-
PDF
- Split View
-
Views
-
Cite
Cite
Peng Wang, Yu Li, Zhe Liu, Xuhan Li, Yicheng Wang, Weijuan Liu, Xiao Li, Jianjian Hu, Wenyi Zhu, Changquan Wang, Shan Li, Tingting Gu, Dongqing Xu, Chao Tang, Yingtao Wang, Chao Li, Shaoling Zhang, Juyou Wu, Reciprocal regulation of flower induction by ELF3α and ELF3β generated via alternative promoter usage, The Plant Cell, Volume 35, Issue 6, June 2023, Pages 2095–2113, https://doi.org/10.1093/plcell/koad067
- Share Icon Share
Abstract
Flowering is critical for sexual reproduction and fruit production. Several pear (Pyrus sp.) varieties produce few flower buds, but the underlying mechanisms are unknown. The circadian clock regulator EARLY FLOWERING3 (ELF3) serves as a scaffold protein in the evening complex that controls flowering. Here, we report that the absence of a 58-bp sequence in the 2nd intron of PbELF3 is genetically associated with the production of fewer flower buds in pear. From rapid amplification of cDNA ends sequencing results, we identified a short, previously unknown transcript from the PbELF3 locus, which we termed PbELF3β, whose transcript level was significantly lower in pear cultivars that lacked the 58-bp region. The heterologous expression of PbELF3β in Arabidopsis (Arabidopsis thaliana) accelerated flowering, whereas the heterologous expression of the full-length transcript PbELF3α caused late flowering. Notably, ELF3β was functionally conserved in other plants. Deletion of the 2nd intron reduced AtELF3β expression and caused delayed flowering time in Arabidopsis. AtELF3β physically interacted with AtELF3α, disrupting the formation of the evening complex and consequently releasing its repression of flower induction genes such as GIGANTEA (GI). AtELF3β had no effect in the absence of AtELF3α, supporting the idea that AtELF3β promotes flower induction by blocking AtELF3α function. Our findings show that alternative promoter usage at the ELF3 locus allows plants to fine-tune flower induction.
Background: Flowering is critical for sexual reproduction and fruit production. The circadian clock regulator EARLY FLOWERING3 (ELF3) serves as a scaffold protein in the evening complex that controls flowering. EARLY FLOWERING4 (ELF4) and the transcription factor LUX ARRHYTHMO (LUX) directly bind to ELF3 to form the evening complex (EC) that gates circadian clock-mediated physiological responses in multiple species. Alternative promoter usage has been identified as a critical response to environmental signals in thousands of genes. However, the function of alternative promoter usage in flowering time regulation, particularly in relation to ELF3 function, remains poorly understood.
Question: How does alternative promoter usage at the ELF3 locus regulate flowering?
Findings: Here we report that alternative promoter usage generates two transcript isoforms from the ELF3 locus. This phenomenon is conserved among plants such as Arabidopsis (Arabidopsis thaliana), soybean (Glycine max), tomato (Solanum lycopersicum), apple (Malus × domestica), and pear (Pyrus sp.). Our results indicate that the full-length transcript ELF3α and the shorter transcript ELF3β antagonistically regulate flower induction. The 2nd intron of the ELF3 locus is critical for ELF3β expression. We determined that the AtELF3β transcript isoform encodes a protein that promotes flowering by competitively binding to AtELF3α and thereby disrupting EC complex formation. Our findings thus establish a mechanism for fine-tuning flower induction that depends on manipulating transcript isoforms through alternative promoter usage.
Next steps: In further studies, we will investigate the detailed mechanisms by which the 2nd intron regulates ELF3β expression, and how ELF3β expression responds to the environment.
Introduction
Flowering is a key developmental decision during the lifetime of angiosperms. The number of flowers each plant produces strongly influences the yield of many crops. Pear (Pyrus sp.) is a fruit tree of the Rosaceae family that is distributed throughout the world. Similar to apple (Malus × domestica), flowering in pear can be divided into four stages: flower induction, flower initiation, floral organ differentiation, and blooming (Hanke et al. 2007). Several pear varieties produce relatively few flower buds; however, the molecular mechanisms determining floral number in pear are largely unknown.
The photoperiod, temperature, vernalization, gibberellic acid (GA), aging, and autonomous pathways all contribute to flower induction (Cerdan and Chory 2003; Strasser et al. 2009; Fornara et al. 2010), with annual and perennial plants sharing similar regulatory mechanisms and pathways for the commitment to flowering (Kotoda et al. 2010; Khan et al. 2014; Yarur et al. 2016; Zhang et al. 2016; Kinoshita and Richter 2020). Whereas defects in flower induction in annual plants result in delayed flowering, defects in flower induction in perennials reduce the number of flower buds produced (Agusti et al. 2020). Indeed, when annual plants reach the adult phase, their meristems typically transition to floral meristems. By contrast, a mature perennial plant can produce either a vegetative or a reproductive shoot. As a perennial fruit tree, flower induction in pear takes place after the cessation of spring shoot growth. Thus, the flower primordium can be observed in the flower bud before dormancy. When flower induction is blocked in perennial plants, the number of flower buds will decrease (Tan et al. 2006; Agusti et al. 2020).
