-
PDF
- Split View
-
Views
-
Cite
Cite
Nielda K.G. Melo, Ricardo E. Bianchetti, Bruno S. Lira, Paulo M.R. Oliveira, Rafael Zuccarelli, Devisson L.O. Dias, Diego Demarco, Lazaro E.P. Peres, Magdalena Rossi, Luciano Freschi, Nitric Oxide, Ethylene, and Auxin Cross Talk Mediates Greening and Plastid Development in Deetiolating Tomato Seedlings , Plant Physiology, Volume 170, Issue 4, April 2016, Pages 2278–2294, https://doi.org/10.1104/pp.16.00023
- Share Icon Share
Abstract
The transition from etiolated to green seedlings involves the conversion of etioplasts into mature chloroplasts via a multifaceted, light-driven process comprising multiple, tightly coordinated signaling networks. Here, we demonstrate that light-induced greening and chloroplast differentiation in tomato (Solanum lycopersicum) seedlings are mediated by an intricate cross talk among phytochromes, nitric oxide (NO), ethylene, and auxins. Genetic and pharmacological evidence indicated that either endogenously produced or exogenously applied NO promotes seedling greening by repressing ethylene biosynthesis and inducing auxin accumulation in tomato cotyledons. Analysis performed in hormonal tomato mutants also demonstrated that NO production itself is negatively and positively regulated by ethylene and auxins, respectively. Representing a major biosynthetic source of NO in tomato cotyledons, nitrate reductase was shown to be under strict control of both phytochrome and hormonal signals. A close NO-phytochrome interaction was revealed by the almost complete recovery of the etiolated phenotype of red light-grown seedlings of the tomato phytochrome-deficient aurea mutant upon NO fumigation. In this mutant, NO supplementation induced cotyledon greening, chloroplast differentiation, and hormonal and gene expression alterations similar to those detected in light-exposed wild-type seedlings. NO negatively impacted the transcript accumulation of genes encoding phytochromes, photomorphogenesis-repressor factors, and plastid division proteins, revealing that this free radical can mimic transcriptional changes typically triggered by phytochrome-dependent light perception. Therefore, our data indicate that negative and positive regulatory feedback loops orchestrate ethylene-NO and auxin-NO interactions, respectively, during the conversion of colorless etiolated seedlings into green, photosynthetically competent young plants.
Chloroplast biogenesis and maturation are critical for plant growth and development, because this organelle is responsible for photosynthesis and many other essential metabolic pathways. During seedling development, chloroplasts may differentiate directly from the plastid progenitor known as proplastid or from the dark-grown transitory form, the etioplast (Pogson and Albrecht, 2011). Considering the fact that under most natural conditions light is unavailable or insufficient during the initial development of germinating seedlings, the formation of etioplasts and their subsequent differentiation into chloroplasts is of high adaptive value for most, if not all, terrestrial seed plants. Facilitating the prompt differentiation into functional chloroplasts, etioplasts typically accumulate thylakoid lipids and large amounts of the chlorophyll precursor protochlorophyllide (Pchlide) bound to Pchlide NADPH-oxidoreductase, forming a semicrystalline, membranous structure known as prolamellar body (PLB; Von Wettstein et al., 1995).
Unarguably, light perception and signaling represent the master switch for the etioplast-to-chloroplast conversion, controlling the transcription of regulatory genes, whose products regulate the synthesis of chlorophylls and photosynthetic apparatus components, ultimately leading to the final structural configuration and biochemical composition of mature chloroplasts (Waters and Langdale, 2009). Among these light-induced elements, GOLDEN2-LIKE (GLK) genes, which encode GARP transcription factors, are known to coordinate the expression of many photosynthesis-related genes, promoting chloroplast maturation and maintenance (Waters et al., 2009; Powell et al., 2012).
The shift from skotomorphogenic to photomorphogenic development relies on the combined action of phytochromes and cryptochromes, which are the two photoreceptor families mainly responsible for gene expression reprogramming in deetiolating seedlings (Jiao et al., 2007; López-Juez et al., 2008; Franklin and Quail, 2010). Photoactive holophytochromes are formed when phytochrome apoproteins, encoded by a small nuclear gene family, become covalently linked to the invariant linear tetrapyrrole chromophore phytochromobilin. Therefore, due to the loss of function of all phytochrome types, the phytochromobilin-deficient mutants usually exhibit more severe chloroplast differentiation impairment than type-specific phytochrome mutants (Kendrick et al., 1997).
Active phytochromes are translocated from cytosol to the nucleus, where they bind to phytochrome-interacting factors and target these basic helix-loop-helix transcription factors for degradation (Franklin and Quail, 2010; Leivar and Quail, 2011). In Arabidopsis (Arabidopsis thaliana), losses of particular phytochrome-interacting factors have been shown to disturb photosynthetic protein accumulation and chloroplast development in both dark- and light-grown seedlings (Leivar and Quail, 2011). Moreover, negative regulators of light signal transduction, such as CONSTITUTIVE PHOTOMORPHOGENIC1 (COP1) or DEETIOLATED1 (DET1), have been identified as suppressors of chloroplast development both in cotyledons of dark-grown seedlings and in nonphotosynthetic tissues of light-grown plants (Chory et al., 1989; Chory and Peto, 1990; Deng and Quail, 1992). The interaction of COP and DET proteins among themselves and with other light-regulated proteins, such as UV-DAMAGED DNA-BINDING PROTEIN1 (DDB1) and CULLIN4 (CUL4), gives rise to the so-called COP9 signalosome, which targets photomorphogenesis-promoting factors, such as LONG HYPOCOTYL5 (HY5), for proteasomal degradation (Wei et al., 2008). Therefore, the light-evoked degradation of COP9 signalosome components promotes HY5 accumulation and, consequently, activates the expression of photomorphogenesis-related genes (Wei et al., 2008).
As in Arabidopsis, orthologs of DET1, COP1, DDB1, and HY5, as well as other light signaling components, also have been demonstrated to control plastid biogenesis and development in leaf and fruit tissues of tomato (Solanum lycopersicum; Liu et al., 2004; Davuluri et al., 2005; Kolotilin et al., 2007; Wang et al., 2008). For instance, mutations in HIGH PIGMENT1 (HP1) and HP2, which are the orthologs of AtDDB1 and AtDET1, respectively, render plants with increased chlorophyll and carotenoid content as well as higher chloroplast number and size in both leaf and fruit cells (Mustilli et al., 1999; Cookson et al., 2003; Kolotilin et al., 2007). More recently, manipulation of light signaling components, such as HP1/DDB1, HP2/DET1, COP1, and HY5, has emerged as an efficient strategy to improve plastidial biogenesis and development and, consequently, the nutritional quality of tomato fruits (Liu et al., 2004; Davuluri et al., 2005; Wang et al., 2008).
As explained above, the role of photoreceptors and light signal transduction proteins in chloroplast development has been explored extensively in different plant models. In contrast, few studies have focused on the interplay between photoreceptors and other endogenous signals, such as plant hormones, during the coordination of plastid differentiation with plant development and environmental stimuli (Egea et al., 2010). In this context, an antagonistic relationship between cytokinins and abscisic acid (ABA) during chloroplast differentiation in deetiolating seedlings has been described (Kusnetsov et al., 1998; Kravtsov et al., 2011). Whereas cytokinins activate cotyledon and leaf greening by stimulating plastid biogenesis and development, ABA has been shown to have the opposite effect (Chory et al., 1994; Kusnetsov et al., 1998; Galpaz et al., 2008; Kravtsov et al., 2011; Cortleven and Schmülling, 2015). In contrast to cytokinins and ABA, the influence of auxins and ethylene on plastid biogenesis and etioplast-to-chloroplast differentiation has received considerably less attention. However, an intimate connection between these hormones has been reported during other important events related to seedling deetiolation, such as the regulation of hypocotyl elongation and the formation, maintenance, and opening of the apical hook in eudicotyledons (Symons and Reid, 2003; Van de Poel et al., 2015). Moreover, the biosynthesis and signaling of these hormones also have been shown to change significantly upon illumination (Symons and Reid, 2003; López-Juez et al., 2008; Van de Poel et al., 2015).
Besides plant hormones, other endogenous signal molecules also seem to participate in controlling seedling greening. The gaseous free radical nitric oxide (NO), for instance, has emerged as a stimulatory signal for the acquisition of photomorphogenic traits in different plant species, regulating processes such as seed germination, hypocotyl elongation, and cotyledon and leaf greening (Beligni and Lamattina, 2000; Zhang et al., 2006; Lozano-Juste and León, 2011). During seedling greening, NO seems to intensify the responses to light stimuli, promoting the accumulation of chlorophylls and chloroplast-related proteins (Beligni and Lamattina, 2000; Zhang et al., 2006). Moreover, increased NO production also has been reported in deetiolating Arabidopsis and wheat (Triticum aestivum) seedlings (Lozano-Juste and León, 2011; Liu et al., 2013).
Although NO biosynthesis in plants is still not fully characterized, nitrate reductase (NR) has been considered one of the most likely candidates responsible for NO production under physiologically relevant conditions (Planchet and Kaiser, 2006a; Gupta et al., 2011). NR is regulated at both the transcription and posttranslational levels by multiple environmental and endogenous factors (Kaiser and Huber, 2001). Light, for instance, is a critical environmental cue that regulates both NR gene transcription and protein phosphorylation (Lillo and Appenroth, 2001). In the presence of nitrate, NR transcript and protein levels increase dramatically in deetiolating seedlings soon after exposure to illumination. Apparently, such a response mostly depends on light perception via phytochromes (Becker et al., 1992; Goud and Sharma, 1994), which can be mimicked or intensified by the exogenous application of cytokinins and/or auxins (Lu et al., 1992; Yu et al., 1998). As a result, light and plant hormones interact intensively to determine the induction of NR during the deetiolation process.
