-
PDF
- Split View
-
Views
-
Cite
Cite
Michael E. Stokes, Abhishek Chattopadhyay, Olivia Wilkins, Eiji Nambara, Malcolm M. Campbell, Interplay between Sucrose and Folate Modulates Auxin Signaling in Arabidopsis , Plant Physiology, Volume 162, Issue 3, July 2013, Pages 1552–1565, https://doi.org/10.1104/pp.113.215095
- Share Icon Share
Abstract
As sessile organisms growing in an ever-changing environment, plants must integrate multiple regulatory inputs to promote the appropriate developmental responses. One such nutritional signal is cellular sugar levels, which rise and fall throughout the day and affect a variety of developmental processes. To uncover signaling pathways that modulate sugar perception, compounds from the Library of Active Compounds in Arabidopsis were screened for the ability to perturb developmental responses to sucrose (Suc) in Arabidopsis (Arabidopsis thaliana) seedlings. This screen found that sulfonamides, which inhibit folate biosynthesis in plants, restrict hypocotyl elongation in a sugar-dependent fashion. Transcriptome analysis identified a small set of transcripts that respond to the interaction between sulfonamide and Suc, including a number of transcripts encoding Auxin/Indole-3-Acetic Acids, negative regulators of auxin signal transduction. Chemical inhibition of auxin transport or genetic disruption of auxin signaling relieved this interaction, suggesting that responses to these two nutritional stimuli are mediated by auxin. Reporter systems used to track auxin signaling and distribution showed enhanced activity in the vascular region of the hypocotyl in response to cotreatment of Suc and sulfonamide, yet no change in auxin abundance was observed. Taken together, these findings suggest that the interplay between Suc and folates acts to fine-tune auxin sensitivity and influences auxin distribution during seedling development.
Sugars play a multifaceted role in plant biology, acting as structural components, sources of energy, and signaling molecules (Rolland et al., 2006). The effect of sugars on development can be seen across all stages of the plant life cycle, from germination and establishment (Zhou et al., 1998; Rognoni et al., 2007) through vegetative growth and floral transition (Ohto et al., 2001; Corbesier et al., 2002) until senescence (Wingler and Roitsch, 2008). At the earliest stages of plant growth, the perception of sugars influences a number of seedling traits, including primary root growth (Freixes et al., 2002; Yazdanbakhsh et al., 2011) and hypocotyl elongation (Stevenson and Harrington, 2009). As carbohydrate availability fluctuates on a diurnal cycle (Bläsing et al., 2005), other metabolic and environmental stimuli are in flux as well (Gibon et al., 2006), and it is therefore necessary for sugar signals to be integrated with other signaling pathways to promote the appropriate developmental responses.
Studies into the effect of sugars on plant growth have revealed a connection between carbohydrates, nutrient status, and primary metabolism (Gibon et al., 2006; Usadel et al., 2008). Transcriptome analysis has uncovered many overlapping and synergistic responses between treatment with sugars and with nutrients, such as nitrate and phosphate, suggesting both similar response pathways as well as interactions between signaling components (Price et al., 2004; Müller et al., 2007). Sugar availability has also been linked to the transcriptional regulation of many components of primary and secondary metabolism, including the oxidative pentose-phosphate pathway (Lejay et al., 2008), the anthocyanin biosynthetic pathway (Solfanelli et al., 2006), and starch metabolism (Sokolov et al., 1998), among others. It is apparent that sugars impact many facets of plant metabolism and that some of these changes may be regulated at the transcriptional level.
Many plant responses to sugar are mediated by the interaction between metabolic and hormone-signaling pathways (Gazzarrini and McCourt, 2001; Rolland et al., 2006; Rook et al., 2006b). Forward genetic screens have uncovered sugar-resistant mutants to be novel alleles of known hormone mutants, highlighting the extensive crosstalk between sugar- and hormone-signaling pathways (Zhou et al., 1998; Arenas-Huertero et al., 2000; Laby et al., 2000; Gibson et al., 2001). Cross talk between hormone pathways is a well-explored phenomenon, and there are many examples of hormone pathways that modify, impinge upon, or promote the signaling of other hormones (Jaillais and Chory, 2010; Depuydt and Hardtke, 2011). In instances where points of cross talk do exist, interplay between metabolite and hormone signaling may offer a point through which primary metabolism can direct plant growth and development (Falkenberg et al., 2008).
Given the level of redundancy that exists within metabolic and hormone-signaling pathways, it is likely that there are pathways that interact with and modify sugar perception that have not been elucidated thus far by classical genetic methods (Smith and Stitt, 2007). As an alternate approach to forward genetic screening, chemical genetics can be used to overcome issues of functional redundancy (McCourt and Desveaux, 2010; Tóth and van der Hoorn, 2010). For example, a chemical could bind and inhibit the common active site of a group of redundant proteins (De Rybel et al., 2009) or activate a single member of a family of closely related receptors (Park et al., 2009). In either scenario, chemical genetics may be used to illuminate biological function that is masked by functional redundancy and likely unattainable through classical forward genetic approaches.
In addition to overcoming functional redundancy, interconnectivity between biological processes can be inferred by screening for compounds that act synergistically on a phenotypic output (Lehár et al., 2007; Yeh and Kishony, 2007; Owens et al., 2010). Termed “combination chemical genetics,” this approach entails screening chemical libraries in the presence of a compound of interest to uncover secondary chemicals that enhance or negate its effect (Lehar et al., 2008). Hits from these screens can act antagonistically, additively, or synergistically, based on the manner in which their targets interact biologically (Lehár et al., 2007, 2008). When chemicals act synergistically on a given phenotype, it is believed that the chemicals affect interacting molecular pathways (Yeh et al., 2006; Lehár et al., 2007).
In this study, a combination chemical genetic screen was used to identify pathways that modify plant responses to sugar. This screen uncovered sulfonamides, known to inhibit tetrahydrofolate (THF) biosynthesis in plants (Prabhu et al., 1998), to act synergistically with Suc to inhibit etiolated hypocotyl elongation. The pool of folates produced by the THF biosynthetic pathway are required for a variety of metabolic processes and provides the cofactors for the methyl transfer reactions that constitute one-carbon (C1) metabolism in plants (Hanson and Roje, 2001). Here, the folate inhibitor sulfamethoxazole (SMX) was used to explore the role of folates in mediating developmental responses to Suc.
RESULTS
Sulfonamides Alter Seedling Responses to Suc
Chemicals were screened from the Library of Active Compounds in Arabidopsis (LATCA) and were selected as positive hits based on the ability to act synergistically with Suc to inhibit hypocotyl elongation in dark-grown seedlings (Fig. 1A). Of the 2,100 compounds screened, 33 positive hits were reconfirmed in a secondary screen (Supplemental Table S1). Though positive hits were derived from a variety of structural classes and functions, the largest group of successful hits belonged to the sulfonamide family of compounds, which were chosen for further study (Fig. 1B).
