, and OsOTS-RNAi lines under salt stress as detected with SUMO1 antibody. Ponceau staining of total proteins was used as a loading control. Molecular weights are indicated next to black arrows.
Our data indicate that the control of SUMOylation by OsOTS1 affects salt tolerance in rice and it is possible that the levels or the activity of OTS1 itself may be affected by salt stress. To determine the effect of salt stress on OsOTS1 protein stability, we treated 10-d-old transgenic HA:OsOTS1 seedlings with 150 mm of NaCl in the presence of cyclohexamide to rule out any involvement of new protein synthesis. We observed that after 2 h HA-OsOTS1 protein levels were significantly reduced and could not be detected at 4 h by anti-HA antibodies. In contrast, control UDP-Glc pyrophosphorylase (UGPase) protein was stable throughout the salt treatment. Our data demonstrate that rice plants trigger the rapid degradation of OsOTS1 as an early response to salt stress (Fig. 8B).
OsOTS1 Regulates SUMO1 Deconjugation in Vivo
In Arabidopsis, the conjugation of two nearly identical SUMO isoforms, AtSUMO1 and AtSUMO2, to target proteins dramatically increases upon a number of abiotic stresses, including salt stress (Kurepa et al., 2003; Catala et al., 2007; Miura et al., 2007a; Conti et al., 2008). Previous data demonstrated that anti-AtSUMO1 antibody cross-reacted with OsSUMO1 in rice (Park et al., 2010) and a dose-dependent increase in SUMO conjugates was observed in wild-type cv Nipponbare plants. We wanted to determine if we could observe a similar effect on OsSUMO1/2 protein conjugation after salt stress treatment in OsOTS1RNAi lines. Total proteins from 2-week-old rice OsOTS1-RNAi seedlings grown in the presence of increasing salt concentration were resolved on SDS-PAGE, and any changes in the SUMOylation pattern were monitored by immunoblotting with anti-AtSUMO1/2 antibodies (Fig. 8C). In the absence of supplemented salt, OsOTS1-RNAi rice plants showed a consistent pattern of anti-SUMO1/2 hybridization consisting of major bands at ∼55, 100, and ∼120 kD along with an ∼17-kD band, corresponding to free OsSUMO1/2. Importantly, very few high M r (above 130 kD) SUMO conjugates were detectable, which is consistent with previous reports of relatively high levels of deSUMOylation activity within cells (Kurepa et al., 2003). Upon salt stress treatment in OsOTS1-RNAi lines, we observed an accumulation of SUMO-conjugated proteins higher than 130 kD, and this was directly dependent on the salt concentration in the growth media (Fig. 8C). Overall, we were able to demonstrate that salt stress leads to SUMOylation of cellular proteins in a dose-dependent manner in the OTS1-RNAi lines. Taken together with the degradative effect of salt on OsOTS1, the hyperaccumulation of SUMO conjugates in OsOTS1-RNAi rice on high salinity is likely due to the loss of OsOTS1-dependent SUMO deconjugation within cells.
Conversely, we wanted to determine if the salt-tolerant phenotype conferred by OsOTS1 overexpression was due to enhanced in vivo deSUMOylation. This was achieved by analyzing the SUMOylation status of the rice OsOTS1 transgenics. SUMOylated proteins from 10-d-old seedlings of the different transgenic lines grown in liquid Murashige and Skoog (MS) medium with 150 mm salt were extracted and analyzed by immunoblotting with anti-SUMO1 antibodies. As expected, we observed an increased abundance of high M r SUMO conjugates in OsOTS-RNAi lines compared to empty vector but reduced SUMO conjugates in OsOTS1-OX transgenic lines (Fig. 8D). Furthermore, we also detected more free SUMO levels in OsOTS1-OX lines, indicating increased release of SUMO from their cognate target proteins. Our results indicate that increased OsOTS1 activity induces salt tolerance by reducing SUMO1/2-dependant SUMOylation levels in rice.