Based on the intertwined NO-hormone signaling cascades described for numerous plant developmental responses (Freschi, 2013; Sanz et al., 2015), interactions between NO and plant hormones during seedling greening also might be expected. These NO-hormone cross talks might involve not only the influence of NO on phytohormone metabolism, perception, or signal transduction but also the modulation of endogenous NO levels by plant hormones (Freschi, 2013). In this context, by a dedicated biochemical characterization and the use of genetic resources, we investigated the role of NO in plastid development during tomato seedling deetiolation and the regulatory interplay of NO with auxins and ethylene. The results showed that NR-dependent NO production mediates light-evoked greening by regulating the expression of light signaling as well as plastid division and differentiation genes. Moreover, our study uncovered a mutual positive and negative feedback between NO-auxin and NO-ethylene metabolism, respectively, during light-dependent responses.
RESULTS
NR-Derived NO Production Temporally Coincides with Light-Evoked Greening
To gain insight into the possible role of endogenous NO in the regulation of etioplast-to-chloroplast differentiation, the time course of plastid structural changes, photosynthetic pigment accumulation, and NO content fluctuations was evaluated in cotyledons of deetiolating and dark-grown tomato seedlings. Wild-type tomato and two contrasting photomorphogenic mutants were compared: (1) the pale-green, phytochromobilin-deficient mutant aurea (au) and (2) the dark-green, light-hypersensitive mutant hp1 (Carvalho et al., 2011).
Plastids of dark-grown wild-type and au seedlings presented an internal structure typical of etioplasts, exhibiting the typical lattice-like membranous structure of PLBs (Fig. 1). In contrast, dark-grown hp1 tomato seedlings presented semideveloped chloroplasts instead of etioplasts, exhibiting PLBs converted into prothylakoid membranes (Fig. 1) and resembling the plastid structure of dark-grown Arabidopsis cop and det mutants (Chory et al., 1989; Deng and Quail, 1992) and tomato hp2 mutants (Mustilli et al., 1999). The plastid internal membranous structure observed in the wild type and hp1 under red light (RL) or blue light (BL), or in au seedlings under BL, was virtually indistinguishable, with the formation of granal thylakoids, the disappearance of PLBs, and, in some cases, the presence of starch grains. In contrast, chloroplasts of RL-treated au seedlings presented neither granal thylakoids nor PLBs (Fig. 1). Ultrastructural plastid features observed in cotyledon cells of wild-type, hp1, and au seedlings grown under white light resembled those found under BL conditions (Supplemental Fig. S1).
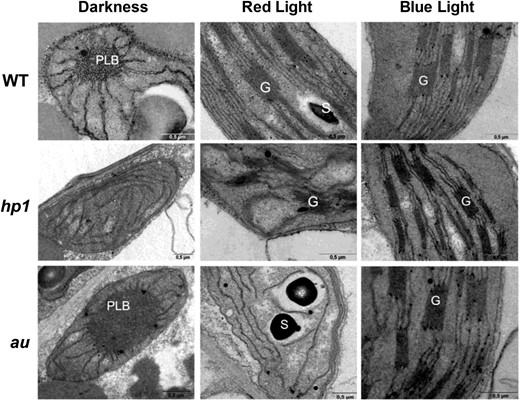
Plastid structure in cotyledon cells of tomato photomorphogenic mutants exposed to distinct light conditions. Wild-type (WT), hp1, and au seedlings dark grown for 120 h were either kept in darkness or transferred to continuous red light (RL) or blue light (BL) treatment for 72 h before ultrastructural analysis. G, Granal thylakoid; S, Starch; PLB, prolamellar body. Bars = 0.5 µm.
In cotyledons of wild-type seedlings, maximum chlorophyll and carotenoid levels were observed 48 h after the start of either RL or BL treatment, remaining relatively stable thereafter (Fig. 2). The time course of pigment accumulation was similar in wild-type and au seedlings exposed to BL. As expected, RL failed to induce photosynthetic pigment accumulation in the phytochromobilin-deficient mutant. Consistent with its light-hypersensitive phenotype, hp1 seedlings exhibited pigment levels considerably higher than the wild type under either RL or BL (Fig. 2).
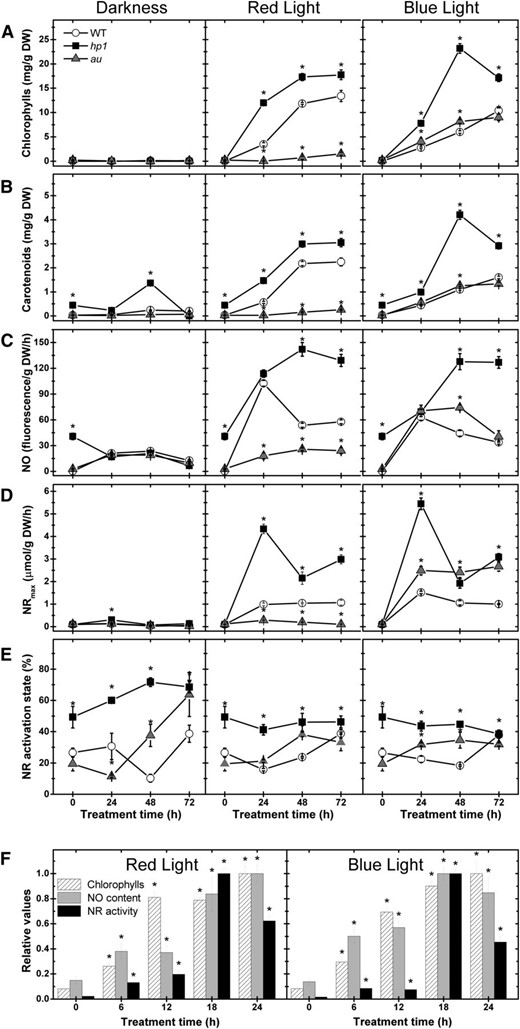
Light-driven tomato seedling greening temporally coincides with the rise in both NO levels and NR activity. Wild-type (WT), hp1, and au seedlings dark grown for 120 h were either kept in darkness or transferred to continuous RL or BL treatment. A, Chlorophylls. B, Carotenoids. C, Fluorometric quantification of endogenous NO release. D, NR maximum activity (NRmax). E, NR activation state. F, Chlorophyll levels, endogenous NO release, and NR maximum activity in wild-type seedlings within the first 24 h of RL or BL exposure. In F, the maximum chlorophyll, NO, and NR values were set to 1 for comparison. Values shown are means ± se. Asterisks indicate statistically significant differences compared with the wild type (in A–E) or compared with the dark treatment (in F) at each sampling time (Student’s t test, P < 0.05). DW, Dry weight.
NO levels in cotyledon tissues of dark-grown and deetiolating wild-type, hp1, and au seedlings were determined using the fluorometric quantification method based on the cell-impermeant NO probe diaminorhodamine-4M (DAR-4M; Fig. 2C) and compared with the maximum activity (Fig. 2D) and activation state (Fig. 2E) of NR. Under continuous darkness, all genotypes exhibited reduced endogenous NO release associated with extremely low levels of maximum NR activity. In contrast, endogenous NO and maximum NR activity dramatically increased in wild-type seedlings during the first 24 h of RL or BL. In au seedlings, NO generation increased exclusively under BL conditions, correlating with the maximum NR activity. The light-hypersensitive mutant hp-1 exhibited the highest values of both NO release and maximum NR activity under either RL or BL, which exceeded by several times the levels detected in wild-type or au seedlings. Regardless of the light treatment, hp-1 seedlings also exhibited slightly higher values of NR activation state, suggesting a negative influence of HP1/SlDDB1 not only on total NR activity but also on NR posttranslational regulation (Fig. 2).
A concomitant and progressive increase in both chlorophyll and NO levels was observed within the first 24 h of either RL or BL exposure, with significant increases in both parameters taking place as soon as 6 h of light treatment (Fig. 2F; Supplemental Fig. S2). The highest values of NR activity and endogenous NO release were observed after 18 and 24 h of light treatment, respectively. In contrast, maximum chlorophyll and carotenoid levels were only observed after 48 or 72 h of either RL or BL exposure, respectively (Supplemental Fig. S2). Therefore, whereas the light-driven accumulation of photosynthetic pigments temporally coincided with increases in endogenous NO content within the first 24 h of light exposure, the maximum accumulation of these pigments was clearly preceded by a transitory peak in both NR activity and NO production (Fig. 2F; Supplemental Fig. S2).
This clear temporal correlation among the time courses of NR activity, NO production, photosynthetic pigment accumulation and plastid development (Figs. 1 and 2; Supplemental Fig. S2) was taken as a first line of evidence suggesting the involvement of NR-dependent NO production in light-evoked greening in tomato seedlings. To confirm the relevance of NR as a source of NO in tomato cotyledons, different treatments were conducted to inhibit the activity of this enzyme, including the use of a nitrogen-free medium and two modified Murashige and Skoog (MS) media containing ammonium or Gln as the sole source of nitrogen. All these approaches significantly inhibited both NR activity and reduced the cotyledon NO levels in wild-type seedlings by about 80% under either RL or BL treatment (Table I).
Maximum and actual NR activity and endogenous NO release in wild-type tomato seedlings cultivated in different sources of nitrogen under RL or BL
When present in the medium, nitrogen was always available at a final concentration of 30 mm. Values shown are means ± se. Statistically significant differences between the control and treatments are indicated in boldface (Student’s t test, P < 0.05). n.d., Not detected.