EARLY FLOWERING3 (ELF3) plays an essential role in flowering by acting as a scaffold for protein–protein interactions in flowering-related protein complexes (Hicks et al. 2001; Huang and Nusinow 2016). In Arabidopsis (Arabidopsis thaliana), loss-of-function elf3 mutants display early flowering (Zagotta et al. 1996), while ELF3 overexpression delays flowering (Liu et al. 2001). ELF4 and the transcription factor LUX ARRHYTHMO (LUX) directly bind to ELF3 to form the evening complex (EC) that gates circadian clock-mediated physiological responses.
The transcript levels of ELF3, ELF4, and LUX follow diurnal and circadian rhythms that peak at dusk, and mutation in any EC component leads to changes in circadian rhythmicity, hypocotyl elongation, and flowering time (Nusinow et al. 2011; Silva et al. 2020). EC has been identified in several species, including soybean (Glycine max) (Bu et al. 2021), rice (Oryza sativa) (Andrade et al. 2022), and maize (Zea mays) (Zhao et al. 2023). Transcriptome analysis and chromatin immunoprecipitation followed by sequencing have revealed that the EC coordinates the expression of hundreds of key regulatory factors involved in photosynthesis, circadian clock, phytohormone signaling, growth, and responses to the environment (Ezer et al. 2017). Indeed, the EC directly regulates PSEUDO-RESPONSE REGULATOR7 (PRR7), PRR9, PHYTOCHROME INTERACTING FACTOR4 (PIF4), and GIGANTEA (GI) expression in the evening to control hypocotyl growth and flowering in Arabidopsis (Nusinow et al. 2011; Chow et al. 2012; Mizuno et al. 2014a; Mizuno et al. 2014b). PRR7 and PRR9 are clock-associated genes, and the prr7 prr9 double mutant exhibits a late-flowering phenotype under long-day conditions (Nakamichi et al. 2007). The overexpression of PIF4, which encodes a basic helix–loop–helix (bHLH) transcription factor, leads to earlier flowering (Araki and Komeda 1993), while loss-of-function mutations of GI, which encodes a plant-specific nuclear protein, is also associated with late flowering in long days (Mizoguchi et al. 2005; Kumar et al. 2012). ELF3 and ELF3-mediated regulatory circuits are functionally conserved in plants, with homologs of ELF3 identified in various plant species (Linde et al. 2017). ELF3 acts as a flowering repressor in Arabidopsis (Zagotta et al. 1996; Hicks et al. 2001; Huang and Nusinow 2016) and chickpea (Cicer arietinum) (Ridge et al. 2017) and as a flowering enhancer in soybean (Lu et al. 2017) and rice (Fu et al. 2009). ELF3 is also associated with flower development in perennial species, such as apple (Xing et al. 2016) and balsam poplar (Populus balsamifera) (Keller et al. 2012).
Alternative promoter usage expands the protein-coding repertoire of a genome (Quelle et al. 1995; Rojas-Duran and Gilbert 2012; Wiesner et al. 2015; Ushijima et al. 2017; Wrighton 2018). Genome-wide studies have shown that phytochromes modulate alternative promoter selection from over 2,000 genes in Arabidopsis to enable adaptation to changing light conditions (Ushijima et al. 2017). Likewise, thousands of Arabidopsis genes possess multiple transcription start sites (TSSs), whose variable use contributes to blue light-mediated regulation (Kurihara et al. 2018). Alternative promoter usage to produce distinct transcript isoforms may thus be a widespread molecular mechanism by which plants respond to various environmental signals.
In this study, we report that alternative promoter usage generates two transcript isoforms from the ELF3 locus. This phenomenon is conserved among plants such as Arabidopsis, soybean, tomato (Solanum lycopersicum), apple, and pear. Our results indicate that the full-length transcript ELF3α and the shorter transcript ELF3β antagonistically regulate flower induction. In pear, the transcript levels of PbELF3β were significantly lower in cultivars harboring a 58-bp deletion in the 2nd intron of the PbELF3 gene. Genetic evidence suggested that this 58-bp deletion was tightly associated with the production of fewer flower buds. We determined that the AtELF3β transcript isoform encodes a protein that promotes flowering by competitively binding to AtELF3α and thereby disrupting EC complex formation. Our findings thus establish a mechanism for fine-tuning flower induction that depends on manipulating transcript isoforms through alternative promoter usage.