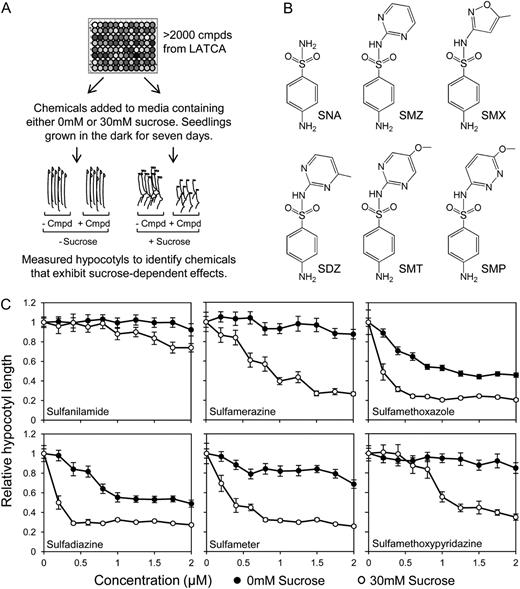
Suc conferred hypersensitivity to sulfonamides. A, Chemicals selected as positive hits based on the ability to act synergistically with Suc to inhibit hypocotyl elongation in dark-grown seedlings. B, Structures of five sulfonamides identified in the chemical screen plus the core structure sulfanilamide. C, Dose response curves of five sulfonamides identified in original chemical screen and the core structure, sulfanilamide, indicate that the effect of sulfonamide on hypocotyl elongation is augmented by the presence of Suc. Data represent the mean ± se of three biological replicates from a representative experiment; each data point represents at least 36 individuals. SNA, Sulfanilamide; SMZ, sulfamerazine; SDZ, sulfadiazine; SMT, sulfameter; SMP, sulfamethoxypyridazine.
Dose response curves for five sulfonamides showed a saturable, dose-dependent inhibition of hypocotyl elongation that was enhanced by the presence of Suc (Fig. 1C; Supplemental Fig. S1). The sulfonamides identified in the chemical screen are derivatives of sulfanilamide (Fig. 1B; Prabhu et al., 1997, 1998; Brackett et al., 2004), a compound not found in the LATCA. These sulfonamides are characterized by the five- or six-membered nitrogen-containing ring attached at the nitrogen of the sulfonamide functional group (Brackett et al., 2004). When tested, sulfanilamide did not exert as strong of an effect compared with other sulfonamides and required a higher concentration to inhibit hypocotyl elongation (Fig. 1C; Supplemental Fig. S1). This indicates that the substituent group plays a role in determining the efficacy of the sulfonamide. Because SMX exerted the strongest effect, it was chosen for use in subsequent experiments. This enabled experiments to be carried out at lower working concentrations of the chemical, which helped reduce any off-target effects of the sulfonamide.
SMX Impinges on Suc-Mediated Development through Inhibition of Dihydropteroate Synthase
Sulfonamides are a class of compounds known to inhibit the THF biosynthetic pathway by competing with para-aminobenzoic acid (pABA) for the active site of dihydropteroate synthase (DHPS; Fig. 2A; Prabhu et al., 1997, 1998; Storozhenko et al., 2007). DHPS is an enzyme that combines dihydropterin and pABA to form dihydropteroate, which is then polyglutamylated and reduced to form THF (Hanson and Gregory, 2002). THF exists bound to C1 units at various oxidation states, collectively termed folates, and acts as a cofactor in a variety of single-carbon transfer reactions (C1 metabolism; Hanson and Roje, 2001; Hanson and Gregory, 2002). Though sulfonamides have previously been shown to inhibit DHPS (Prabhu et al., 1997), it is possible that the compounds affected other processes as well or that the sulfonamides were metabolized to new compounds with alternate modes of action (Zhao et al., 2007). It was therefore necessary to confirm that the phenotypes observed were related to the inhibition of DHPS, rather than secondary effects of the compound. To this end, chemical complementation was performed by supplementing the growth medium with either pABA or folic acid (FA). The addition of the DHPS substrate (pABA) or product (FA) negated the inhibitory effect of SMX and rescued hypocotyl elongation (Fig. 2, B and C). This was consistent with the fact that FA supplements the folate pool downstream of DHPS and therefore compensates for the effect of the compound. In the case of pABA, the effect of SMX was likely reversed by outcompeting SMX at the DHPS active site.
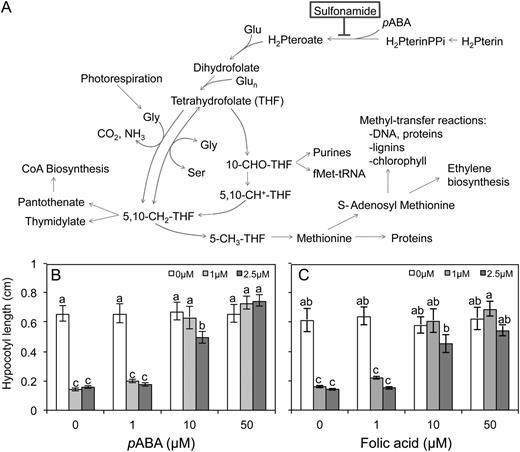
Overview of C1 metabolism in plants. A, SMX inhibits DHPS, an early step in THF biosynthesis. Supplementing media with either FA (B) or pABA (C) rescues hypocotyl elongation. Dark-grown seedlings were raised in the presence of 30 mm Suc and SMX, as indicated. Data represent the mean ± se of three biological replicates from a representative experiment; each data point represents at least 36 individuals. Letters indicate values with statistically significant differences (P < 0.05; ANOVA followed by Tukey’s b test).
To verify that the inhibition of DHPS was modifying sensitivity to Suc, the effect of SMX was tested on plants with elevated DHPS activity. It was hypothesized that if DHPS is the true target of SMX, then elevating DHPS abundance would confer resistance to the compound. The Arabidopsis (Arabidopsis thaliana) genome contains two DHPS isoforms, one mitochondrial (At4g30000) and one cytosolic (At1g69190; Storozhenko et al., 2007); each was constitutively expressed under the control of the Cauliflower mosaic virus 35S promoter in planta. Activity of the construct was confirmed by measuring the expression of DHPS in three independently transformed lines using quantitative PCR (Supplemental Fig. S2A). Although constitutive expression of either isoform attenuated the inhibitory effect of SMX at a lower concentration, higher concentrations of sulfonamide still inhibited hypocotyl elongation at a level similar to the wild type (Fig. 3; Supplemental Fig. S2B). The need for higher concentrations of SMX to produce an effect equivalent to the wild type in plants overexpressing DHPS was consistent with DHPS being the primary target for the sulfonamide. As a means of explaining the heightened sensitivity to SMX conferred by Suc, it was hypothesized that Suc negatively regulates DHPS and that when growing in Suc, the seedlings simply have lower DHPS abundance. To test this, the expression of both isoforms was measured in response to Suc using quantitative PCR, and it was found that Suc did not affect transcript abundance of either DHPS isoform (Supplemental Fig. S2C).
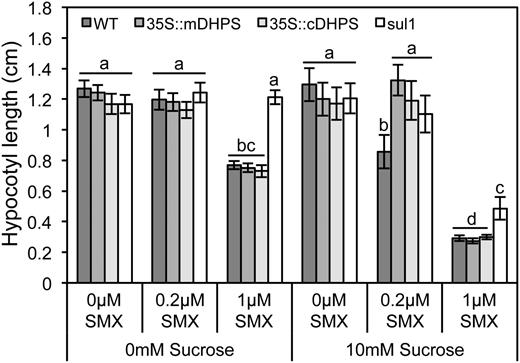
Enhanced DHPS activity attenuated the interaction between Suc and SMX. Hypocotyls were measured from 7-d-old dark-grown seedlings raised in the presence of Suc and SMX, as indicated. Plants constitutively expressing either the mitochondrial (35S::mDHPS) or the cytosolic (35S::cDHPS) isoform exhibited mild resistance to the synergistic effect of Suc and SMX, compared with the wild type (WT). Plants expressing the bacterial SMX-resistant DHPS (sul1) exhibited greater resistance than plants expressing wild-type plant alleles. Data represent the mean ± se of three biological replicates from a representative experiment; each data point represents at least 36 individuals. Letters indicate values with statistically significant differences (P < 0.05; ANOVA followed by Tukey’s b test).