DISCUSSION
As sessile organisms, plants have evolved sophisticated rapid response mechanisms to adapt to environments where they often face a combination of different biotic and abiotic stresses. Understanding these mechanisms will add to the yield potential of crop plants such as rice. Previously we demonstrated that SUMO conjugation on target proteins is one such mechanism that plays a vital role in regulating Arabidopsis growth under salt stress by revealing the role of the SUMO proteases AtOTS1 and 2 (Conti et al., 2008, 2014). However, until now very little information exist on the role of SUMO in crop plants such as rice.
As a first step to understanding the role of SUMO in stress tolerance in crops, we identified the ULP class of SUMO proteases in rice. Like Arabidopsis, the expansion of the SUMO protease gene family in rice compared to the E3 ligases indicates the reliance of plants on deconjugation as a selective mechanism for the control of molecular pathways.
We demonstrated the SUMO protease activity for the ortholog OsOTS1 from rice and show that it has a crucial role in salinity tolerance in rice. Unlike AtOTS1, OsOTS1 expression is downregulated in heat, cold, and salt stress, indicating that rice stimulates the conjugation of SUMO onto target proteins as a response to abiotic stresses by down-regulating the gene expression of OsOTS1. These data also indicate that in rice the control of gene expression of orthologous SUMO proteases is regulated differently. We demonstrated that OsOTS1 protein possesses ability to cleave SUMOylated substrates in vitro (Fig. 2A). This evidence is validated in vivo by the hyperaccumulation of SUMO1-conjugated proteins in transgenic rice plants deficient in OsOTS1/2. When treated with high salt, OsOTS1/2-deficient rice overaccumulates SUMO conjugates (Fig. 8C). These transgenic rice plants are also significantly more growth sensitive in high salinity when compared to empty vector rice transgenics. This is the first evidence directly linking the accumulation of SUMO conjugated proteins to salt sensitivity in crop plants.
Correspondingly, OsOTS1-overexpressing rice plants performed better than wild-type plants under salinity conditions at the vegetative stage. Intriguingly, transgenic OsOTS1-overexpressing rice showed no obvious morphological difference from empty vector plants under nonstressed conditions (Fig. 3). The salinity-induced loss of chlorophyll was significantly less in independent OsOTS1-overexpressing lines compared with OsOTS-RNAi lines and wild-type plants (Fig. 4). We attributed this tolerance to the up-regulation of the antioxidant system. Activation of antioxidant levels is a typical response to a plethora of stresses that stimulate the production of active oxygen species (Apel and Hirt, 2004). There maybe two plausible mechanisms for how OsOTS1/2 may activate cellular antioxidant system; peroxidases and dismutases do possess predicted SUMO attachment sites. During stress, these enzymes may be deactivated or destabilized by SUMO conjugation. OsOTS1 may directly deSUMOylate them to restore their activity and hence prevent free radical damage. Another mechanistic possibility for the role of OsOTS1 in the nucleus is the stress-dependent deSUMOylation of transcriptional regulators that control the expression of genes encoding components of the cellular antioxidant system. Both mechanisms are not mutually exclusive.
Root system architecture is important for crop yield under stress, particularly in the salt sensitive rice crop. It is well established that stresses such as drought, salinity, and nutrient deficiencies modify root system architecture in rice (Nakashima et al., 2007; Ogawa et al., 2011). However, the underlying mechanisms have not been defined. As part of our effort to decipher the function of SUMO in rice, we discovered that the OsOTS1 SUMO protease-deficient rice roots are nearly 50% shorter under salt stress. Conversely, OsOTS1-OX lines were significantly larger, indicating substantial tolerance conferred by OsOTS1 in rice to salinity (Fig. 5, A–H). This effect on root development was evident in soil grown rice plants even without stress, indicating that unlike Arabidopsis rice OsOTS1 has a role in root development in the absence of high salinity. These data indicate that the SUMO-conjugated targets of OsOTS1 SUMO protease will be critical players in root development per se in rice. However, the identity of these targets and the mechanistic consequence of SUMOylation are beyond the scope of this study.
Gene expression analysis showed that OsOTS1-OX lines had a dramatic up-regulation of key genes involved in the control of cell size particularly in the roots (Jun et al., 2011; Ogawa et al., 2011; Toda et al., 2013; Uga et al., 2013), while these genes were suppressed in the OsOTS-RNAi lines (Fig. 7). Taken together, our data indicate that rice OTS1 acts as a positive regulator of root development. Our study reveals a novel mechanism mediated by SUMO for the control of root growth in rice during salt stress.