Results
A 58-bp deletion in the 2nd intron of PbELF3 is associated with fewer flower buds
We evaluated the number of flower buds per branch in eight pear varieties over three consecutive years. ‘Kuikejuju’ (KKJJ), ‘Korla pear’ (KL), and ‘Lvjuju’ (LJJ) produced significantly fewer flower buds than ‘Dangshansuli’ (DS), ‘Yali’ (YL), ‘Cuiguan’ (CG), ‘Jinchuanxueli’ (JCXL), and ‘Housui’ (HS) (Fig. 1A; Supplemental Fig. S1). To identify the genes that regulate the number of flower buds in pear, we searched the genome resequencing data of these eight pear varieties for homologs of genes that affect flowering in other species (Wu et al. 2018). Among these genes, we identified ELF3 in pear (PbELF3). PbELF3 has four exons and three introns (Fig. 1B). We detected two haplotypes at the PbELF3 locus, which differed by the presence/absence of a 58-bp sequence (named the D region) in the 2nd intron (Fig. 1, B and C). PbELF3N (represented by the reference sequence from the DS variety) contained the 58-bp D region and was present in DS, YL, CG, JCXL, and HS varieties, which all produced more flower buds. By contrast, PbELF3D (represented by the sequence from the KL variety) lacked the 58-bp D region and was present in KKJJ, KL, and LJJ, which had fewer flower buds (Fig. 1C; Supplemental Fig. S1).
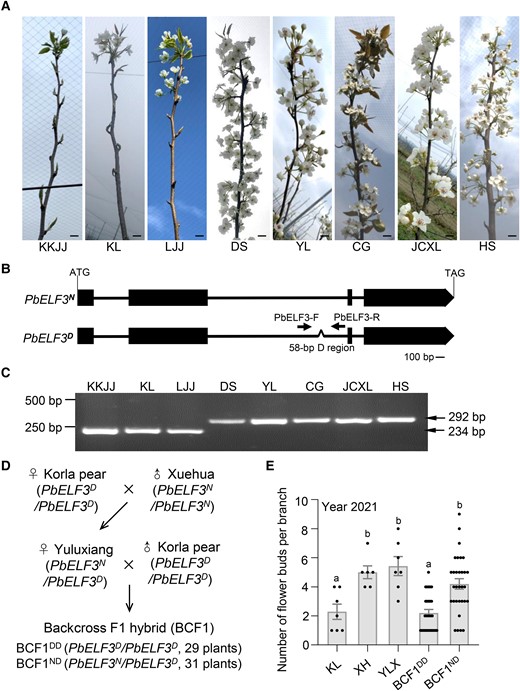
The 58-bp region in the PbELF3 gene is associated with the number of flower buds produced by pear trees. A) Representative photographs of the number of flower buds produced by different pear varieties: Kuikejuju (KKJJ), Korla pear (KL), Lvjuju (LJJ), Dangshansuli (DS), Yali (YL), Cuiguan (CG), Jinchuanxueli (JCXL), and Housui (HS). KKJJ, KL, and LJJ produce fewer flowers than DS, YL, CG, JCXL, and HS. Scale bar, 2 cm. B) Two types of gene structure for PbELF3. A 58-bp deletion was identified in the 2nd intron of the PbELF3D-type sequence. Black boxes indicate exons, lines indicate introns, and Λ indicates the 58-bp deletion. The arrows indicate the primers used to detect the 58-bp deletion. C) PCR analysis showing the presence of a 58-bp deletion in the 2nd intron of PbELF3 in KKJJ, KL, and LJJ pear varieties. The primers used are indicated in B). D) Genetic background of the backcross populations. Yuluxiang (YLX; with the PbELF3N/PbELF3D genotype) is the offspring of Korla (KL; with the PbELF3D/PbELF3D genotype, used as the female parent) and Xuehua (XH; with the PbELF3N/PbELF3N genotype, used as the male parent). The backcross F1 hybrid (BCF1) population of 60 independent trees was prepared by backcrossing YLX as the female parent to KL as the male parent. E) Number of flower buds per branch of KL, XH, YLX, and the hybrid offspring population. The population was divided into BCF1DD (PbELF3D/PbELF3D, homozygote, n = 29) and BCF1ND (PbELF3N/PbELF3D, heterozygote, n = 31) groups according to their genotype. Different letters indicate significant differences (P < 0.05), as determined using Duncan's multiple range test.
To test whether flower bud number was associated with PbELF3, we constructed a genetic hybrid population of pears. The pear variety ‘Yuluxiang’ (YLX; PbELF3N/PbELF3D) was previously obtained by crossing ‘Xuehua’ (XH; PbELF3N/PbELF3N) as the female parent and KL (PbELF3D/PbELF3D) as the male parent (Guo et al. 2001). We used KL as the female parent and YLX as the male parent to obtain a backcross F1 hybrid (BCF1) population (Fig. 1D). We determined their genotype at PbELF3 and scored the number of flower buds over two consecutive years for 60 BCF1 offspring. The PbELF3 genotype in the BCF1 population showed a close to 1:1 segregation ratio between PbELF3D/PbELF3D (n = 29) and PbELF3N/PbELF3D (n = 31), as expected (Fig. 1D; Supplemental Fig. S2). We also scored the number of leaf buds and flower buds per branch in a morphological analysis of longitudinal sections (Supplemental Fig. S3). The plants homozygous for PbELF3D (named BCF1DD, with the genotype PbELF3D/PbELF3D) had significantly fewer flower buds than the plants heterozygous for PbELF3 (named BCF1ND, with the genotype PbELF3N/PbELF3D) (Fig. 1E; Supplemental Fig. S4). These results suggest that PbELF3 is genetically associated with flower bud induction in pear.