As plants that constitutively expressed the wild-type DHPS alleles maintained sensitivity to higher concentration of SMX, an additional test was undertaken to confirm that the binding of DHPS was necessary to confer sensitivity to Suc. Plants expressing a microbial sulfonamide-resistant DHPS were used to corroborate the mild resistance conferred by overexpressing the wild-type plant DHPS. Consistent with the hypothesis that inhibition of DHPS by the sulfonamide caused hypersensitivity to Suc, plants harboring the sulfonamide-resistant1 (sul1) marker were highly resistant to SMX (Fig. 3; Supplemental Fig. S2B). These plants exhibited a markedly higher resistance to the sulfonamide, compared with the plant harboring wild-type DHPS alleles. Taken together, the chemical complementation assays and the resistance conferred by enhanced DHPS activity supported the notion that the inhibition of DHPS caused heightened sensitivity to Suc.
Changes in Transcript Abundance of Specific Auxin Signal Transduction Components Occurs in Response to the Combined Action of SMX and Suc
The transcriptome-level responses of seedlings to Suc and SMX were examined by microarray analysis. Concentrations of Suc and SMX were identified that had no effect on hypocotyl elongation when administered separately (10 mm Suc and 0.2 µm SMX) but inhibited elongation when they were present together in the growth media (Fig. 4A). When the relationship between these treatments was tested using a two-way ANOVA, it was determined that the effect Suc and SMX exhibited an interaction (P < 0.01). These treatments were then used as the basis for a microarray experiment aimed at uncovering changes in the transcriptome that may underpin this interaction.
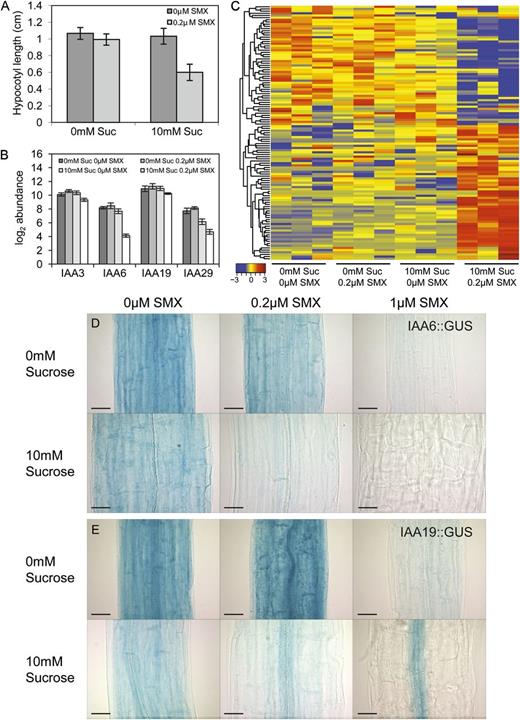
Synergy between Suc and SMX restricts the expression of a set of Aux/IAAs. A, Concentrations of Suc and SMX were determined, which when administered independently, induced no effect on hypocotyl elongation, yet inhibited it when administered together (10 mm Suc and 0.2 µm SMX). Two-way ANOVA indicated an interaction between the two treatments (P < 0.01); these conditions formed the basis of the microarray experiment to explore transcriptome-level changes that may underpin the interaction. Three Aux/IAAs identified by ANOVA exhibited Suc-dependent responses to SMX (IAA3, IAA6, and IAA29). IAA19 transcript abundance responded synergistically to cotreatment with Suc and SMX. B, Transcript abundance values are displayed in log2 scale. C, Heat map includes 117 probe sets that correspond to transcripts identified for which the effect of Suc and SMX together (columns 9–12) was at least 2-fold greater than the additive effect of treatment with SMX alone (columns 4–6) and Suc alone (columns 7–9). Each column corresponds to a discrete biological sample, and all treatments are presented as biological triplicate replicates. Red indicates higher, and blue indicates lower, levels of transcript abundance. Hierarchical clustering performed using Pearson correlation coefficients, and data are row normalized to identify trends in transcript abundance across treatments irrespective of absolute abundance. D to E, In the absence of SMX, IAA6::GUS and IAA19::GUS expression is observed throughout the hypocotyl tissues of 7-d-old dark-grown seedlings; cotreatment of Suc and SMX caused a restriction of reporter expression. Images were taken along the center of the hypocotyl, midway between the shoot apical meristem and the root-hypocotyl junction. Bar = 50 µm.
Complementary statistical analyses were conducted to identify transcripts that may underpin cross talk between Suc and SMX. The first approach made use of a two-way ANOVA to identify transcripts that exhibit a Suc-dependent response to SMX (interaction). This interaction was significant for 19 transcripts (Table 1;Supplemental Table S2), including a number of cell wall-related enzymes and three members of the Auxin/Indole-3-Acetic Acid (Aux/IAA) family of transcriptional regulators, IAA3, IAA6, and IAA29. All three Aux/IAAs exhibited decreased transcript abundance in response to cotreatment with Suc and SMX (Fig. 4B).
A second approach was used to identify transcripts that exhibited synergistic responses to the cotreatment with Suc and SMX. Linear models were used to identify genes with differential transcript accumulation in which the effect of Suc and SMX together was at least 2-fold greater than the additive effect of treatment with Suc alone and SMX alone (synergy). A total of 117 transcripts met these criteria (Supplemental Table S3). The abundance values for these transcripts were row normalized and visualized using a heat map, so that trends in transcript accumulation across the four treatments could be observed, irrespective of the absolute abundance (Fig. 4C). Within this group, a fourth Aux/IAA was identified that has previously been shown to regulate auxin responses in the hypocotyl, IAA19/MASSUGU2 (MSG2) (Tatematsu et al., 2004). Similar to the three Aux/IAAs identified by the two-way ANOVA, IAA19 was characterized by decreased transcript abundance in response to the cotreatment of Suc and SMX (Fig. 4B).
Promoter::GUS fusions were generated to assess the spatial regulation of each of the four Aux/IAAs identified in the microarray analysis. The promoter region of each gene was fused upstream of the uidA sequence, and GUS staining was assessed. IAA6::GUS and IAA19::GUS showed expression patterns that were consistent with the microarray analysis and supported the hypothesis that these Aux/IAAs may regulate responses to SMX and Suc in the hypocotyl. When grown in the absence of SMX, the IAA6::GUS reporter showed a high level of expression throughout the hypocotyl tissues (Fig. 4D). The presence of SMX restricted expression of the reporter when administered in the presence of Suc, and the interaction between Suc and SMX can be seen in the expression of the reporter at the four concentrations used in the microarray analysis (10 mm Suc and 0.2 µm SMX). Treating seedlings with both 10 mm Suc and 1 µm SMX together completely inhibited expression of the reporter in the hypocotyl, whereas treatment with 1 µm SMX in the absence of Suc still allowed moderate expression. The IAA19::GUS reporter exhibited expression throughout the hypocotyl, similar to the IAA6::GUS line (Fig. 4E). Treatment with SMX did not restrict IAA19::GUS expression independently of the presence of Suc; however, cotreatment with Suc resulted in expression of the construct exclusively in the vascular cylinder and inhibited expression in other tissues of the hypocotyl. Neither the IAA3::GUS nor the IAA29::GUS reporter showed any change in expression within the hypocotyl tissues in response to the treatments (Supplemental Fig. S3). Changes in the expression profiles of IAA3 and IAA29 were observed in other tissues, suggesting that the Suc-SMX interaction is not limited to the hypocotyl, and may be a more general response throughout the plant.