Treatment | Maximum NR Activity | Actual NR Activity | NO Fluorescence | |||
RL | BL | RL | BL | RL | BL | |
µmol NO2 − g−1 dry wt h−1 | g−1 dry wt h−1 | |||||
Control | 0.60 ± 0.04 | 0.87 ± 0.07 | 0.27 ± 0.03 | 0.34 ± 0.03 | 76.9 ± 15.4 | 65.5 ± 2.9 |
Nitrogen free | 0.05 ± 0.01 | 0.03 ± 0.01 | 0.02 ± 0.00 | 0.01 ± 0.00 | 21.0 ± 2.4 | 16.5 ± 0.6 |
Ammonium | 0.05 ± 0.01 | 0.13 ± 0.02 | 0.02 ± 0.01 | 0.04 ± 0.01 | 14.4 ± 1.2 | 14.8 ± 1.6 |
Gln | n.d. | n.d. | n.d. | n.d. | 11.6 ± 1.1 | 11.4 ± 0.9 |
Treatment | Maximum NR Activity | Actual NR Activity | NO Fluorescence | |||
RL | BL | RL | BL | RL | BL | |
µmol NO2 − g−1 dry wt h−1 | g−1 dry wt h−1 | |||||
Control | 0.60 ± 0.04 | 0.87 ± 0.07 | 0.27 ± 0.03 | 0.34 ± 0.03 | 76.9 ± 15.4 | 65.5 ± 2.9 |
Nitrogen free | 0.05 ± 0.01 | 0.03 ± 0.01 | 0.02 ± 0.00 | 0.01 ± 0.00 | 21.0 ± 2.4 | 16.5 ± 0.6 |
Ammonium | 0.05 ± 0.01 | 0.13 ± 0.02 | 0.02 ± 0.01 | 0.04 ± 0.01 | 14.4 ± 1.2 | 14.8 ± 1.6 |
Gln | n.d. | n.d. | n.d. | n.d. | 11.6 ± 1.1 | 11.4 ± 0.9 |
When present in the medium, nitrogen was always available at a final concentration of 30 mm. Values shown are means ± se. Statistically significant differences between the control and treatments are indicated in boldface (Student’s t test, P < 0.05). n.d., Not detected.
Treatment | Maximum NR Activity | Actual NR Activity | NO Fluorescence | |||
RL | BL | RL | BL | RL | BL | |
µmol NO2 − g−1 dry wt h−1 | g−1 dry wt h−1 | |||||
Control | 0.60 ± 0.04 | 0.87 ± 0.07 | 0.27 ± 0.03 | 0.34 ± 0.03 | 76.9 ± 15.4 | 65.5 ± 2.9 |
Nitrogen free | 0.05 ± 0.01 | 0.03 ± 0.01 | 0.02 ± 0.00 | 0.01 ± 0.00 | 21.0 ± 2.4 | 16.5 ± 0.6 |
Ammonium | 0.05 ± 0.01 | 0.13 ± 0.02 | 0.02 ± 0.01 | 0.04 ± 0.01 | 14.4 ± 1.2 | 14.8 ± 1.6 |
Gln | n.d. | n.d. | n.d. | n.d. | 11.6 ± 1.1 | 11.4 ± 0.9 |
Treatment | Maximum NR Activity | Actual NR Activity | NO Fluorescence | |||
RL | BL | RL | BL | RL | BL | |
µmol NO2 − g−1 dry wt h−1 | g−1 dry wt h−1 | |||||
Control | 0.60 ± 0.04 | 0.87 ± 0.07 | 0.27 ± 0.03 | 0.34 ± 0.03 | 76.9 ± 15.4 | 65.5 ± 2.9 |
Nitrogen free | 0.05 ± 0.01 | 0.03 ± 0.01 | 0.02 ± 0.00 | 0.01 ± 0.00 | 21.0 ± 2.4 | 16.5 ± 0.6 |
Ammonium | 0.05 ± 0.01 | 0.13 ± 0.02 | 0.02 ± 0.01 | 0.04 ± 0.01 | 14.4 ± 1.2 | 14.8 ± 1.6 |
Gln | n.d. | n.d. | n.d. | n.d. | 11.6 ± 1.1 | 11.4 ± 0.9 |
In plants, the production of NO from Arg via a nitric oxide synthase (NOS)-like activity also has been linked to NO production during some plant developmental and stress responses (Gupta et al., 2011; Mur et al., 2013). However, treatments with the mammalian NOS inhibitor NG-nitro-l-Arg methyl ester neither affected NO production nor altered the greening process in RL- or BL-exposed wild-type tomato seedlings (Supplemental Fig. S3). Moreover, no significant nitrite accumulation was detected in cotyledons of deetiolating tomato seedlings (data not shown); therefore, physiologically relevant levels of nonenzymatic NO production from nitrite seem unlikely (Bethke et al., 2004).
NO Fumigation Promotes Greening in the Phytochromobilin-Deficient Tomato Mutant
To further investigate the causal relationship between NO and chloroplast differentiation, pharmacology-based experiments involving the continuous fumigation of tomato seedlings with NO-enriched atmospheres were performed. Whereas NO fumigation had no marked impact on chlorophyll and carotenoid levels in dark- or BL-exposed tomato seedlings (Supplemental Fig. S4), a very clear increase in both these pigments was observed when au seedlings were fumigated continuously with 50, 100, or 150 µL L−1 under RL (Fig. 3A). Such NO-induced greening was shown to be dose dependent, since significant increases in chlorophylls and carotenoids were observed as quickly as 24 h after simultaneous treatment with RL and 150 µL L−1 NO, whereas fumigation with 50 or 100 µL L−1 NO led to significant increases in these pigments only after 48 h of treatment. The levels of pigments in au cotyledons under RL and 150 µL L−1 NO (Fig. 3A) were similar to those observed in RL-treated wild-type cotyledons (Fig. 2). NO fumigation also induced the partial formation of granal thylakoids in RL-treated au seedlings (Fig. 3B). At the end of the treatment, the chloroplast number per cotyledon cell in au seedlings exposed to RL plus NO fumigation (38.2 ± 5.3) was statistically similar to that observed in RL-treated wild-type seedlings (36.1 ± 4.2). No significant influence of NO fumigation on Pchlide content was observed in etiolated au seedlings (Supplemental Fig. S4); therefore, the positive influence of NO fumigation on chlorophyll accumulation apparently does not involve significant changes in chlorophyll precursor levels prior to light exposure.
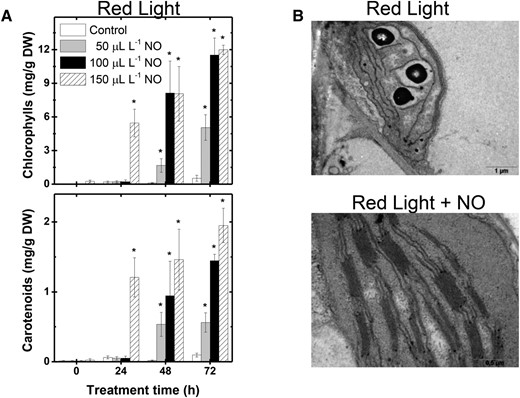
NO fumigation promotes cotyledon greening in tomato phytochromobilin-deficient mutant seedlings. au seedlings dark grown for 120 h were transferred to continuous RL under constant fumigation with NO-free air (control) or different NO concentrations. A, Chlorophylls and carotenoids. B, Plastid structure in au cotyledon cells after 72 h of treatment. Values shown are means ± se. Asterisks indicate statistically significant differences compared with the untreated control at each sampling time (Student’s t test, P < 0.05). DW, Dry weight.
Therefore, NO fumigation partially rescued the severe defect in greening and chloroplast development typically observed in au seedlings grown under monochromatic RL, and such an experimental setup emerged as a particularly interesting model system to study the endogenous mechanisms responsible for converting NO signals into cotyledon greening and chloroplast differentiation.
NO Fumigation Alters mRNA Accumulation of Photomorphogenesis-Related and Plastid Division and Differentiation Genes
To better understand the mechanisms underlying the NO-dependent rescue of the cotyledon greening deficiency phenotype in RL-treated au seedlings, a transcript profile of genes encoding phytochrome, light signaling, and plastid division and differentiation proteins was evaluated. This analysis revealed that, except for SlPHYF, all other genes encoding for phytochrome apoproteins in tomato (SlPHYA, SlPHYB1, SlPHYB2, and SlPHYE; Kendrick et al., 1997) exhibited significantly higher mRNA levels in au than in wild-type seedlings under RL conditions. Interestingly, a progressive reduction in the transcript levels of SlPHYA, SlPHYB1, and SlPHYE, but not in SlPHYB2 and SlPHYF, was observed in RL-treated au seedlings upon NO fumigation (Fig. 4A; Supplemental Fig. S5).