Intriguingly, as a natural response to salt, rice induces the degradation of OsOTS1, in effect generating an OTS1 deficient environment in the cell akin to the OsOTS-RNAi lines. This results in the hyperaccumulation of SUMO conjugates and, as demonstrated here, leads to reduced growth. The static nature of plants dictates that growth must be integrated with changes in the natural environment. Recent evidence indicates that an important strategy employed by plants to survive adverse conditions is to restrain growth via DELLA accumulation (Achard et al., 2006; 2008a, 2008b; Navarro et al., 2008; Hou et al., 2010). DELLA proteins act as integrators of multiple environmental cues by repressing molecular pathways governed by the growth promoting phytohormone gibberellin (Peng et al., 1997; Silverstone et al., 1997; Peng et al., 1999; Ikeda et al., 2001). The integrative role of DELLAs is heavily reliant on the plant’s ability to control DELLA protein levels. Previously, we demonstrated a key role for SUMOylation in stabilizing DELLA proteins under stress conditions (Conti et al., 2014). During stress, SUMOylation of DELLA proteins increases, leading to their accumulation to exert growth restraint. AtOTS1 SUMO protease is critical for destabilizing DELLA proteins by removing SUMO. OsOTS1 protease is rapidly degraded upon salt stress in rice, leading us to speculate that like in Arabidopsis, OsOTS1 degradation may contribute to hyperSUMOylation and accumulation of the rice DELLA protein SLR1 and consequently causing beneficial growth restraint. SUMOylation may therefore act as a rapid growth retardation mechanism (“growth brakes”) at the onset of stress in rice. We can speculate that in OsOTS1-OX plants, increased deSUMOylation may play a role in promoting growth by attenuating growth retardation mechanisms during stress.
Although the SUMO system has been characterized and demonstrated to play important roles in growth and development in Arabidopsis, in crops, its role is poorly understood. We show that manipulating SUMO deconjugation from target proteins has a significant impact on rice crops to cope with salinity. It will be imperative to isolate, identify, and characterize targets and interacting partners of OsOTS1 to better understand how the SUMO system imparts salinity stress tolerance in rice. SUMOylation may refocus multiple salt stress signaling pathways by targeting key nodes within this pathway and thereby enact growth restraint observed as salt sensitivity. Therefore, SUMO could provide a tool to uncover regulatory nodes within stress pathways. Our study thus paves the way for developing salt tolerant rice varieties based on the SUMO system.
MATERIALS AND METHODS
Plant Materials and Treatments
Seeds of rice (Oryza sativa cv Nipponbare) were sown in pots (8 × 8 × 10 cm) containing 180 g of soil soaked with water. All plants were grown under white fluorescent light (600 photons m−2 s−1, 14 h of light/12 h of dark) at 27°C ± 1°C/24°C ± 1°C and 60% relative humidity. After 4 d of growth, the seedlings were transferred to beakers containing cotton soaked in water, and for treatments, water containing the desired solute was used. Sodium chloride was added as required (50–150 mm). Abscisic acid (Sigma-Aldrich) was dissolved in DMSO to make a stock of 10 mm and was diluted further in water. For cold stress, the seedlings were maintained at 4°C ± 1°C. Desiccation was simulated by treating the seedlings with 10% polyethylene glycol.
Phylogenetic Analysis
Alignment was performed using the webPRANK algorithm by embl-ebi and edited in Jalview (Löytynoja and Goldman, 2010). The evolutionary history was inferred by using the maximum likelihood method based on the Le_Gascuel_2008 model (Le and Gascuel, 2008). The percentage of replicate trees in which the associated taxa clustered together in the bootstrap test (1,000 replicates) is shown next to the branches (Felsenstein, 1985). Initial tree for the heuristic search was obtained by applying the neighbor-joining method to a matrix of pairwise distances estimated using a JTT model. A discrete Gamma distribution was used to model evolutionary rate differences among sites [five categories (+G, parameter = 1.7715)]. The rate variation model allowed for some sites to be evolutionarily invariable ([+I], 6.6522% sites). The analysis involved 17 amino acid sequences. All positions containing gaps and missing data were eliminated. There were a total of 85 positions in the final data set. Evolutionary analyses were conducted in MEGA6 (Tamura et al., 2013).