Interaction between Suc and SMX Is Dependent on Auxin Transport and Signal Transduction
To test whether auxin transport is necessary to mediate the interaction between Suc and SMX, plants were grown in the presence of Suc, SMX, and/or the inhibitor of polar auxin transport, naphthylphthalamic acid (NPA; Morgan, 1964). The presence of 1 µm NPA in the growth media attenuated the interaction between Suc and SMX and rescued hypocotyl elongation (Fig. 5A). NPA also affected hypocotyl elongation differently depending on whether Suc was present in the media or not. NPA restricted elongation in the absence of Suc yet exerted no effect in its presence, suggesting that despite equal hypocotyl lengths, auxin transport is regulated differentially between these two treatments. These data support the findings of the microarray experiment and suggest that the interaction between folates and Suc is dependent on polar auxin transport.
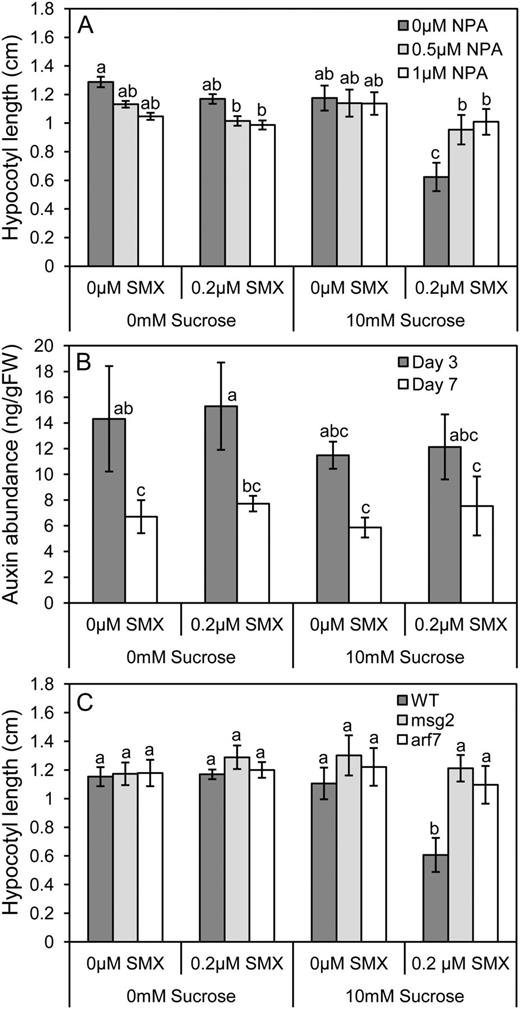
Auxin transport and signaling mediate synergy between Suc and SMX. A, Blocking polar auxin transport with NPA attenuated the synergistic effect of Suc and SMX on hypocotyl elongation. Data represent the mean ± se of three biological replicates from a representative experiment; each data point represents at least 36 individuals (P < 0.01; ANOVA followed by Tukey’s b test). B, IAA was extracted from 3- and 7-d-old dark-grown seedlings and quantified by liquid chromatography/mass spectrometry. No change in auxin abundance was observed in response to the Suc or SMX treatments, though seedlings sampled after 7 d of growth exhibited reduced auxin levels, on a per-gram fresh weight (FW) basis. Data represent the mean ± sd from three independent experiments (P < 0.05; ANOVA followed by Tukey’s b). C, The IAA19/ARF7 auxin-signaling pathway is necessary for the interaction between Suc and SMX. msg2 is a stable IAA19 allele resistant to degradation in the presence of auxin (Tatematsu et al., 2004), whereas arf7 is a loss-of-function mutant (Okushima et al., 2005). In both mutant backgrounds, synergy between Suc and SMX is attenuated and hypocotyl elongation is rescued. Data represent the mean ± se of three biological replicates from a representative experiment; each data point represents at least 36 individuals. Letters indicate values with statistically significant differences (P < 0.01; ANOVA followed by Tukey’s b test).
Given that SMX perturbs plant metabolism, it was hypothesized that the compound affects auxin homeostasis, which might explain the changes in Aux/IAA transcript abundance uncovered by the microarray experiment. To test this hypothesis, IAA was extracted from dark-grown seedlings raised in the presence of Suc, SMX, or a combination of the two treatments and quantified using liquid chromatography-mass spectrometry. Seedlings were sampled after 3 or 7 d of growth to assess changes in IAA abundance in response to growth treatments across time. Though IAA levels were lower in samples taken after 7 d of growth, there was no change in IAA abundance in response to the growth treatments (Fig. 5B). Auxin was also quantified from seedlings grown in higher concentrations of Suc and SMX (30 mm and 1 µm, respectively) to test whether there were concentration-dependent effects not observed at lower concentrations. Similar to the trends observed at lower concentrations, higher levels of Suc and SMX did not affect IAA abundance (Supplemental Fig. S4). It was concluded that any changes in auxin activity suggested by the transcriptome analysis were occurring independently of auxin levels.
As an alternate hypothesis, the role of auxin signal transduction in mediating cross talk between Suc and SMX was explored. This genetic analysis made use of mutants lacking the functional auxin-signaling components IAA19 and Auxin Response Factor7 (ARF7). IAA19 has an established role in mediating hypocotyl elongation in response to auxin and has been shown to interact with ARF7 (Tatematsu et al., 2004). A dominant negative allele of IAA19, msg2, is able to stably block auxin signal transduction, overcoming redundancy in the signaling pathway (Tatematsu et al., 2004). Similarly, the ARF7-dependent signaling pathway can be shut down by loss-of-function mutations in ARF7. Though many arf mutants do not exhibit mutant phenotype due to functional redundancy, arf7 loss-of-function mutants display reduced auxin sensitivity and tropic responses in the hypocotyl, suggesting that ARF7 may promote specific and partially nonredundant functions in auxin responses (Okushima et al., 2005). Both the dominant negative msg2 allele and the arf7 loss-of-function mutants were resistant to the inhibition of hypocotyl elongation induced by the action of SMX and Suc (Fig. 5C). These findings support the hypothesis that the interaction between Suc and SMX is dependent on auxin signaling and that phenotypes observed in the hypocotyl are likely related to the activity of the ARF7-dependent auxin response pathway.
Having established that cotreatment with Suc and SMX perturbed auxin signaling, it was hypothesized that treatment with FA would alter seedling responses to auxin. Treatment with exogenous IAA inhibits hypocotyl elongation in dark-grown seedlings (Tatematsu et al., 2004). This hypothesis was not supported, in that supplementing the growth media with concentrations of FA up to 50 µm did not alter responses to exogenous auxin (Supplemental Fig. S5). Similar findings were observed using lines with enhanced DHPS activity, where heightened DHPS expression had no effect on exogenous auxin (Supplemental Fig. S6), indicating that DHPS itself is not directly involved in auxin signaling. Taken together, it is concluded that though folates may be necessary to maintain normal endogenous auxin signaling, they are not sufficient to alter seedling responses to exogenous auxin.