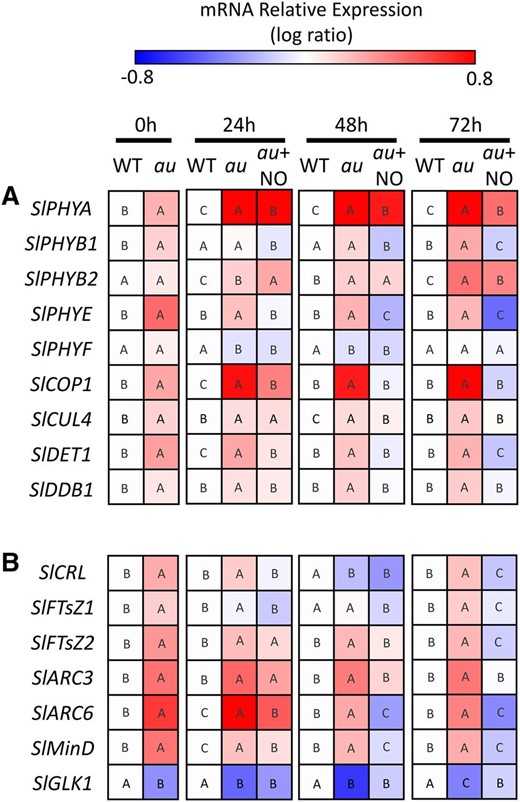
NO fumigation restores wild-type transcript levels of photomorphogenesis-related genes in phytochromobilin-deficient mutant seedlings maintained under RL. Wild-type (WT) and au seedlings dark grown for 120 h were transferred to continuous RL under constant fumigation with NO-free air or 150 μL L−1 NO (au + NO). A, Quantitative reverse transcription-PCR analysis of genes encoding phytochromes and light signaling proteins. B, Quantitative reverse transcription-PCR analysis of genes encoding proteins involved in plastid division and differentiation. Values shown are means ± se. The mean relative expression was calculated from means of two technical replicates of at least three biological replicates and normalized against the samples from wild-type 0-h samples. Different letters indicate statistically significant differences among the genotypes and treatments at each sampling time (P < 0.05) according to a permutation test that lacks assumptions of sample distribution (Pfaffl et al., 2002).
Under continuous RL, mRNA levels of genes encoding the photomorphogenic repressor proteins SlCOP1, SlCUL4, SlDET1, and SlDDB1 were also more abundantly detected in au than in wild-type seedlings, and, quite remarkably, NO fumigation was found to trigger a progressive reduction in the expression of all these genes. After 72 h of NO treatment, the mRNA level of these genes in au were as low as those observed in fully deetiolated wild-type seedlings (Fig. 4A; Supplemental Fig. S5).
A marked influence of NO fumigation was also observed on the mRNA abundance of tomato genes encoding key components of the plastid division machinery (Fig. 5B; Supplemental Fig. S5). The tomato genome possesses at least two paralogs of FILAMENTOUS TEMPERATURE SENSITIVE Z (SlFtsZ1 and SlFtsZ2), which encode tubulin-like GTPases that assemble into a ring-like structure at the plastid division site, thereby representing a central component of the division process (Basak and Møller, 2013). Interacting with FtsZ proteins, a number of structural and regulatory elements, such as ACCUMULATION AND REPLICATION OF CHLOROPLASTS (ARC), CRUMPLED LEAF (CRL), and Min proteins, facilitate the correct placement, assembly, and stabilization of the plastid-dividing FtsZ ring (Basak and Møller, 2013). All six tomato plastid division-associated genes analyzed in this study (SlFTsZ1, SlFTsZ2, SlARC3, SlARC6, SlCRL, and SlMinD) exhibited significantly higher transcript abundance in au than in wild-type seedlings during most of the RL treatment period (Fig. 4B; Supplemental Fig. S6). As observed for the photomorphogenesis-related genes, NO fumigation triggered the reduction in plastid division genes mRNA in au seedlings toward similar, or even lower, amounts than those observed in wild-type seedlings. Finally, it is also worth mentioning that, upon NO fumigation, au seedlings displayed a progressive increase in mRNA amounts of SlGLK1, which encodes a key transcription factor responsible for chloroplast differentiation in tomato vegetative tissues (Fig. 4B; Supplemental Fig. S6). Therefore, NO-induced chloroplast differentiation correlates with the accumulation of SlGLK1 transcripts and a concomitant reduction in mRNA abundance of genes encoding key components of the plastid division machinery.
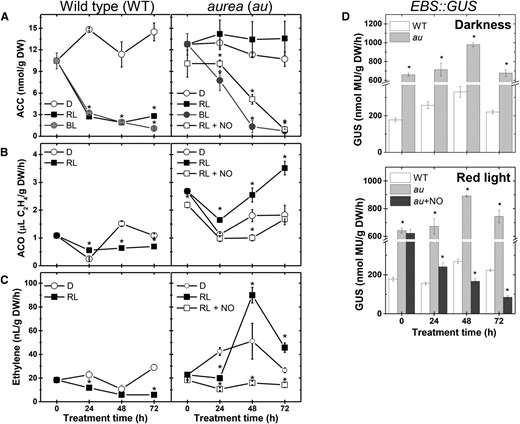
Light and NO down-regulate ethylene metabolism and signaling output during tomato seedling greening. Seedlings dark grown for 120 h were either kept in darkness (D) or transferred to continuous BL, RL, or RL and continuous fumigation with 150 μL L−1 gaseous NO (RL + NO). A, ACC content. B, ACO activity. C, Ethylene emission. D, In vitro GUS activity assayed in EBS::GUS seedlings carrying the synthetic ethylene-responsive promoter EBS fused to the GUS reporter gene. Values shown are means ± se. Asterisks indicate statistically significant differences compared with the dark treatment (in A–C) or with the wild type (WT; in D) at each sampling time (Student’s t test, P < 0.05). au + NO, au seedlings fumigated with 150 μL L−1 NO; DW, Dry weight.
NO and Ethylene Antagonistically Interact during Light-Driven Cotyledon Greening
Given the importance of ethylene during plant responses to light (Rodrigues et al., 2014; Van de Poel et al., 2015; Weller et al., 2015) and its close interplay with NO during diverse plant responses (Manjunatha et al., 2012; Freschi, 2013), we next analyzed whether NO-ethylene interactions regulate tomato seedling greening and chloroplast development.
Whereas the endogenous levels of the ethylene precursor 1-aminocyclopropane-1-carboxylic acid (ACC) remained relatively stable in dark-grown wild-type and au seedlings, a drastic reduction in endogenous ACC in wild-type seedlings was observed soon after exposure to either RL or BL (Fig. 5A). In contrast, BL, but not RL, triggered significant reductions in the ACC content of au seedlings. The activity of ACC oxidase (ACO), a key enzyme in ethylene production, and ethylene emission rates were significantly higher in the au mutant than in wild-type seedlings under either complete darkness or RL conditions (Fig. 5, B and C). Interestingly, fumigation of au seedlings with NO under RL conditions triggered a significant reduction in ACC content, ACO activity, and ethylene emission (Fig. 5), denoting a repressor influence of NO on ethylene biosynthesis during tomato seedling deetiolation.
Additionally, the impact of NO fumigation on ethylene signaling was estimated by determining the activity of the reporter protein GUS expressed under the control of the EBS ethylene-responsive promoter (Stepanova et al., 2007). An enhanced ethylene signaling output was observed in au seedlings maintained under either complete darkness or RL treatment (Fig. 5D). NO fumigation significantly reduced EBS promoter activity in RL-treated au seedlings (Fig. 5D). Interestingly, such a NO-triggered reduction in ethylene signaling output does not seem associated with changes in the tissue sensitivity to this hormone, since simultaneous treatments with exogenous NO and ethylene rendered EBS activity levels similar to those observed in seedlings treated with ethylene alone (Supplemental Fig. S7).
Given that NO down-regulates ethylene production in deetiolating tomato seedlings, we further investigated the ethylene effect on NO production. Treating wild-type seedlings with gaseous ethylene or its precursor ACC resulted in a drastic reduction in endogenous NO release, almost entirely abolishing the RL- or BL-driven up-regulation of NO production (Fig. 6A). Of particular note, both these hormonal treatments significantly reduced cotyledon greening in deetiolating wild-type seedlings (Fig. 7A). Similar analyses conducted in seedlings of the ethylene-insensitive Never ripe (Nr) mutant confirmed the repressor influence of ethylene on NO production in deetiolating tomato seedlings. Compared with the wild type, Nr seedlings released higher amounts of NO (Fig. 6A), which positively correlated with total NR activity (Fig. 6B), NR activation state (Fig. 6C), and pigment levels (Fig. 7) under either RL or BL treatment.
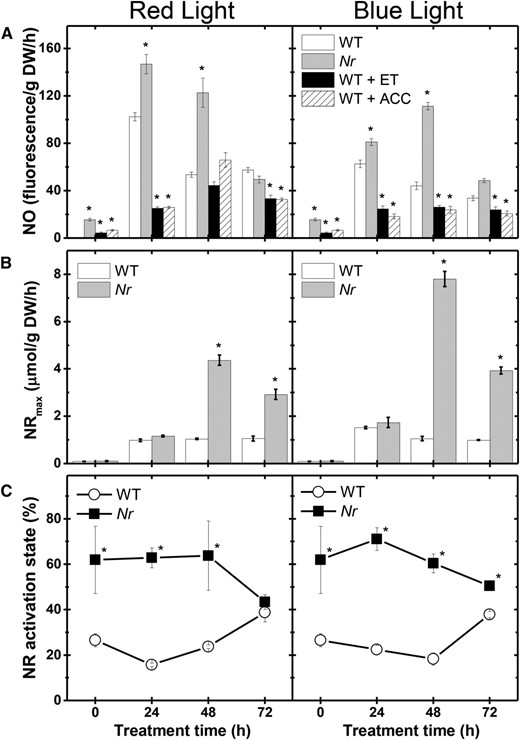
Ethylene negatively influences endogenous NO production in deetiolating tomato seedlings. Seedlings dark grown for 120 h were transferred to continuous RL or BL treatment. Treatment of wild-type (WT) seedlings with 100 μL L−1 gaseous ethylene (ET) or 100 µm ACC was initiated 48 h before starting the light exposure. Ethylene concentration was renewed daily, and ACC was supplemented only once to the growth medium. Seedlings of the ethylene-insensitive Never ripe (Nr) mutant were not subjected to ethylene or ACC treatment. A, Fluorometric quantification of endogenous NO release. B, NR maximum activity (NRmax). C, NR activation state. Values shown are means ± se. Asterisks indicate statistically significant differences compared with the wild type at each sampling time (Student’s t test, P < 0.05). DW, Dry weight.