To investigate the effects of the 58-bp PbELF3 region in flower induction, we placed the full-length genomic sequences of PbELF3N and PbELF3D under the control of the constitutive cauliflower mosaic virus (CaMV) 35S promoter for heterologous expression (HE). We introduced these constructs into the wild-type Arabidopsis accession Col-0, yielding the PbELF3N-HE and PbELF3D-HE transgenic lines (Supplemental Fig. S5A). Both sets of PbELF3N-HE and PbELF3D-HE transgenic lines flowered later than nontransformed control plants (Supplemental Fig. S5B), indicating that both PbELF3 versions negatively regulate flowering time, as is the case with Arabidopsis ELF3 (Liu et al. 2001). Compared to PbELF3N-HE plants, PbELF3D-HE plants flowered much later, indicating that the variation in genomic sequence at PbELF3 plays an important role in flowering (Supplemental Fig. S5, B and C).
A closer look at the PbELF3N and PbELF3D sequences identified three synonymous mutations in the fourth exon, a single nucleotide polymorphism (SNP) in the first intron, as well as a SNP and the 58-bp deletion in the 2nd intron of PbELF3D (Fig. 2A). To clarify whether the phenotypic differences observed in pear varieties were caused by the deletion or the other polymorphisms, we deleted the 58-bp D region in the PbELF3N sequence, resulting in the PbELF3N−D construct, and conversely inserted the 58-bp D region into PbELF3D to obtain the PbELF3D+D construct. We placed both variants under the control of the CaMV 35S promoter and introduced them into Col-0 plants (Fig. 2, A to C). The flowering time and number of rosette leaves were comparable in the PbELF3D+D-HE and PbELF3N-HE plants. Likewise, the flowering phenotypes of PbELF3N−D-HE plants were similar to those of PbELF3D-HE plants, both of which flowered later than the PbELF3D+D-HE or PbELF3N-HE plants (Fig. 2, B, D, and E). These results suggest that the 58-bp sequence in the 2nd intron of PbELF3 plays a critical role in flower induction.
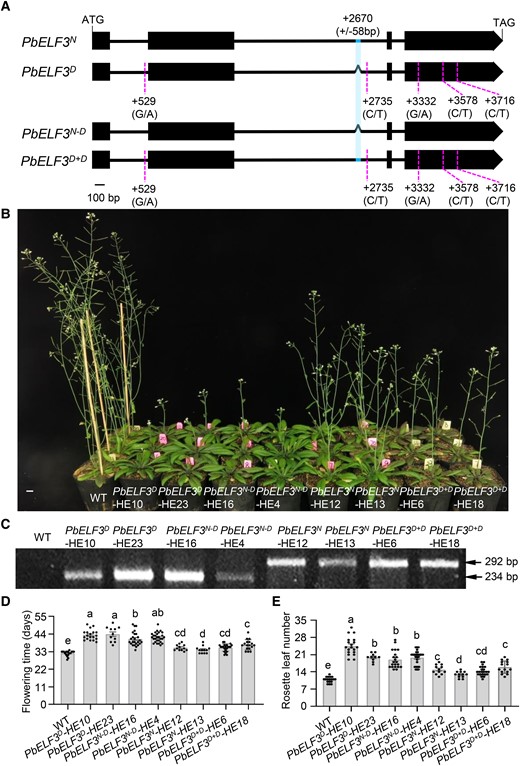
The 58-bp region, rather than SNP mutations, in the PbELF3 gene influences flowering time. A) Schematic diagram of the PbELF3 gene structure. Black boxes represent exons, dotted lines represent SNP sites with the polymorphisms under the corresponding location, and Λ indicates the 58-bp deletion. PbELF3N−D and PbELF3D+D are the artificially modified PbELF3N and PbELF3D sequences. PbELF3N−D represents PbELF3N in which the 58-bp sequence was artificially deleted; PbELF3D+D represents PbELF3D in which the 58-bp deletion of PbELF3D was artificially added. B) Flowering time phenotype of transgenic Arabidopsis plants harboring the PbELF3N, PbELF3D, PbELF3N−D, and PbELF3D+D transgenes. C) Genotype identification of PbELF3 transgenic lines using PbELF3-F and PbELF3-R primers as in Fig. 1C. D) Flowering time and E) number of rosette leaves at the opening of the first flower in the indicated independent transgenic lines overexpressing PbELF3: PbELF3N-HE12, PbELF3N-HE13, PbELF3D-HE10, PbELF3D-HE23, PbELF3N−D-HE16, PbELF3N−D-HE4, PbELF3D+D-HE6, and PbELF3D+D-HE18, grown in long-day conditions (16 h light/8 h dark). Data are means ± s.e.m. (n ≥ 10). Different letters indicate significant differences (P < 0.05), as determined using Duncan's multiple range test. Scale bar, 1 cm.