Quantification of Gene Expression
Total RNA was isolated using the RNA isolation kit (Sigma-Aldrich). After RNase-free DNase treatment, 2 μg RNA was reversibly transcribed using oligo(dT) primer and reverse transcriptase II. Quantitative PCR was performed on the Qiagen Rotor Gene Q using Sigma SYBR Green qPCR mix. The results were analyzed using software (Qiagen Rotor Gene Q). Each data set had three replicates and the experiments were repeated twice. Primers are listed in Supplemental Table S1.
Plasmid Construction and Generation of Transgenic Plants
The full-length OsOTS1 cDNA sequences were amplified from cv Nipponbare cDNA and subcloned into a binary vectors pIPKb002 for overexpression under the control of ubiquitin promoter. To generate the OsOTS1 RNAi plants, a 436-bp cDNA fragment was amplified with the primers indicated in Supplemental Table S1 and cloned into the Gateway entry vector D-Topo. The RNAi cassette was then recombined into the pIPKb027 vector (Himmelbach et al., 2007) using Gateway L/R clonase II (Invitrogen). Each of the two constructs was introduced into Agrobacterium tumefaciens strains EHA105 and then transformed into rice (O. sativa cv Nipponbare) as described by Nishimura et al. (2006) with slight modification to the protocol. The transgenic rice plants that showed the single-copy insertion in T0 and 3:1 segregation ratios in the T1 were used in this study.
Subcellular Localization Assays
To determine the subcellular localization of OsOTS1, the full-length OsOTS1 cDNA sequences were amplified and subcloned into the pEarlyGATE103-YFP vector, in which YFP coding sequence was fused in frame to the 3′ end of the OsOTS1 gene sequence. Primers are listed in Supplemental Table S1. The constructs were transformed into Agrobacterium and infiltrated into tobacco leaves for confocal microscopy. The subcellular localization of YFP was visualized using a confocal laser scanning microscope (Leica SP5 CLSM) with ×63 objective lenses as previously described (Lee et al., 2015). The 4′6-Diamino-2-phenylindole (DAPI) staining was carried out by infiltration of leaves with 10 μg mL−1 DAPI in 10 mm MgCl2, 20 min before imaging. DAPI was excited at 405 nm and transmission was collected between 420 and 470 nm.
Recombinant Proteins and in Vitro SUMO Protease Assay
Purification of recombinant protein and SUMO protease assays was performed as described before (Conti et al., 2008) with slight modification to the protocol. OsOTS1 cDNA was cloned into the expression vector (pDEST-17-His). All the constructs and empty vectors were transferred into Escherichia coli BL21 (D3E) cells. E. coli cultures (50 mL) were grown at 37°C to an OD600 of 0.8 before adding isopropylthio-β-galactoside at a final concentration of 1 mm to induce protein expression at 28°C for 2 h. Cells were pelleted and resuspended in Bugbuster (Novagen). The soluble protein fraction (supernatant) was recovered, and its concentration was determined with Millipore direct detect spectrometer. Poly-His:SUMO1:FLC (Murtas et al., 2003) was produced in E. coli with affinity chromatography using His columns (GE-Healthcare) according to the manufacturer’s instructions and eluted in 5 mL of elution buffer. The eluate was dialyzed overnight at 4°C against SUMO protease buffer (50 mm Tris-HCl, pH 7.9, 150 mm NaCl, 0.1% [v/v] Triton X-100, and 2 mm DTT).
The SUMO protease assay was performed by mixing 20 μg of substrate poly-His:SUMO1:FLC and OSOTS1 SUMO protease. The reaction mixture was incubated in SUMO protease buffer for 6 h at 30°C. The reactions were stopped by adding 4× SDS-PAGE loading buffer, and proteins were resolved on a 15% SDS-PAGE gel. Proteins were transferred to polyvinylidene difluoride membranes for immunoblot analysis.