Suc and SMX Promote Auxin Signaling in Vascular Tissues of the Hypocotyl
Two auxin-responsive reporter systems were used to assess changes in auxin signaling and accumulation within the hypocotyl, DII-VENUS and DR5. The DII-VENUS system was created by fusing the degron motif of Aux/IAA28 to the VENUS fluorescent protein (Brunoud et al., 2012). Due to the auxin-sensitive degron, the reporter is degraded in the presence of auxin. Consequently, cells with greater auxin concentrations have lower abundance of the reporter (Brunoud et al., 2012). Thus, the DII-VENUS reporter system inversely tracks the distribution of auxin in plant tissues (Brunoud et al., 2012). By contrast, intensity of the reporter in the DR5 system is greater in cells where auxin is being perceived. The DR5 system consists of tandem repeats of the Auxin-responsive element fused to a minimal promoter (Ulmasov et al., 1997), driving the expression of a reporter gene (Sabatini et al., 1999).
When raised in the absence of Suc, the DII-VENUS lines showed expression of the reporter in the nuclei of cells along the center of the hypocotyl in what appears to be the vasculature. In the absence of sugar, the reporter was observed along the vasculature region irrespective of the presence of SMX. Seedlings grown in the presence of both Suc and SMX exhibited less expression of the reporter in the vascular region, and at higher concentrations of SMX, expression of the reporter was greatly restricted (Fig. 6A).
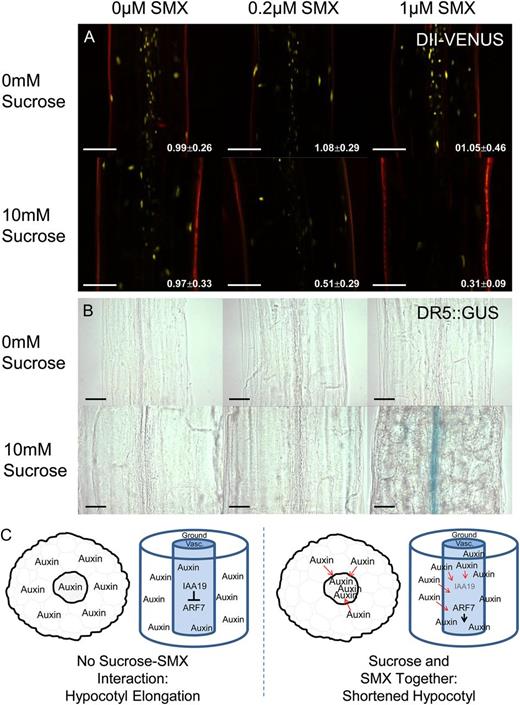
Cotreatment of Suc and SMX promotes auxin signaling in the hypocotyl. The DII-VENUS reporter system exhibited high levels of fluorescence in the vascular region of the hypocotyl. Treatment with SMX and Suc caused a decrease in VENUS fluorescence, yet this effect was not observed when seedlings were treated with SMX or Suc alone. White numbering indicates the ratio of fluorescence intensity in vasculature relative to the ground tissues (mean ± sd for at least 10 individuals). A, Fluorescence decreased preferentially in the vasculature compared with the ground tissues. Bar = 100 µm. B, DR5::GUS expression was observed in the hypocotyl vascular region during growth in the presence of both Suc and SMX, suggesting auxin signaling is enhanced by the combined action of these two treatments. Bar = 50 µm. Images were taken along the center of the hypocotyl, midway between the shoot apical meristem and the root-hypocotyl junction. C, A proposed model depicting changes in auxin distribution that may underpin the Suc-SMX interaction. In the absence of SMX, auxin is evenly distributed at low concentrations throughout the hypocotyl. SMX causes auxin accumulation in the vasculature, resulting in hypocotyl inhibition via IAA19/ARF7 signaling.
Fluorescence intensity was quantified to assess tissue-specific changes in auxin distribution in response to Suc and SMX. The fluorescence intensity of nuclei from vascular cells relative to those of ground tissue cells was represented as a ratio, where a value of 1 indicated equal distribution across vascular and ground tissues. In the absence of Suc, the ratio of fluorescence remained close to 1, irrespective of the presence of SMX. This indicated that auxin remained evenly distribution between the tissues of the hypocotyl. SMX caused a decrease in fluorescence in the vasculature, indicated by a reduced ratio of intensity between vasculature and ground tissues. These data support the qualitative assessment that auxin appears to accumulate preferentially in the vascular tissues in response to Suc and SMX.
Auxin signaling as indicated by the DR5 reporter system was consistent with the trends observed using the DII-VENUS system. Treatment with either SMX or Suc individually did not induce expression of the DR5::GUS reporter, nor was expression observed in response to the combined treatments of Suc and SMX at lower concentrations (Fig. 6B). By contrast, expression of the reporter appeared within the vascular region of plants treated with 1 µm SMX, but only in the presence of Suc. Taken together, the reporter systems indicate that the interaction between Suc and SMX promotes auxin accumulation in the vasculature of the hypocotyl.
Based on these data, a model is proposed in which folate deprivation caused by SMX results in changes to auxin distribution. Treatment with SMX resulted in auxin becoming localized to the vasculature, where IAA19/ARF7-dependent signaling mediates responses to auxin and inhibits hypocotyl elongation (Fig. 6C). The accumulation of auxin in the vasculature is likely the result of transport from other tissues, as evidenced by the attenuation of this affect by treatment with NPA.
Number of probe sets with significant main effect or interaction determined by ANOVAa
Benjamini-Hochberg adjusted P < 0.1 (Benjamini and Hochberg, 1995).
Benjamini-Hochberg adjusted P < 0.1 (Benjamini and Hochberg, 1995).
DISCUSSION
Combination chemical genetics can be used to overcome genetic redundancy and probe molecular pathways for interaction (Lehár et al., 2007, 2008). By screening for compounds that act synergistically on a given phenotype, connectivity between targeted pathways can be explored (Lehár et al., 2007, 2008). As exogenous Suc exerts a mild restrictive effect on etiolated hypocotyl elongation, it was reasoned that Suc could be used as the base compound in a combination chemical screen to identify pathways that modify sugar perception. Through this screen, an interaction between folate biosynthesis and Suc signaling was uncovered. These two pathways appeared to influence auxin signaling through the regulation of a subset of Aux/IAAs. In this sense, the metabolic system has been perturbed by restricting folate biosynthesis, and a small and clearly defined signaling mechanism was changed as a result. Metabolic, hormonal, and developmental pathways represent a web of interconnected signaling processes that are integrated to shape growth and development, and it is possible that during normal seedling, development folates and Suc act in concert to shape auxin distribution and sensitivity.
Forward genetic studies have been widely implemented to uncover the genetic basis for plant responses to sugars and have proven successful in uncovering multiple sugar perception and signaling pathways (Jang et al., 1997; Zhou et al., 1998; Xiao et al., 2000; Rook and Bevan, 2003; Rook et al., 2006a). Despite these successes, this approach is often limited by an inability to uncover mutant phenotypes masked by genetic redundancy, where a loss in the activity of one gene through mutation can be compensated by the activity of a related gene (McCourt and Desveaux, 2010; Tóth and van der Hoorn, 2010). The Arabidopsis genome contains two isoforms of DHPS (Storozhenko et al., 2007), which may explain why dhps mutants have not been uncovered in screens for sugar-sensitive mutants, as it is possible that either isoform is sufficient to maintain wild-type levels of metabolic flux through the THF biosynthetic pathway. The general inhibition by SMX is believed to restrict the activity of both isoforms equally and thus promote a phenotypic response while maintaining seedling viability. In this sense, treatment with SMX promoted a phenotype analogous to a double knockdown mutation that uncovered a role for DHPS in mediating Suc responses.