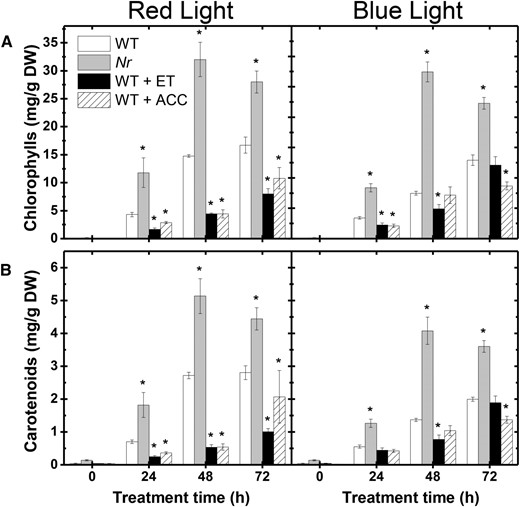
Ethylene inhibits chlorophyll and carotenoid accumulation in deetiolating tomato seedlings. Photosynthetic pigment accumulation is shown in cotyledons of wild-type (WT) seedlings treated with gaseous ethylene (ET) or ACC as well as in the ethylene-insensitive Nr mutant exposed to either RL or BL treatment. Treatment details are as described in Figure 6. A, Chlorophylls. B, Carotenoids. Values shown are means ± se. Asterisks indicate statistically significant differences compared with the wild type at each sampling time (Student’s t test, P < 0.05). DW, Dry weight.
NO Positively Interacts with Auxins during Light-Driven Cotyledon Greening
NO-auxin cross talk has been associated with numerous plant developmental responses (Freschi, 2013; Sanz et al., 2015). In wild-type tomato seedlings, RL-evoked deetiolation was accompanied by a 10-fold increase in the endogenous indole acetic acid (IAA) content (Fig. 8, A and B). In contrast, RL-exposed au seedlings exhibited a very modest increment in IAA endogenous levels, which never exceeded 3 times the IAA levels observed under continuous darkness. Interestingly, NO fumigation of RL-treated au seedlings strongly promoted the accumulation of IAA in cotyledon tissues, which exceeded IAA levels detected in RL-exposed wild-type seedlings (Fig. 8B). The relative transcript amounts of SlARF4, a member of the tomato auxin response factor gene family that acts as a repressor of the auxin response (Sagar et al., 2013), was markedly higher in au than wild-type seedlings under RL conditions (Fig. 8C). SlARF4 mRNA abundance in au was reduced drastically upon NO fumigation, achieving levels even lower than those detected in RL-treated wild-type seedlings (Fig. 8C). Reinforcing a positive interaction between NO, auxins, and light signaling, the auxin-responsive promoter DR5 was strongly activated in au seedlings simultaneously treated with RL and NO fumigation (Fig. 8D). In the absence of NO fumigation, RL exposure failed to stimulate the DR5 promoter in au, which is consistent with the very modest increment in IAA endogenous levels observed under these circumstances (Fig. 8).
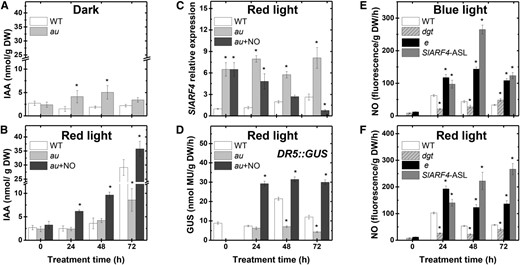
NO and auxin positive interactions during tomato seedling greening. Seedlings dark grown for 120 h were either kept in darkness or transferred to continuous BL, RL, or RL and continuous fumigation with 150 μL L−1 gaseous NO (au + NO). A and B, IAA endogenous levels. C, SlARF4 relative expression. D, In vitro GUS activity assayed in seedlings carrying the synthetic auxin-responsive promoter DR5 fused to the GUS reporter protein (DR5::GUS). E and F, Fluorometric quantification of endogenous NO release. Values shown are means ± se. Asterisks indicate statistically significant differences compared with the wild type (WT) at each sampling time (Student’s t test, P < 0.05). DW, Dry weight; e, entire; SlARF4-ASL, SlARF4-silenced line.
The positive influence of NO on auxin content and signaling output prompted us to evaluate whether alterations in auxin signaling could also affect NO endogenous levels. To test this, endogenous NO was measured in tomato deetiolating mutant or transgenic seedlings with altered auxin responsiveness. The auxin-insensitive tomato mutant diagetropica (dgt) exhibited reduced NO levels when compared with wild-type seedlings (Fig. 8, E and F). In contrast, RL- or BL-exposed seedlings of entire, a mutant overresponsive to auxins due to a single-base deletion in the coding region of the auxin signaling transcriptional repressor AUX/IAA9 (Zhang et al., 2007), or an SlARF4-silenced line (Sagar et al., 2013) exhibited significantly higher endogenous NO release compared with wild-type seedlings (Fig. 8, E and F). Collectively, these data suggest a mutually positive feedback between auxins and NO during tomato seedling deetiolation.
DISCUSSION
The rapid acquisition of functional chloroplasts is of obvious importance for seedlings exposed to light and determines the transition of a purely heterotrophic metabolism to an essentially autotrophic behavior (Waters and Langdale, 2009). Not surprisingly, light is the central environmental cue eliciting and orchestrating the endogenous signaling events responsible for controlling chloroplast differentiation (López-Juez et al., 2008); however, little is known about the cross talk between light and other signaling pathways during this photomorphogenic response. The free radical NO, for instance, has been demonstrated to influence cotyledon and leaf greening when applied exogenously (Beligni and Lamattina, 2000; Zhang et al., 2006; Liu et al., 2013), but the role of endogenous NO in light-evoked chloroplast differentiation and the mechanisms responsible for NO production and action during this photomorphogenic event have remained fairly elusive so far. Here, we provide a line of evidence for the involvement of NO in a complex regulatory network that interconnects light perception, auxins, and ethylene toward the acquisition of photomorphogenic traits in deetiolating tomato seedlings.
NR-Dependent NO Production Is Controlled by Light and Plant Hormones
In plants, NR is arguably the NO-generating route most clearly characterized at both the biochemical and regulatory levels (Lea et al., 2004; Planchet and Kaiser, 2006b; Planchet et al., 2006). In agreement with previous reports (Becker et al., 1992; Goud and Sharma, 1994), tomato NR activity was shown to be phytochrome-dependently induced upon light exposure and repressed by HP1/DDB1 protein (Fig. 2). Interestingly, the light-evoked increase in NO levels always coincided with significant increases in NR activity in both wild-type and mutant tomato seedlings, and all distinct strategies employed to inhibit the induction of this enzyme successfully reduced NO production (Table I). Moreover, a strict temporal correlation between the highest NR and NO levels was observed regardless of the light treatment applied (i.e. RL or BL; Fig. 2F; Supplemental Fig. S4). Therefore, besides being the main NO-generating route involved in numerous plant defense responses (for review, see Mur et al., 2013), our data demonstrated that NR is also the major source of NO during tomato seedling greening. In addition to the reported influence of auxins, cytokinins, and ABA on NR gene expression and enzyme activity (Lu et al., 1992; Yu et al., 1998), our study demonstrated that ethylene negatively affects both NR total activity and activation state (Fig. 6), revealing that NR-dependent NO production in tomato seedlings is under the strict control of both light and hormone signals.
Endogenously Produced or Exogenously Applied NO Promotes Greening
In wild-type seedlings of grasses such as wheat (Beligni and Lamattina, 2000) and barley (Hordeum vulgare; Zhang et al., 2006; Liu et al., 2013; Chen et al., 2014), the positive influence of NO on seedling greening has been demonstrated by the increment in chlorophyll content upon the application of sodium nitroprusside (SNP), an NO donor. However, in wild-type tomato seedlings, no alterations were observed after NO fumigation (Supplemental Fig. S4). This may reflect the significant differences in the pharmacological approach employed in these studies, since SNP decomposition generates not only NO but also other physiologically active compounds such as cyanide (Bethke et al., 2006). Alternatively, this may also indicate that endogenous NO production in light-exposed wild-type tomato seedlings is already sufficient to trigger maximal photosynthetic pigment accumulation, thereby explaining the lack of further increases in chlorophyll and carotenoid levels upon NO fumigation (Supplemental Fig. S4).
However, dose-response greening was observed in au seedlings simultaneously exposed to RL and gaseous NO (Fig. 3), suggesting that NO might complement in some way the partial deficiency in the RL perception characteristic of this phytochromobilin-deficient mutant. Etiolated au seedlings contain a small amount of functional phytochromes, which is estimated to range between 3% and 5% of wild-type levels (Parks et al., 1987), thus conferring some residual RL perception and response to this mutant. Therefore, the stimulatory effect of NO on seedling greening apparently depends on the previous or concomitant activation of at least some phytochrome molecules, which agrees with earlier observations about the importance of concomitant light pulses for the NO-induced partial greening in etiolated wheat seedlings (Beligni and Lamattina, 2000). In addition to these pharmacological data, analyses of mutant tomato seedlings with altered light or hormone perception or signaling revealed that intensified greening always coincided with increased NO production (Figs. 2 and 6). Moreover, a clear temporal coincidence was observed between the endogenous NO generation and the greening process. NO and chlorophyll levels increased concomitantly within the first 24 h of light exposure, whereas the maximal accumulation of photosynthetic pigments was clearly preceded by a transitory peak in endogenous NO levels (Fig. 2F; Supplemental Fig. S4). Therefore, the positive influence of NO on tomato seedling greening is here supported by both genetic and pharmacological evidence.