Measurement of Chlorophyll Content and Leaf Disc Assay
About 100 mg of flag leaves excised to 2 to 3 cm in length was immersed in extract solution (95% ethanol + 5% water) at room temperature (25°C) until the leaves were bleached. The total chlorophyll content was assayed by measuring the absorbance of the extracts at 647 and 665 nm (Inskeep and Bloom, 1985). One-centimeter plant flag leaf segments were excised and floated on 0, 50, 100, and 150 mm NaCl solution for 72 h, and at the end, chlorophyll retention in each sample was calculated.
Protein Extraction and Immunoblotting
Rice seedlings were frozen in liquid nitrogen and homogenized in extraction buffer (PBS, pH = 7.4) with EDTA-free protease inhibitor cocktail (Roche). The homogenate was microcentrifuged at 13,000g for 10 min at room temperature, and the supernatant was quantified with Millipore direct detect spectrometer before mixing with SDS-PAGE loading buffer. Equal amounts of proteins for each sample were loaded onto standard SDS-PAGE gel. Proteins were then transferred to a polyvinyl difluoride membrane (Bio-Rad) for immunoblot analysis. Filters were blocked in TBST-milk (5% [w/v] dry nonfat milk, 10 mm Tris-HCl, pH 8, 150 mm NaCl, and 0.1% [v/v] Tween 20) before incubation with primary antibody anti-SUMO1 (Abcam) diluted 1:2,000. Filters were washed in TBST and incubated with secondary antibody (anti-rabbit horseradish peroxidase conjugate; Sigma-Aldrich) diluted 1:10,000 in TBST-milk. To detect HA tag proteins, filters were incubated with peroxidase antiperoxidase (Sigma-Aldrich) diluted 1:10,000. Filters were washed and incubated with the horseradish peroxidase substrate (Immobilon western; Millipore) before exposure to film (Kodak). UGPase, a cytosolic protein (Martz et al., 2002), was detected with anti-UGPase rabbit polyclonal antibodies (Agrisera) diluted 1:3,000 followed by anti-rabbit alkaline phosphatase-conjugated antibodies diluted 1:5,000. Membranes were then submerged to luminol mix and incubate for 2 min followed by x-ray film exposure.
Accession Numbers
Sequence data from this article can be found in the Rice Genome Annotation Project (http://rice.plantbiology.msu.edu/) data libraries under the following accession numbers: OsOTS1 (LOC_Os06g29310) and OsOTS2 (LOC_Os12g41380).
Supplemental Data
The following supplemental materials are available.
Supplemental Figure S1. Full-length protein alignment of putative rice SUMO proteases with three known Arabidopsis and two known yeast SUMO proteases.
Supplemental Figure S2. Transcript analysis of OsOTS1 in rice plants subjected to heat (42°C), cold (4°C), salt (150 mm NaCl), PEG (10%), and ABA (100 µm) treatment.
Supplemental Figure S3. Transcript analysis of HptR (hygromycin phosphor transferase) gene was analyzed to determine copy number.
Supplemental Figure S4. Transcript analysis of OsOTS1 in vector only, OsOTS1-OX, and RNAi transgenic rice plants.
Supplemental Figure S5. Transcript analysis of Os12g41380 (OsOTS2) and its nearest homolog Os01g53680 in RNAi transgenic rice plants.
Supplemental Figure S6. The expression of the OsOTS1-YFP fusion proteins was confirmed by immunoblotting with anti-GFP antibody.
Supplemental Table S1. Primers used in the study.
LITERATURE CITED
Author notes
This research was supported by a European Research Council grant to A.S.
Address correspondence to [email protected].
The author responsible for distribution of materials integral to the findings presented in this article in accordance with the policy described in the Instructions for Authors (www.plantphysiol.org) is: Ari Sadanandom ([email protected]).
A.K.S. and A.S. designed the research; A.K.S., C.Z., G.Y., M.B., and A.B. carried out the experiments; A.K.S. and A.S. analyzed the data and wrote the article.
Articles can be viewed without a subscription.