Though this study highlights a novel role for DHPS during seedling development, the effects of the folates in shaping plant growth have been well documented (Storozhenko et al., 2007; Van Wilder et al., 2009; Mehrshahi et al., 2010; Srivastava et al., 2011). A direct connection between folate and Suc was observed by Mehrshahi et al. (2010), who uncovered an important role for folylpolyglutamate synthetase (FPGS) across many facets of plant development, including hypocotyl and root elongation. Double fpgs2 fpgs3 mutants were seedling lethal but could be partially rescued by the presence of Suc (Mehrshahi et al., 2010). To our knowledge, this finding represents the only reported connection directly linking Suc to the folate pool; however, most studies involving folates were undertaken using light-grown materials, and it is possible that the interaction between folates and photosynthetically derived sugars underpin some phenotypes observed in these studies.
Treating the aerial tissues of dark-grown seedlings with Suc can induce profound changes in morphology, such as the initiation of leaf development and root elongation (Roldán et al., 1999). Though the promotion of these traits is generally thought to be underpinned by changes in local auxin distribution (Reinhardt et al., 2003; Grieneisen et al., 2007), no change in total auxin abundance was observed during growth in the presence of Suc. It is possible that the promotive effect of Suc on the development of these traits is a result of a redistribution of auxin rather than de novo biosynthesis. Though auxin has been proposed not to play a dominant role during etiolated hypocotyl elongation (Jensen et al., 1998), our data suggest that the effect of Suc and SMX on hypocotyl elongation is dependent on polar auxin transport. If Suc is promoting a change in auxin distribution, this may explain why blocking polar auxin transport attenuated the interaction between Suc and SMX.
Consistent with the notion that sugars promote auxin activity, Suc conferred hypersensitivity to exogenous IAA (Supplemental Figs. S5 and S6). As sugars can promote the development of traits often associated with auxin, such as leaf development and root elongation (Roldán et al., 1999; Kircher and Schopfer, 2012), this hypersensitivity to auxin may underpin some developmental responses to Suc. Though the treatments used in this study did not affect total auxin abundance, recent studies highlight the influence of sugars on auxin accumulation during seedling development (Lilley et al., 2012; Sairanen et al., 2012). The hypothesis that Suc promotes auxin activity in etiolated seedlings is supported by the observation that transcript abundance of many auxin-related signaling components was changed by growth in the presence of Suc (Supplemental Table S4).
Folate deprivation resulted in changes to auxin distribution, resulting in the inhibition of hypocotyl elongation. Quantifying DII-VENUS expression indicated a preferential accumulation of auxin in the vascular tissues, relative to ground tissues, in response to SMX. It is possible that folates are especially important in the vasculature and that phenotypic responses to folate deprivation are a result of tissue-specific signaling. Blocking the effect of SMX with NPA suggests that auxin may be transported from neighboring tissues to the vasculature in response to the sulfonamide, though the possibility that differential rates of auxin synthesis and metabolism between tissue types is contributing to this distribution cannot be ruled out. Though folates are not sufficient to alter responses to exogenous IAA, they are necessary to maintain normal auxin signaling during etiolated hypocotyl elongation. It may be that auxin inhibits hypocotyl elongation by signaling in the ground tissues or through signaling pathways not influenced by folates.
Aux/IAAs are core components of auxin signal transduction that negatively regulate auxin signaling through their interaction with ARFs (Hagen and Guilfoyle, 2002; Liscum and Reed, 2002; Tiwari et al., 2003). In the presence of auxin, Aux/IAAs are degraded, allowing ARFs to mediate transcription of auxin-responsive genes (Tiwari et al., 2001; Liscum and Reed, 2002). The four Aux/IAAs identified in the microarray analysis have previously been shown to regulate hypocotyl elongation (Kim et al., 1996; Reed et al., 1998; Tatematsu et al., 2004; Koini et al., 2009). IAA3/SHORT HYPOCOTYL2 (SHY2) and IAA6 were originally identified in genetic screens for suppressors of the long-hypocotyl phenotypes of phytochrome B (phyB) and elongated hypocotyl2 (hy2) mutants, respectively (Kim et al., 1996; Reed et al., 1998). In both cases, dominant alleles were isolated that suppress hypocotyl elongation and were posited to impinge on light-mediated hypocotyl elongation (Kim et al., 1998; Reed et al., 1998). IAA19/MSG2 was identified in a screen for mutants exhibiting auxin insensitivity in the hypocotyl and has been shown to mediate tropic responses (Tatematsu et al., 2004; Saito et al., 2007). Though less is known about the specific role of IAA29, a study into plant growth at high temperatures found that IAA29 mediated hypocotyl elongation in response to heat stress (Koini et al., 2009). In a number of different contexts, these Aux/IAAs have been found to regulate hypocotyl development in response to both environmental and endogenous stimuli, suggesting that perhaps they might serve as a point of integration for multiple regulatory inputs.
Transcriptional reporters for each of the four Aux/IAAs identified by the microarray experiment supported the hypothesis that interplay between Suc and SMX impinged on the expression of these Aux/IAAs. The IAA6::GUS reporter exhibited marked changes in activity throughout the hypocotyl, suggesting this regulator may play a more prominent role in the hypocotyl phenotype observed by treatment with SMX and Suc. Similarly, altered IAA19::GUS expression was observed in the ground tissues of the hypocotyl. These changes were similar to those reported by Saito et al. (2007), who observed that during tropic responses, IAA19 expression was observed in expanding cells of the hypocotyl ground tissue layers and inhibited in cells that were restricted in elongation. Perhaps this regulation underpins the short-hypocotyl phenotype, as a restriction of IAA19 expression may result in an inhibition of cell expansion throughout the ground tissues of the hypocotyl. Given that the transcription of Aux/IAAs is activated by auxin (Abel et al., 1995), it may be that the decrease in IAA6::GUS and IAA19::GUS expression in the ground tissue, and the concomitant increase in IAA19::GUS expression in the vascular tissues, indicated a change in auxin distribution (Fig. 6C). In this model, auxin becomes transported from the ground tissue to the vasculature, where IAA19/ARF7-dependent signaling occurs to restrict hypocotyl elongation. The genetic and pharmacogenetic analyses supported this hypothesis, as either the inhibition of polar auxin transport or the inactivation of the IAA19/ARF7-signaling pathway resulted in insensitivity to the Suc-SMX interaction.
Given the high level of redundancy within the Aux/IAA gene family (Overvoorde et al., 2005), it is possible that all four Aux/IAAs uncovered by the array analysis act in concert to modify plant growth in response to metabolic cues. Changes to Aux/IAA transcript abundance has been observed in response to treatment with hormones (Nakamura et al., 2003), as well as changes to nutrient status (Falkenberg et al., 2008), and may offer a point of cross talk through which other pathways may modify auxin signaling (Nakamura et al., 2003). By adjusting the abundance of these negative regulators, it may be possible that nutrient status can fine-tune auxin sensitivity. Currently, the mechanism through which SMX promotes changes to Aux/IAA transcript abundance remains unclear, and whether the compound directly regulates Aux/IAA transcript levels, or whether the changes in overall transcript abundance are a result of changed auxin distribution in the seedling, remains to be tested. Future work will explore the mechanisms through which auxin signaling and distribution are regulated in response to nutrient cues during seedling development.
MATERIALS AND METHODS
Plant Material and Growth Conditions
Wild-type Arabidopsis (Arabidopsis thaliana ecotype Columbia) seeds were obtained from the Nottingham Arabidopsis Stock Centre; all transgenic lines and mutants are of the Columbia ecotype. Plants harboring the DR5::GUS construct were described previously (Sabatini et al., 1999), as were the dominant negative IAA19 allele (msg2) and the arf7 loss-of-function mutants (Tatematsu et al., 2004; Okushima et al., 2005). All plant materials were grown in Conviron growth cabinets at 21°C in a 16-h/8-h photoperiod at 135 µmol m–2 s–1.