Alternative promoter usage produces the PbELF3β transcript isoform from the PbELF3 gene locus
To explore how the 58-bp sequence in the intron influences PbELF3 functions, we measured the transcript levels of PbELF3 in different pear varieties. However, the PbELF3 transcript levels did not correspond with the number of flowers produced by the eight pear varieties tested in this study (Fig. 3C), indicating that the 58-bp deletion does not affect PbELF3 transcript levels.
Alternative promoter usage produces multiple mRNAs whose individual transcription is initiated from different TSSs within a given locus (Landry et al. 2003; Davuluri et al. 2008; Ushijima et al. 2017). A reverse transcription PCR (RT-PCR) analysis detected a previously unknown transcript derived from the PbELF3 gene that included the 2nd intron (Fig. 3, A and B). As the current annotation of the pear genome does not include a transcript corresponding to this new transcript (Wu et al. 2013), we performed rapid amplification of cDNA ends (RACE) to clone full-length transcripts arising from PbELF3 based on the 2nd intron region. Using first-strand cDNAs prepared from total RNA extracted from leaves of the DS variety, we cloned a previously unknown transcript with a short length, designated PbELF3β, and named the currently annotated full-length PbELF3 transcript PbELF3α (Fig. 3A; Supplemental File S1). A comparison of gene models determined that the PbELF3β transcript contains a fragment from the 2nd intron sequence of the PbELF3α pre-mRNA, and the complete sequence of the third and fourth exons of PbELF3α, with the 58-bp D region located in the 5′ untranslated region (5′ UTR) of PbELF3β (Fig. 3A). The transcript levels of PbELF3β in the KKJJ, KL, and LJJ varieties (PbELF3D/PbELF3D genotype) were significantly lower than those in the other five varieties with the PbELF3N/PbELF3D genotype (Fig. 3D). These results indicate that the presence/absence of the D region is closely related to PbELF3β expression.
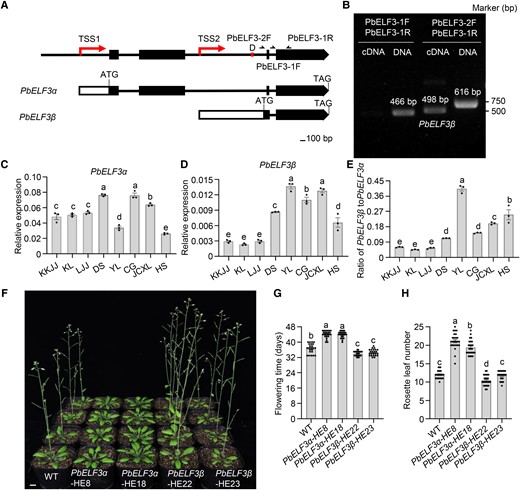
PbELF3β promotes flower induction. A) Schematic diagram showing the two transcriptional start sites (TSSs) in the PbELF3 genomic region. 5′ UTRs and coding sequences (exons) are represented by white and black boxes, respectively. Black lines indicate introns. PbELF3α encodes the full-length protein, while PbELF3β encodes a shorter predicted protein. D indicates the position of the 58-bp InDel. B) PCR analysis revealing two TSSs in the PbELF3 genomic region. The cDNA and genomic DNA samples were analyzed using the same primers, indicated in A). C), D) Reverse transcription quantitative PCR (RT-qPCR) analysis of PbELF3α(C) and PbELF3β(D) transcript levels in KKJJ, KL, LJJ, DS, YL, CG, JCXL, and HS. Data are means ± s.e.m. (n = 3). Different letters indicate significant differences (P < 0.05), as determined using Duncan's multiple range test. E) Ratios of PbELF3β and PbELF3α transcript levels in the eight pear cultivars. Data are means ± s.e.m. (n = 3). Different letters indicate significant differences (P < 0.05), as determined using Duncan's multiple range test. F) Flowering time phenotypes of nontransformed control plants, PbELF3α-HE8, PbELF3α-HE18, PbELF3β-HE22, and PbELF3β-HE23 lines under long-day conditions (16 h light/8 h dark). Scale bar, 1 cm. G), H) Flowering time (G) and number of rosette leaves (H) at the opening of the first flower for two independent transgenic lines of each genotype: PbELF3α-HE8, PbELF3α-HE18, PbELF3β-HE22, and PbELF3β-HE23 grown under long-day conditions (16 h light/8 h dark). Data are means ± s.e.m. (n ≥ 23). Different letters indicate significant differences (P < 0.05), as determined using Duncan's multiple range test.
We then characterized the expression pattern of PbELF3α and PbELF3β. Both PbELF3α and PbELF3β transcripts were present at high levels in leaves (Supplemental Fig. S6, A and B). A time course assay indicated that PbELF3α and PbELF3β were expressed with a diurnal rhythm and peaked in the night (Supplemental Fig. S6, C and D). Under continuous light, PbELF3α and PbELF3β both followed a circadian rhythm, peaking in the subjective night (Supplemental Fig. S6, E and F).