NO Fumigation Regulates Photomorphogenesis-Related and Plastid Division Genes
Light perception is known to repress Arabidopsis PHYA and PHYB gene expression during seedling deetiolation (Somers and Quail, 1995). Consistent with these findings, the transcript abundance of most tomato genes encoding phytochrome apoproteins was significantly higher in au than in wild-type seedlings under RL, and such differences were consistently reverted upon NO fumigation (Fig. 4). These observations indicate that NO can restore the feedback mechanisms responsible for repressing phytochrome gene expression upon light exposure. Interestingly, it has also been proposed that NO modulates PHYB protein levels in Arabidopsis seedlings under RL (Lozano-Juste and León, 2011). In addition, SNP application was reported to influence PHYA mRNA levels in etiolated wheat seedling leaves maintained under continuous darkness (Liu et al., 2013), suggesting that NO might act as a light-independent upstream regulator of phytochrome gene expression. In our system, the NO-imposed reduction in phytochrome apoprotein mRNA levels took place under RL conditions; therefore, it does not necessarily indicate a direct action of NO as an upstream regulator of phytochrome gene expression, but obviously such a possibility cannot be ruled out. Regardless of acting upstream or downstream of phytochromes, NO fumigation led to a progressive reduction in transcript levels of the photomorphogenic repressor proteins SlCOP1, SlCUL4, SlDET1, and SlDDB1, implying a gradual increase in tissue responsiveness to light stimuli (Jiao et al., 2007), which, in turn, could have facilitated the conversion of the residual RL perception of au seedlings into photomorphogenic responses.
The influence of both light and NO on the plastid division machinery has received little attention in the literature (Basak and Møller, 2013). Our data demonstrated that both of these signals can modulate the mRNA abundance of key plastid division genes (Fig. 4). The impairment in phytochrome-dependent light perception in the au mutant induced the mRNA accumulation of genes involved in plastid division, such as SlFTsZ2, SlARC3, SlARC6, SlCRL, and SlMinD (Fig. 4). This might be either a direct effect of the absence of phytochrome-dependent signaling or the lack of a negative feedback regulation of the plastid division machinery by fully mature chloroplasts (Basak and Møller, 2013). Upon NO fumigation, the mRNA level of plastid division genes decreased at the same time that transcripts of the key chloroplast differentiation gene SlGLK1 accumulated (Fig. 4B; Supplemental Fig. S6). Hence, NO apparently represents one of the endogenous signals responsible for coordinating the balance between plastid biogenesis and differentiation in response to environmental light conditions.
NO Interconnects Light and Plant Hormones during Seedling Greening
The conversion of light stimuli into photomorphogenic responses usually involves the integrated action of both light and hormone signaling cascades (Halliday and Fankhauser, 2003; Lau and Deng, 2010). According to our data, the light-evoked greening in tomato seedlings is at least partially controlled by a mutual antagonism between NO and ethylene metabolisms. Several lines of evidence seem to support this finding. First, regardless of the genotype, the time course of NO and ethylene production was clearly opposite during light-triggered seedling deetiolation. Second, any experimental condition resulting in increased NO production also caused significant reductions in ACC levels, ACO activity, and ethylene emission. Third, NO fumigation drastically reduced the enhanced ethylene biosynthesis and signaling observed in RL-treated au seedlings. Similar to the au mutant, increased ethylene production also has been demonstrated in pea (Pisum sativum) phytochrome-deficient plants (Foo et al., 2006). Treatments with ethylene biosynthesis inhibitor or mutation in the ethylene signaling gene ETHYLENE INSENSITIVE2 rescued many of the phenotype alterations observed in this pea mutant (Foo et al., 2006; Weller et al., 2015). Therefore, light perception via phytochromes is believed to play a critical role in reducing ethylene synthesis to levels compatible with the induction and progression of key photomorphogenic responses (Vangronsveld et al., 1988; Foo et al., 2006; Weller et al., 2015).
The NO-ethylene antagonism detected in tomato seedlings seems to rely mainly on NO-triggered restriction of ethylene biosynthesis rather than on changes in the tissue sensitivity to this hormone. This is evident by the reduction in ACC content and ACO activity followed by the significant decrease in ethylene emission observed in NO-fumigated tomato seedlings (Fig. 5) and is in agreement with pharmacology-based experiments that have suggested NO as a potent inhibitor of ethylene biosynthesis during the onset of fruit ripening and leaf senescence (Leshem et al., 1998; Wills et al., 2000; Manjunatha et al., 2010, 2012). As NO antagonizes ethylene biosynthesis and promotes seedling greening, a negative impact of ethylene on the chlorophyll accumulation might be expected. In fact, deetiolating wild-type seedlings treated with ACC or gaseous ethylene exhibited impaired cotyledon greening and reduced NO levels, while the ethylene-insensitive Nr mutant displayed significantly higher NO, chlorophyll, and carotenoid contents (Figs. 6 and 7).
Over the last 2 years, significant progress has been made in our understanding of the mechanisms responsible for NO sensing and signal transduction in plants (Gibbs et al., 2014; Abbas et al., 2015). The basis for this progress was the identification of NO as a key signal controlling the degradation of group VII ethylene response factors (VII-ERFs) by the N-end rule pathway of targeted proteolysis (Gibbs et al., 2014). Interestingly, NR was shown to represent the main biosynthetic source of NO responsible for modulating the turnover of VII-ERFs during early Arabidopsis development, and the abundance of these transcription factors seems critical for controlling key photomorphogenic events such as hypocotyl elongation (Gibbs et al., 2014), apical hook opening, and cotyledon greening (Abbas et al., 2015). Supporting this view, it has been demonstrated that apical hook opening is repressed significantly in the nia1nia2 NR-deficient double mutant, a phenotype clearly reverted upon NO fumigation or in a combination mutant where VII-ERFs were knocked out in the NR-deficient mutant background (Abbas et al., 2015). Moreover, data obtained by Abbas et al. (2015) also suggest that NO promotes Arabidopsis cotyledon greening by catalyzing the destruction of VII-ERFs, since these transcription factors were shown to repress the expression of key chlorophyll biosynthesis genes. These findings are consistent with our working hypothesis that NR-derived NO promotes tomato cotyledon greening through a close interplay with ethylene signaling and also suggest substantial conservation of the mechanisms responsible for the NO-dependent regulation of early photomorphogenic development between Arabidopsis and tomato.
Multiple cross talk possibilities between light and auxin transduction networks have been described in plants, including a light-driven impact on auxin biosynthesis, catabolism, transport, and tissue responsiveness (for review, see Halliday et al., 2009), but their impacts on seedling greening have remained largely unexplored. Our data point out that, unlike ethylene, NO and auxins interact positively during tomato seedling deetiolation. A marked increase in IAA content was induced in wild-type tomato seedlings upon illumination, which was accompanied by a reduction in the mRNA accumulation of the repressor of auxin response SlARF4 and, consequently, the activation of the auxin-responsive promoter DR5. These changes were observed in RL-treated au seedlings exclusively upon NO fumigation (Fig. 8). Therefore, as observed in some other plant species (Symons and Reid, 2003; Halliday et al., 2009), phytochrome-dependent light perception promotes auxin accumulation and signaling during tomato seedling deetiolation. Moreover, our data also indicate that NO can modulate auxin metabolism and/or transport, and thereby auxin signaling, in tomato cotyledons. This NO-auxin interaction has been reported in other systems and physiological events (Xu et al., 2010; Fernández-Marcos et al., 2011; Terrile et al., 2012). Although the exact mechanisms through which NO and auxin interact in plants still deserve further elucidation, the fact that NO has been shown to promote auxin-dependent gene expression via posttranslational modifications of the Arabidopsis auxin receptor protein TRANSPORT INHIBITOR RESPONSE1 is of particular note (Terrile et al., 2012). This finding implies that changes in auxin tissue sensitivity may be modulated by NO; therefore, the activation of auxin signaling upon NO fumigation in tomato seedlings might reflect not only the increased auxin levels detected under these circumstances (Fig. 8) but also some increment in auxin tissue responsiveness.
Our data exposed a mutually positive feedback regulatory loop during NO-auxin cross talk in tomato cotyledons, because not only did NO promote auxin accumulation and signaling but auxin signaling itself also stimulated endogenous NO production. As evidence of this, seedlings with exaggerated auxin responsiveness, such as the entire mutant or SlARF4-silenced lines, showed increased endogenous NO content, whereas the opposite was observed in the auxin-resistant mutant dgt (Fig. 8). This is consistent with the increased NO production detected upon exogenous auxin application (Pagnussat et al., 2002; Correa-Aragunde et al., 2004; Hu et al., 2005; Lombardo et al., 2006) or in Arabidopsis auxin overproducer mutants (Chen et al., 2010). Finally, considering that auxins have been reported to regulate NR at both the transcriptional and posttranscriptional levels (Vuylsteker et al., 1997) and that the NR-deficient Arabidopsis mutant fails to generate NO in response to exogenous auxin (Kolbert et al., 2008), it seems tempting to speculate that this enzyme is responsible for the auxin-triggered changes in NO production observed in this study.