Chemical Screen
Seeds were sown in 96-well microtiter plates containing 200 µL 1× Murashige and Skoog (MS) liquid growth media (Murashige and Skoog, 1962), with alternate rows supplemented with 30 mm Suc. Gamborg’s vitamin solution (Sigma-Aldrich) was added (0.1% [v/v]) after autoclaving the media (Gamborg et al., 1968). Chemicals were drawn from the LATCA, provided by Sean Cutler (University of California, Riverside), and adjusted to a final concentration of 2.5 µm in each well. Approximately 20 seeds were added to each well in the microtiter plate. The plates were wrapped in aluminum foil and cold stratified at 4°C, after which they were exposed to 6 h of light to promote germination, rewrapped in the foil, and then transferred to the growth chamber. After 7 d in the growth cabinet, the microtiter plates were unwrapped, the growth media was drawn from the wells, and the seedlings were fixed by adding approximately 250 µL Farmer’s Fixative (ethanol:acetic acid [3:1]) to each well, ensuring that all seedlings were entirely submersed in the fixative. Upon rehydration using autoclaved distilled water, seedlings were aligned on agarose plates and photographed using a Canon EOS Rebel XT EF-S 18-55 digital camera. Hypocotyl lengths were measured using ImageJ software (http://rsbweb.nih.gov/ij/).
Dose Response Curves and Chemical Treatments
Dose response curves and chemical complementation analyses made use of a concentrated stock solution of each chemical dissolved in dimethyl sulfoxide. Experimental concentrations were adjusted from this stock solution by dilution in sterile liquid growth media. An equivalent volume of dimethyl sulfoxide was added to MS medium as a control. Seeds were sterilized as described above and sown in 24-well microtiter plates in 2 mL full-strength MS growth media supplemented with the experimental treatments (Murashige and Skoog, 1962). Plates were then wrapped in light-blocking foil and cold stratified for 3 d at 4°C. After stratification, plates were unwrapped and exposed to light for 6 h to promote germination. Plates were wrapped in foil and transferred to a Conviron growth cabinet at 21°C in a 16-h/8-h photoperiod at 135 µmol m–2 s–1. After 7 d of growth in the cabinet, seedlings were fixed, aligned on agarose plates, and measured as described above.
Tissue Preparation, RNA Extraction, and Microarray Analysis
Columbia seedlings were grown in 24-well microtiter plates in MS liquid media (Murashige and Skoog, 1962), supplemented with 10 mm Suc, 0.2 µm SMX, or both 10 mm Suc and 0.2 µm SMX together. Four plates, comprising three experimental treatments and one nontreatment control, were prepared together using sterilized seeds as described above. The four plates were each individually wrapped in light-blocking foil and cold stratified at 4°C for 3 d. The plates were then unwrapped and exposed to light for 6 h to promote germination, after which they were wrapped and placed in a Conviron growth chamber as described above. Together, these four plates comprise one biological replicate; the experiment was repeated three times to generate three biological replicates for the microarray experiment. After 3 d of growth, hundreds of seedlings were harvested from each plate under a green safelight, snap frozen in liquid nitrogen, and ground to a fine powder in a mortar and pestle. RNA was extracted using the RNeasy RNA extraction kit (Qiagen) and was precipitated overnight in 3 m sodium acetate at –80°C to achieve concentrations necessary for prehybridization procedures. RNA quality was determined electrophoretically. For each sample, 5 μg of total RNA was reverse transcribed (SuperScript II, Invitrogen), labeled, and hybridized to the Arabidopsis ATH1 Genome Array according to manufacturer’s protocols (Affymetrix) at the Centre for the Analysis of Genome Evolution and Function at the University of Toronto.
GeneChip data analysis was performed using the Bioconductor suite (Gentleman et al., 2004) in R statistical programming language (R Development Core Team, 2009; http://www.R-project.org) using the affy package (Gautier et al., 2004). All 12 arrays were preprocessed together using GeneChip-robust multiarray analysis (Wu et al., 2004). Expression data were filtered to remove probe sets, which reported low transcript abundance and low variance across all arrays (minimum intensity of 100 on at least two arrays, minimum interquartile range of 0.5 on the log2-scale). The preprocessed data were analyzed as a 2 × 2 factorial complete randomized ANOVA using the linear model for microarray package in R (R Development Core Team, 2009). The linear model was parameterized by group means with a manually defined sum-to-zero contrast matrix to test directly for the contrasts of interest: the main and interaction effects overall, as well as the effect of the cotreatment with Suc and SMX. A Benjamini-Hochberg false discovery rate of 0.1 was applied to the output of all tests (Benjamini and Hochberg, 1995). Data, description of the experimental design, and methods are available for download as Gene Expression Omnibus accession GSE37484.
Quantitative Reverse Transcription-PCR
Total RNA was prepared as described above, except that RNA was treated with DNase to reduce risk of amplification from genomic DNA. A total of 4 µg RNA was used for complementary DNA synthesis from oligo(dT)18 with SuperScript II Reverse Transcriptase (Invitrogen), following the manufacturer’s instructions. Real-time quantitative PCR was performed using the iCycler iQ real-time PCR detection system (Bio-Rad). The relative abundance of cDHPS and mDHPS transcripts were determined by the Pfaffl method (Pfaffl, 2001), using ACTIN2 as a reference gene. A melting curve was performed to assess primer specificity. The primers used were as follows: mDHPS, forward, 5′-TGTTGATAATGATACAGTTGC-3′, reverse, 5′-CTCAAGTAGAGTTCAGAAGCA-3′; cDHPS, forward, 5′-GACTATGGGATCAGTGTAACC-3′, reverse, 5′-CCTTTGTATAATACCGTCTTT-3′; and ACT2, forward, 5′-GGCTCCTCTTAACCCAAAGGC-3′, reverse, 5′-CACACCATCACCAGAATCCAGC-3′.
Creation of GUS Reporter Constructs and Histochemical Analysis
Four IAA promoter::GUS constructs were created using the Clontech In-Fusion reporter system (Clontech). For each construct, primers were designed to amplify 2 kb upstream of the transcriptional start site from genomic Columbia DNA, as follows: IAA3, forward, 5′-CCGGCGCGCCAAGCTGTCATTGGTTACACGTGTACG-3′, reverse, 5′-GATCTACCATGTCGAAACTTTGAGGCAAAAGTGTGT-3′; IAA6, forward, 5′-CCGGCGCGCCAAGCTTTCTCTCCCTCGCAAATGTCT-3′, reverse, 5′-GATCTACCATGTCGAATCTTGCTGGAGACCAAAACC-3′; IAA19, forward, 5′-CCGGCGCGCCAAGCTATCCTTGAGACTTGTAATATT-3′, reverse, 5′-GATCTACCATGTCGAATTGTAGTAATGGCACCGCGT-3′; and IAA29, forward, 5′-CCGGCGCGCCAAGCTAACAAAACATATGTCAATGTC-3′, reverse, 5′-GATCTACCATGTCGAGAATTAGAGGATCATGAGTAT-3′. These PCR products were incorporated into a modified pCAMBIA1390 by homologous recombination directly upstream of the uidA sequence. These plasmids were introduced into Escherichia coli by heat shock for amplification of plasmids, which were then extracted using a miniprep kit (Qiagen) and sequenced to ensure accuracy. These plasmids were transformed into Agrobacterium tumefaciens strain GV3101 by heat shock method. Arabidopsis ecotype Columbia was then inoculated using the floral dip method (Clough and Bent, 1998). Successful T1 transformants were selected by growth on medium containing 25 µg mL–1 hygromycin.