To assess the individual role of PbELF3α and PbELF3β in flower induction, we generated heterologous Arabidopsis transgenic lines expressing each coding sequence (Supplemental Fig. S7). PbELF3α-HE plants flowered later than nontransformed control plants, indicating that PbELF3α, like Arabidopsis ELF3 (Liu et al. 2001), negatively regulates flower induction. By contrast, PbELF3β-HE plants flowered earlier than nontransformed control plants, suggesting that PbELF3β promotes flowering (Fig. 3, F to H). Consistent with this role, the ratio of PbELF3β to PbELF3α transcript abundance in the KKJJ, KL, and LJJ varieties with fewer flower buds was much lower than that in the other five pear varieties (Fig. 3E). Thus, we have identified a transcript isoform PbELF3β produced from the PbELF3 locus by an alternative promoter that exerts a function opposite to that of PbELF3α in flower induction.
The short ELF3β transcript is present in other species
ELF3 encodes a signaling hub protein upon which light and temperature signals converge to regulate the circadian clock in plants (Huang and Nusinow 2016; Legris et al. 2017; Jung et al. 2020; Bu et al. 2021; Andrade et al. 2022; Zhao et al. 2023). Our results above revealed that the PbELF3β transcript isoform is produced in pear due to alternative promoter usage. We next examined whether a similar mechanism might also occur at the ELF3 genomic region in other species. Accordingly, we conducted RT-PCR and RACE analyses in Arabidopsis and detected a similar AtELF3β transcript (Fig. 4, A and B; Supplemental File S2). By analogy with PbELF3α, the previously reported full-length ELF3 transcript in Arabidopsis was renamed AtELF3α here. The translation start site of AtELF3β is located in the fourth exon of AtELF3α (Fig. 4A). The full-length AtELF3α transcript encodes a 695-amino acid (aa) protein, while the shorter AtELF3β transcript encodes a 278-aa protein with the same C-terminal sequence. We detected AtELF3α and AtELF3β transcripts in roots, stems, leaves, flowers, and siliques in Arabidopsis. Both AtELF3α and AtELF3β transcripts were present at high levels in leaves (Supplemental Fig. S8, A and B). The 2nd intron of AtELF3 has been found to be retained and cycled (Hazen et al. 2009); thus, we explored the time-dependent expression pattern of AtELF3β. Both AtELF3α and AtELF3β were expressed with a diurnal rhythm and peaked at night (Supplemental Fig. S8, C and D). In continuous light conditions, AtELF3α and AtELF3β both followed a circadian rhythm with peak expression in the subjective night (Supplemental Fig. S8, E and F). Moreover, we also detected the shorter ELF3β transcript from the ELF3 locus in soybean, apple, and tomato (Supplemental Fig. S9). Our data indicate the presence of a short transcript isoform produced from the ELF3 locus in diverse plant species, suggesting that a general regulatory mechanism underlies flowering time.
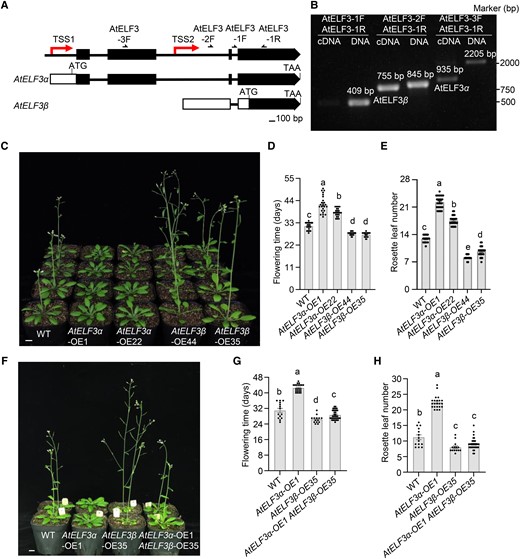
AtELF3α and AtELF3β oppositely regulate flower induction. A) The AtELF3 genomic region has two TSSs. The 5′ UTRs and coding sequences (exons) are represented by white and black boxes, respectively. Black lines indicate introns. AtELF3α encodes the full-length protein, while AtELF3β encodes the shorter form. B) PCR analysis reveals two TSSs in the AtELF3 genomic region. The cDNA and genomic DNA samples were analyzed using the same primers, as indicated in A). The AtELF3β band (755 bp) was amplified in cDNA samples using primers AtELF3-2F and AtELF3-1R. C) Phenotype of AtELF3α-OE-1, AtELF3α-OE-22, AtELF3β-OE-35, and AtELF3β-OE-44 under long-day conditions (16 h light/8 h dark). D), E) Flowering time (D) and number of rosette leaves (E) at the opening of the first flower shown in C). Data are means ± s.e.m. (n ≥ 22). Different letters indicate significant differences (P < 0.05), as determined using Duncan's multiple range test. Scale bar, 1 cm. F) Phenotype of transgenic Arabidopsis lines co-expressing AtELF3β and AtELF3α. G), H) Flowering time (G) and number of rosette leaves (H) at the opening of the first flower. Plants were grown in long-day conditions (16 h light/8 h dark). Data are means ± s.e.m. (n ≥ 14). Different letters indicate significant differences (P < 0.05), as determined using Duncan's multiple range test.