Although not explored in this study, another NO-hormone interaction critically important for seedling photomorphogenesis consists of the intricate interplay between NO and gibberellins (GAs) (Lozano-Juste and León, 2011; Freschi, 2013). A mounting body of evidence has indicated that a mutual NO-GA antagonism regulates hypocotyl elongation in Arabidopsis mainly due to the positive influence of NO on the accumulation of DELLA proteins (Lozano-Juste and León, 2011). In agreement, a dramatic reduction in GA1 levels was reported in pea seedlings soon after transfer from darkness to light (Symons and Reid, 2003; Weller et al., 2009), which would imply increased DELLA levels upon light exposure. Interestingly, DELLAs are considered to act as promoters of greening (Cheminant et al., 2011); therefore, the NO-GA antagonism already described during the regulation of hypocotyl elongation (Lozano-Juste and León, 2011) may also be involved in the light-evoked cotyledon-greening process. Further studies focusing on NO-GA interactions during cotyledon greening are clearly required to substantiate this possibility.
In conclusion, this study brings evidence of a close interaction between NO, ethylene, and auxins during the light-induced etioplast-to-chloroplast conversion in deetiolating tomato seedlings. During this process, the light-induced production of NO via NR activity apparently antagonizes ethylene production and intensifies auxin accumulation in cotyledon tissues. As a consequence, negative and positive feedback regulatory loops apparently orchestrate ethylene-NO and auxin-NO interactions, respectively (Fig. 9). Therefore, NO seems to represent an important element interconnecting phytochrome-dependent light perception, auxins, and ethylene toward the acquisition of fully mature chloroplasts in deetiolating seedlings.
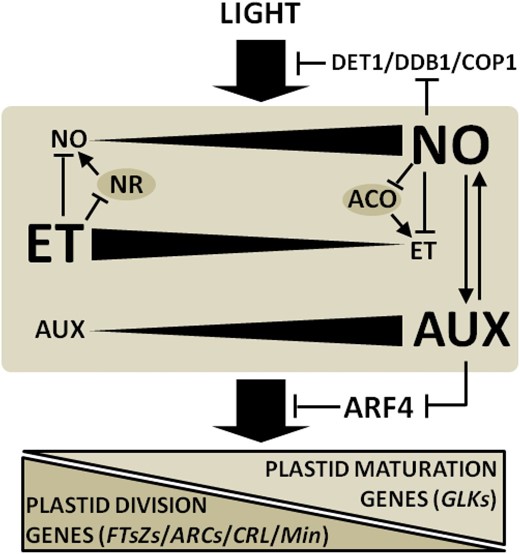
Diagram illustrating light, NO, ethylene (ET), and auxin (AUX) cross talk during the acquisition of photomorphogenic traits in tomato seedlings. During light-evoked cotyledon greening and chloroplast differentiation, negative and positive feedback regulatory loops orchestrate NO-ET and NO-AUX interactions, respectively. The mutual NO-ET antagonism occurs at biosynthetic levels involving the negative regulation of NR and ACO by ET and NO, respectively. By repressing negative regulators of light signal transduction such as DET1, DDB1, and COP1 and modulating the AUX-ET balance, NO promotes the accumulation of the chloroplast differentiation transcription factor GLK and inhibits key plastid division genes such as FTsZs, ARCs, CRL, and Min. Biosynthetic enzymes are represented with gray ovals. Arrows at the ends of lines indicate stimulatory effects, whereas bars indicate inhibitory effects.
MATERIALS AND METHODS
Plant Material and Growth Conditions
Wild-type tomato (Solanum lycopersicum ‘Micro-Tom’) and the near-isogenic lines harboring the mutations au, hp1, entire, dgt, and Nr were obtained by Carvalho et al. (2011). The wild type, near-isogenic lines, and transgenic cv Micro-Tom carrying the synthetic auxin-responsive (DR5) and ethylene-responsive (EBS) promoters fused to the reporter gene uid (encoding a GUS) were obtained from the tomato mutant collection maintained at the Escola Superior de Agricultura Luiz de Queiroz, Universidade de São Paulo (http://www.esalq.usp.br/tomato/). Seeds of the cv Micro-Tom SlARF4-silenced line (SlARF4-ASL) were obtained from the University of Toulouse (Sagar et al., 2013). Additionally, crosses and phenotypical screening were performed to generate the double mutants au-DR5::GUS and au-EBS::GUS.
Growth Conditions and Treatments
Seeds were surface sterilized with 0.5% (v/v) sodium hypochlorite and sown directly in magenta vessels containing sterile medium composed of one-half-strength MS salts and 2% (w/v) Phytagel. After 120 h of pregermination in absolute darkness, seedlings were transferred to continuous RL or BL or maintained under absolute darkness. RL and BL were supplied by an array of SMD5050 Samsung light-emitting diodes mounted in a temperature-controlled growth chamber maintained at 25°C ± 1°C and installed inside a darkroom. Both BL and RL were delivered at approximately 50 µmol m−2 s−1, with peak output at 470 and 625 nm, respectively, as defined by the manufacturer. In all cases, tissue was harvested either under the specific light conditions used for seedling growth or under dim green light, as appropriate.
For the treatment of seedlings with inhibitors of NR activity, seeds were grown in the same manner described above except that the MS medium was modified, as appropriate. In the nitrogen-free MS medium, NH4NO3 was omitted and KNO3 was replaced by 0.35 g L−1 KCl and 0.4 g L−1 K2SO4. Modified MS medium containing ammonium or Gln as the exclusive source of nitrogen was essentially the same formulation as the nitrogen-free MS medium with the addition of 1.61 g L−1 NH4Cl or 2.19 g L−1 Gln, respectively. Therefore, when present in the medium, nitrogen was always available at a final concentration of 30 mm. The medium containing Gln was sterilized by ultrafiltration, whereas the others were autoclaved.
Hormonal and NO treatments were initiated 72 h before starting the light treatments and were maintained thereafter. ACC was dissolved in water, sterilized by ultrafiltration, and applied directly to the growth medium containing the seedlings. Treatments with gaseous ethylene (100 µL L−1) and NO (50, 100, or 150 µL L−1) were conducted in sealed magenta boxes (total airspace volume of 360 mL). Ethylene application was renewed on a daily basis. NO fumigation was performed by mixing a commercial standard mixture (10 mL L−1 NO in nitrogen) with NO-free breathing air at appropriate flow rate ratios. NO gas mixtures were bubbled through a flask containing 500 mm KOH to eliminate any nitrous acid traces and humidify the gas mixture. A continuous flow (100 mL min−1) was maintained throughout the experiments, and final NO concentration was routinely checked by injecting 1-mL aliquots of each magenta box’s internal atmosphere into a chemiluminescence detector (CLD 88ep; Eco-Physics), as described by Freschi et al. (2010).
Chlorophyll and Carotenoid Quantification
For chlorophyll and carotenoid extraction, samples of cotyledons were weighed (typically 20 mg fresh weight), immersed in a 100× excess volume of N,N-dimethylformamide, and incubated for 48 h at 25°C in absolute darkness. The supernatant absorbance was recorded at 480, 647, and 664 nm, and the total chlorophyll and carotenoid contents were estimated using the equations published by Porra et al. (1989) and Wellburn (1994).
NO Measurements
For fluorometric NO determination, the cell-impermeant fluorophore DAR-4M was used. Cotyledons were gently fragmented into small pieces (typically 5 × 5 mm), immediately weighed (approximately 100 mg fresh weight), and incubated with 1 mL of 50 mm phosphate buffer (pH 7.2) containing 37.5 µm DAR-4M for 30 min at 25°C in absolute darkness on a rotary shaker (200 rpm). The supernatant fluorescence was measured using a spectrofluorometer (LS55; Perkin Elmer) with 560-nm excitation and 575-nm emission wavelengths (5-nm bandwidth). Fluorescence was measured at the same instrument settings in all experiments and was expressed as arbitrary fluorescence units per gram dry weight per hour.
NR Activity Assay and Activation State
NR maximum activity and activation state were assayed according to Tucker et al. (2004) with some modifications. Briefly, samples were ground in liquid nitrogen and subsequently homogenized in extraction buffer (approximately 300 mg tissue fresh weight mL−1) composed of 100 mm HEPES-KOH (pH 7.6), 10 µm FAD, 10 µm Na2MoO4, 1 mm EDTA, 0.5% (w/v) polyvinylpyrrolidone, 5 mm dithiothreitol (DTT), and 10 µm leupeptin. After centrifugation (13,000g, 10 min, and 4°C), 100 µL of the supernatant was added to 900 µL of reaction medium composed of 100 mm HEPES-KOH (pH 7.6), 10 µm FAD, 5 mm DTT, 20 mm KNO3, 200 µm NADH, and 10 mm EDTA (for NR maximum activity) or 15 mm MgCl2 (for actual NR activity). The reactions were incubated for 5 min at 30°C and then stopped by adding 100 µL of 500 mm zinc acetate. The unreacted NADH was oxidized by incubation with 100 µm phenazine methosulfate for 20 min, and the nitrite produced by the reactions was quantified by standard colorimetric reaction (Man et al., 1999). For the determination of NR maximum activity, the same extract was preincubated in ice for 12 min in the presence of 20 mm AMP, 15 mm EDTA, and 10 mm KH2PO4. In all cases, blanks were made by adding zinc acetate to the reaction medium prior to the addition of the protein extract. NR activation state is given as the ratio of maximum NR activity (measured after preincubation of extracts with excess EDTA plus AMP) and the values of NR activity (measured in the presence of excess Mg2+; Man et al., 1999). NR activities obtained in the presence of excess Mg2+ are believed to reflect NR activity in situ, whereas maximum NR activity indicates the total pool of NR protein available in the tissues (Man et al., 1999).