To facilitate GUS staining, seedlings were immersed in 90% (v/v) acetone at –20°C for 20 min, followed by two rinses in 50 mm sodium phosphate buffer to remove acetone. GUS expression was assessed by incubating seedlings at 37°C in GUS buffer containing 50 mm sodium phosphate, pH 7, 1 mg mL–1 (w/v) 5-bromo-4-chloro-3-indolyl-β-d-glucuronide (Sigma-Aldrich), 0.5 mm K3Fe(CN)6, 0.5 mm K4Fe(CN)6, and 10 mm EDTA. Staining times vary between constructs. IAA6::GUS was incubated for 16 h; IAA3::GUS, IAA19::GUS, IAA29::GUS, and DR5::GUS were incubated for 24 h. After staining, seedlings were rinsed in autoclaved distilled water, followed by fixation in formaldehyde-acetic acid (50% (v/v) ethanol, 10% (v/v) acetic acid, and 10% (v/v) formaldehyde). Tissues were cleared using a chloral hydrate solution (125 g chloral hydrate in 50 mL 30% (v/v) glycerol solution) as described previously (Willemsen et al., 1998). Seedlings were observed using an Olympus BX5 microscope, and images were captured with a QImaging MicroPublisher 3.3RTV digital camera using QCapture version 2.7 software.
Confocal Microscopy
The DII-VENUS reporter was visualized using a Leica SP5 confocal microscope. An argon laser at 514 nm was used for excitation, while emittance was measured at wavelengths between 518 nm and 560 nm (yellow channel/VENUS) and between 595 nm and 690 nm (red channel/propidium iodide). Hypocotyls were counterstained with propidium iodide by transferring seedlings to the appropriate growth media containing 10 µg mL–1 propidium iodide (Sigma-Aldrich). This was performed under a green safe light, while being careful to disturb the seedlings as little as possible. Images were prepared using Leica Application Suite Advanced Fluorescence Lite. Fluorescence was quantified using ImageJ. The intensities of at least 10 randomly selected nuclei were quantified from both the vasculature and the ground tissue. For each nucleus, a neighboring region was also measured for intensity to subtract background noise. A ratio of intensity of vasculature cells and the ground tissue cells was generated for 10 individuals and averaged for each treatment.
Auxin Quantification
IAA was extracted from whole dark-grown seedlings after 3 or 7 d of growth using solid-phase extraction as described by Preston et al. (2009) with minor modifications. Approximately 10 to 15 mg of frozen tissue was submerged in 1 mL of 80% (v/v) methanol containing 1% (v/v) glacial acetic acid along with 300 picograms of deuterated IAA (Sigma-Aldrich) as an internal standard. Tissues were disrupted in a TissueLyser II (Qiagen) and stored at 4°C overnight. The samples were centrifuged to remove debris, and the pellet was washed twice. The supernatant was evaporated in a SpeedVac, reconstituted in 1 mL of 1% (v/v) acetic acid, and passed through a preequilibrated Oasis HLB column (Waters) according to the manufacturer’s instruction. The IAA fraction was washed with 1 mL of water containing 1% (v/v) acetic acid and eluted with 1 mL methanol containing 1% (v/v) acetic acid. The sample was evaporated in a SpeedVac and reconstituted in 1 mL of water containing 1% (v/v) acetic acid. The resultants were applied to preconditioned Oasis WAX columns (Waters), washed with 1 mL of methanol, and eluted with 1 mL of methanol containing 1% (v/v) acetic acid. The solvent was removed under vacuum and subjected to liquid chromatography electrospray ionization tandem mass spectrometry (Agilent 6410 TripleQuad liquid chromatography/mass spectrometry system). A liquid chromatograph (Agilent 1200 series) equipped with a 50 mm × 2.1 mm, 1.8-μm Zorbax StableBond-Phenyl column (Agilent) was used with a binary solvent system comprising 0.01% (v/v) acetic acid in water (solvent A) and 0.05% (v/v) acetic acid in acetonitrile (solvent B). Separations were performed using a gradient of increasing acetonitrile content and an initial flow rate of 0.2 mL min–1. The gradient was increased linearly from 97% A and 3% B to 50% A and 50% B over 15 min, and then acetonitrile content was increased linearly to 98% over 1 min and held for 1 min. This condition was held for an addition 0.5 min with an increased flow rate of 0.3 mL min–1. After 1 min, the initial condition was restored and allowed to equilibrate for 7.5 min for the next analysis. The retention time of IAA and d2-IAA was 11.6 min. Tandem mass spectrometry conditions were as follows: capillary 4.0 kV; source temperature, 100°C; desolvation temperature, 350°C; cone gas flow, 0 L min–1; desolvation gas flow, 12 L min–1; fragmentor, 110; collision energy, 18; polarity, positive; and tandem mass spectrometry transition, 178/132 mass-to-charge ratio for d2-IAA and 176/130 mass-to-charge ratio for endogenous IAA. A calibration curve was made using MassHunter software.
Supplemental Data
The following materials are available in the online version of this article.
Supplemental Figure S1. Suc confers hypersensitivity to sulfonamides.
Supplemental Figure S2. Plants with enhanced DHPS activity exhibit resistance to the Suc-SMX interaction.
Supplemental Figure S3. Suc and SMX do not affect IAA3::GUS and IAA29::GUS expression in the hypocotyl.
Supplemental Figure S4. Auxin abundance is unchanged by growth in the presence of Suc and SMX.
Supplemental Figure S5. FA is not sufficient to alter sensitivity to exogenous auxin.
Supplemental Figure S6. Enhanced DHPS activity does not alter the effect of exogenous auxin on hypocotyl elongation.
Supplemental Table S1. Complete list of positive hits from initial chemical screen.
Supplemental Table S2. Probe sets with significant Suc-SMX interaction.
Supplemental Table S3. Probe sets exhibiting synergy to cotreatment with Suc and SMX.
Supplemental Table S4. Summary of probe sets corresponding to core auxin-signaling components with significant Suc main effect.
ACKNOWLEDGMENTS
We thank Joan Ouellette and Thanh Nguyen for excellent technical assistance, Hilda Doan and Ray Persaud for assistance with data collection, Dr. Kotaro Yamamoto for sharing previously published materials, and anonymous reviewers for the thoughtful and helpful commentary.
Glossary
- THF
tetrahydrofolate
- LATCA
Library of Active Compounds in Arabidopsis
- SMX
sulfamethoxazole
- pABA
para-aminobenzoic acid
- FA
folic acid
- NPA
naphthylphthalamic acid
- MS
Murashige and Skoog
LITERATURE CITED
Author notes
This work was supported by the Centre for Analysis of Genome Evolution and Function at the University of Toronto, the Natural Science and Engineering Research Council of Canada (to M.M.C.), the Canadian Foundation for Innovation (to M.M.C.), and the University of Toronto (to M.M.C.).
Present address: Center for Genomics and Systems Biology, New York University, 12 Waverly Place, New York, NY 10003.
Corresponding author; e-mail [email protected].
The author responsible for distribution of materials integral to the findings presented in this article in accordance with the policy described in the Instructions for Authors (www.plantphysiol.org) is: Malcolm M. Campbell ([email protected]).
The online version of this article contains Web-only data.
Open Access articles can be viewed online without a subscription.