ELF3β-OE suppresses the late-flowering phenotype conferred by ELF3α-OE
To understand how AtELF3α and AtELF3β affect flowering in Arabidopsis, we generated transgenic lines overexpressing each transcript, cloned in-frame and upstream of the green fluorescent protein (GFP) sequence, in the Col-0 background (Fig. 4C). AtELF3α and AtELF3β were overexpressed in the AtELF3α-OE and AtELF3β-OE lines, respectively (Supplemental Fig. S10, A and B). We visualized GFP fluorescence in the root tip cells of seedlings from the resulting transgenic lines. AtELF3α-GFP accumulated in the nucleus as previously reported (Liu et al. 2001; Ronald et al. 2022), while AtELF3β-GFP localized in both the nucleus and cytoplasm (Supplemental Fig. S11). Compared to nontransformed control plants, AtELF3α-OE plants flowered later, which was consistent with a previous report (Liu et al. 2001). By contrast, AtELF3β-OE plants flowered earlier than nontransformed control plants, indicating that AtELF3β induces earlier flowering (Fig. 4, C to E). ELF3 also participates in hypocotyl growth (Nusinow et al. 2011); we observed shorter hypocotyls in the AtELF3α-OE and PbELF3α-HE lines relative to nontransformed control plants, while the AtELF3β-OE and PbELF3β-HE lines exhibited longer hypocotyls (Supplemental Fig. S12).
We further examined the genetic relationship between ELF3α and ELF3β. To this end, we crossed AtELF3α-OE plants with AtELF3β-OE plants to generate lines overexpressing both transcript isoforms (AtELF3α-OE AtELF3β-OE). The resulting AtELF3α-OE AtELF3β-OE F1 plants flowered earlier than AtELF3α-OE plants, suggesting that AtELF3β-OE partially suppresses the late-flowering phenotype imposed by AtELF3α-OE (Fig. 4, F to H), with AtELF3α and AtELF3β being co-expressed to similar levels as in their parental lines (Supplemental Fig. S13). We observed a similar phenotype when crossing Arabidopsis transgenic lines expressing PbELF3α and PbELF3β (Supplemental Fig. S14). In AtELF3β-OE plants, only considering the AtELF3β-GFP, the relative ratio of AtELF3β to AtELF3α transcript abundance was much higher than ratio of AtELF3β to AtELF3α in AtELF3α-OE plants and the ratio of native AtELF3β to AtELF3α in nontransformed control plants (Supplemental Fig. S10C). These results support the notion that ELF3β and ELF3α play opposite roles in flower induction.
Knocking down ELF3β transcript levels results in late flowering
To validate the role of AtELF3β, we generated gene-edited Arabidopsis lines via clustered regularly interspaced short palindromic repeat (CRISPR)/CRISPR-associated nuclease 9 (Cas9), carrying a deletion in the 2nd intron of the AtELF3 locus (Fig. 5, A and B). We obtained two independent lines, named AtELF3-Δ60 and AtELF3-Δ53, for analysis (Fig. 5, A and B). The transcript level of AtELF3β was significantly lower in both AtELF3-Δ60 and AtELF3-Δ53 compared to the nontransformed plants (Col-0), while the transcript level of AtELF3α was comparable in these lines (Fig. 5, C and D). AtELF3-Δ60 and AtELF3-Δ53 both showed a delay in flowering time and more rosette leaves (Fig. 5, E to G).