Ethylene Measurements
Ethylene emission was analyzed as described by Freschi et al. (2010). Briefly, intact tomato seedlings (typically 100 individuals) growing inside sealed magenta boxes were flushed with ethylene-free air (1 L min−1) for 5 min and incubated for 24 h under specific experimental conditions, as appropriate. After incubation, 1-mL gas samples were withdrawn with a gas-tight syringe and injected into a Trace Ultra gas chromatograph (Thermo Electron) fitted with a flame ionization detector and an RT-Alumina Plot column (Restek). Nitrogen was used as the carrier gas at a flow rate of 3 mL min−1, and commercial standard mixtures of ethylene were used for the calibration curves. Column, injector, and detector temperatures were 40°C, 250°C, and 250°C, respectively.
IAA and ACC Measurements
Endogenous IAA and ACC levels were determined simultaneously by gas chromatography-tandem mass spectrometry-selective ion monitoring. Approximately 200 mg fresh weight of frozen cotyledon samples was ground in liquid nitrogen in the presence of 20 mg of polyvinylpyrrolidone and subsequently homogenized in 1 mL of extraction solution composed of 10 mm ascorbic acid, 10 mm EDTA, and 10 mm DTT. Exactly 1 μg of [13C6]IAA (Cambridge Isotopes) and 2 μg of [2H4]ACC (Sigma-Aldrich) were added to each sample as internal standards. After centrifugation (25,000g, 20 min, and 4°C), the supernatant was purified via solid-phase extraction (Supelclean LC-NH2 column; Supelco), as described by Purgatto et al. (2002). Purified samples were evaporated, resuspended in 50 μL of pyridine, followed by a 60-min derivatization at 92°C using 50 μL of N-tert-butyldimethylsilyl-N-methyltrifluoroacetamide. The analysis was performed on a gas chromatograph coupled to a mass spectrometer (model GCMS-QP2010 SE; Shimadzu) in selective ion monitoring mode. The chromatograph was equipped with a fused-silica capillary column (30 m, 0.25 mm i.d., and 0.25-μm-thick internal film) DB-5 MS stationary phase using helium as the carrier gas at a flow rate of 4.5 mL min–1 in the following program: 2 min at 100°C, followed by a ramp of 10°C min–1 to 140°C, 25°C min–1 to 160°C, 35°C min–1 to 250°C, 20°C min–1 to 270°C, and 30°C min–1 to 300°C. The injector temperature was 250°C, and the following mass spectrometry operating parameters were used: ionization voltage, 70 eV (electron-impact ionization); ion source temperature, 230°C; and interface temperature, 260°C. Ions with a mass ratio/charge of 244, 202, and 130 (corresponding to endogenous IAA), 250, 208, and 136 (corresponding to [13C6]IAA), 272, 244, and 188 (corresponding to endogenous ACC), and 276, 248, and 192 (corresponding to [2H4]ACC) were monitored. Endogenous concentrations were calculated based on extracted chromatograms at mass ratio/charge 244 and 250 for IAA and 272 and 276 for ACC.
Quantitative GUS Activity Assay
GUS activity was assayed according to Jefferson et al. (1987) with some modifications. Briefly, cotyledon samples were ground in liquid nitrogen and subsequently homogenized in 4-methylumbelliferyl-β-d-glucuronide extraction buffer (approximately 150 mg tissue fresh weight mL–1) composed of 50 mm HEPES-KOH (pH 7), 5 mm DTT, and 0.5% (w/v) polyvinylpyrrolidone. After centrifugation (13,000g, 20 min, and 4°C), 200-µL aliquots of the supernatant were mixed with 200 µL of GUS assay buffer composed of 50 mm HEPES-KOH (pH 7), 5 mm DTT, 10 mm EDTA, and 2 mm 4-methylumbelliferyl-β-d-glucuronide and incubated at 37°C for 30 min. Subsequently, aliquots of 100 µL were taken from each tube, the reactions were stopped with 2.9 mL of 0.2 m Na2CO3 (pH 9.5), and fluorescence was determined using a spectrofluorometer (LS55; Perkin Elmer) with 365-nm excitation and 460-nm emission wavelengths (5-nm bandwidth). Fluorescence was measured at the same instrument settings in all experiments.
Measurement of ACO Activity
The extraction and activity assay of ACO was carried out according to Vriezen et al. (1999) with some modifications. Briefly, samples were ground in liquid nitrogen and subsequently homogenized in extraction buffer (approximately 500 mg tissue fresh weight mL–1) composed of 300 mm Tris-HCl (pH 8), 50 mg mL–1 insoluble polyvinylpyrrolidone, 10% (v/v) glycerol, and 30 mm sodium ascorbate. After centrifugation (20,000g, 20 min, and 4°C), 200 µL of the supernatant was added to 1.7 mL of reaction medium composed of 100 mm Tris-HCl (pH 8), 10% (v/v) glycerol, 30 mm sodium ascorbate, 100 µm FeSO4, 50 mm NaHCO3, 5 mm DTT, and 2 mm ACC. ACO activity was determined by measuring the ability of the extract to convert exogenous ACC to ethylene after incubation at 30°C for 20 min. The ethylene levels were measured by gas chromatography-flame ionization detection, as described above.
Transmission Electron Microscopy
Cotyledons were cut into small pieces (1 × 1 mm) and fixed with 2.5% (w/v) glutaraldehyde in 0.1 m sodium phosphate buffer (pH 7.2), postfixed in 1% (w/v) osmium tetroxide in 0.1 m sodium phosphate buffer (pH 7.2), dehydrated in a graded acetone series, and embedded in Spurr’s resin. Semithin sections were stained with 1% (w/v) Toluidine Blue, and the ultrathin sections were counterstained with uranyl acetate (Watson, 1958) and lead citrate (Reynolds, 1963) and analyzed with a Zeiss EM 900 transmission electron microscope. The electron micrographs illustrate the relevant results obtained.
Quantitative PCR
RNA extraction and quantitative PCR (qPCR) were performed as described by Lira et al. (2014) with some modifications. Total RNA was extracted from 200 mg fresh weight of powdered cotyledon material using the Plant RNeasy Kit (Qiagen) and subsequently treated with the TURBO DNA-free Kit (Ambion). The complementary DNA was synthesized using the SuperScript III One-Step RT-PCR System (Invitrogen), as recommended by the manufacturer. mRNA levels were quantified using a 7500 Real-Time PCR system (Applied Biosystems) and SYBR Select Master Mix (Applied Biosystems). Data were analyzed as described by Lira et al. (2014). All reactions were performed with two technical replicates and four biological replicates. Expression values were normalized against the geometric mean of two reference genes, SlUBIQ and SlGAGA. All primers used are shown in Supplemental Table S1.
Statistical Analysis
Statistical analyses were performed using the Jump statistical software package (14th edition) and Microsoft Excel 2010. Test results with P ≤ 0.05 were considered statistically significant.
Sequence data from this article can be found in the GenBank/EMBL data libraries under accession numbers.
Supplemental Data
The following supplemental materials are available.
Supplemental Figure S1. Plastid structure in wild-type and mutant tomato seedlings exposed to RL, BL, and white light.
Supplemental Figure S2. Detailed time course of greening, NO levels, and NR activity in wild-type seedlings.
Supplemental Figure S3. Influence of pharmacological treatments with NOS-like inhibitor.
Supplemental Figure S4. NO fumigation effects under continuous darkness or BL.
Supplemental Figure S5. qPCR data of genes encoding phytochromes and light signaling proteins.
Supplemental Figure S6. qPCR data of genes encoding plastid division and differentiation proteins.
Supplemental Figure S7. Tissue sensitivity to ethylene in NO-treated seedlings.
Supplemental Table S1. Primers used for qPCR.
ACKNOWLEDGMENTS
We thank Mondher Bouzayen (University of Toulouse) for providing seeds of the cv Micro-Tom SlARF4 antisense line.
Glossary
- Pchlide
protochlorophyllide
- PLB
prolamellar body
- ABA
abscisic acid
- NO
nitric oxide
- RL
red light
- BL
blue light
- DAR-4M
diaminorhodamine-4M
- MS
Murashige and Skoog
- ACC
1-aminocyclopropane-1-carboxylic acid
- IAA
indole acetic acid
- SNP
sodium nitroprusside
- VII-ERFs
group VII ethylene response factors
- DTT
dithiothreitol
- qPCR
quantitative PCR
LITERATURE CITED
Author notes
This work was supported by the Conselho Nacional de Desenvolvimento Científico e Tecnológico (grant nos. 472669/2010–9 and 442045/2014–0 to L.F.) and the São Paulo Research Foundation (grant no. 2013/18056–2 to L.F.).
Address correspondence to [email protected].
The author responsible for distribution of materials integral to the findings presented in this article in accordance with the policy described in the Instructions for Authors (www.plantphysiol.org) is: Luciano Freschi ([email protected]).
N.K.G.M. conducted most of the experiments; R.E.B., B.S.L., and M.R. participated in the gene expression experiments; P.M.R.O. performed the hormonal analysis; D.D. participated in the ultrastructure analysis; R.Z. and D.L.O.D. conducted part of the experiments; L.E.P.P. produced the transgenic lines and mutants and participated in the interpretation of results; L.F. conceived the project and supervised the experiments; N.K.G.M. and L.F. wrote the article with contributions from the other authors.
Articles can be viewed without a subscription.