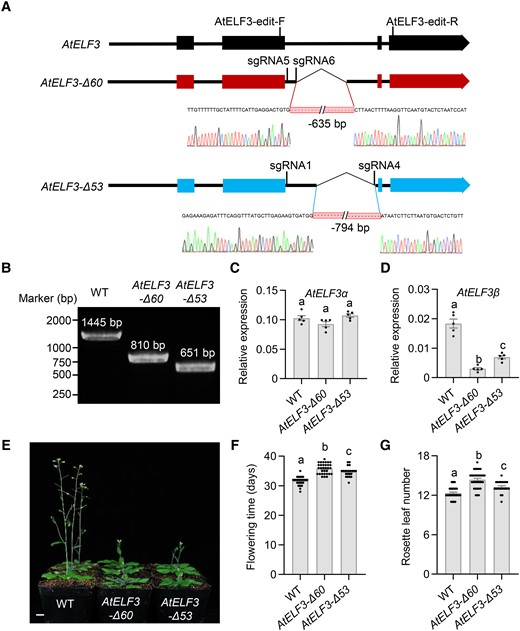
Deletion of the 2nd intron of AtELF3 results in AtELF3β downregulation and late flowering in Arabidopsis. A) Schematic diagram of the AtELF3 locus in the two AtELF3 CRISPR lines. The annotated AtELF3 gene structure is shown at the top; exons are marked as squares and introns as straight lines. The edited AtELF3-Δ60 and AtELF3-Δ53 lines are represented in the middle and bottom, respectively. Close-ups provide detailed information on the positions of the deletions as determined by Sanger sequencing of the CRISPR lines, aligned to the wild-type AtELF3 genomic sequence. B) Identification of the mutant site in AtELF3-Δ60 and AtELF3-Δ53 lines by PCR. C), D) Transcript level of AtELF3α(C) and AtELF3β(D) in AtELF3-Δ60 and AtELF3-Δ53 lines. Data are means ± s.e.m. (n = 5). Different letters indicate significant differences (P < 0.05), as determined using Duncan's multiple range test. E) Flowering time phenotypes of the nontransformed control plants (Col-0), AtELF3-Δ60, and AtELF3-Δ53 lines under long-day conditions (16 h light/8 h dark). Scale bar, 1 cm. F), G) Flowering time (F) and number of rosette leaves (G) at the opening of the first flower in plants grown under long-day conditions (16 h light/8 h dark). Data are means ± s.e.m. (n ≥ 22). Different letters indicate significant differences (P < 0.05), as determined using Duncan's multiple range test.
We independently transformed the elf3-3 mutant plants with a modified AtELF3 full genomic locus. Accordingly, we generated the genomic construct AtELF3-Δintron, in which the native AtELF3 promoter (2,969 bp upstream of the ATG) drove the expression of the AtELF3 genomic sequence lacking a 1,080-bp fragment from the 2nd intron. We also cloned the full-length intact AtELF3 sequence as a positive control (Supplemental Fig. S15A). Transgenic elf3-3 mutant plants harboring the full-length intact AtELF3 construct flowered at a similar time as nontransformed control plants, indicating full complementation by this construct (Supplemental Fig. S15, B to D). By contrast, the AtELF3-Δintron lines showed delayed flowering time and more rosette leaves relative to nontransformed control plants (Supplemental Fig. S15, B to D). However, because elf3-3 is a point mutant (E281stop; Hicks et al. 2001), the transcript level of AtELF3β was similar in elf3-3 and the nontransformed control plants (Supplemental Fig. S15F). The expression of AtELF3α was higher in both AtELF3-full and AtELF3-Δintron lines, which might be caused by additional copies of AtELF3 (Supplemental Fig. S15E). The transcript levels of AtELF3β in the AtELF3-Δintron lines were lower than in transgenic lines harboring the full-length intact AtELF3 construct (Supplemental Fig. S15F). The ratio of AtELF3β to AtELF3α transcript abundance in AtELF3-Δintron lines was also lower than that in the full-length intact AtELF3 transgenic lines (Supplemental Fig. S15G). Thus, the 2nd intron of AtELF3 appears to be critical for AtELF3β expression, and the lower expression of AtELF3β is associated with a late-flowering phenotype in Arabidopsis.
AtELF3β disrupts the interaction between AtELF3α and LUX and alleviates the repression of downstream targets of the EC
AtELF3α was reported to interact with transcription factors to regulate the transcription of target genes (Nusinow et al. 2011). We thus tested whether AtELF3β might also affect the transcript levels of AtELF3α target genes. Accordingly, we carried out a transcriptomic analysis using the elf3-3 mutant and its isogenic wild-type Ws, as well as Col-0, AtELF3α-OE, and AtELF3β-OE, to identify differentially expressed genes (Supplemental Dataset S1). We identified 429 upregulated genes (at least 2-fold increase, P < 0.05) in AtELF3β-OE lines relative to the nontransformed control plants. Of these, 63 genes were also upregulated in the elf3-3 mutant compared to its wild-type, Ws (Fig. 6A), and 5 of the 63 genes were downregulated in the AtELF3α-OE plants (Fig. 6A). Strikingly, four of these five genes, GI (Mizoguchi et al. 2005), PRR7 (Nakamichi et al. 2007), PIF4 (Araki and Komeda 1993), and BBX6 (B-BOX6) (Hassidim et al. 2009), have previously been reported to affect flowering time. To validate these results, we profiled the mRNA levels of the four candidate genes above using reverse transcription quantitative PCR (RT-qPCR), revealing that the expression of all four genes was induced in AtELF3β-OE plants but repressed in AtELF3α-OE plants (Fig. 6, B to E). In the AtELF3β knockdown lines AtELF3-Δ60 and AtELF3-Δ53, the transcript levels of the target genes PRR7, PIF4, GI, and BBX6 were relatively lower than in nontransformed control plants (Supplemental Fig. S16). In addition, PIF4 and GI showed arrhythmic patterns and elevated transcript levels in AtELF3β-OE lines during the subjective night (Fig. 7), similar to their expression pattern in elf3 mutants (Thines and Harmon 2010). These results further indicated that AtELF3β promotes flowering at least in part by suppressing the activity of AtELF3α toward its target genes.
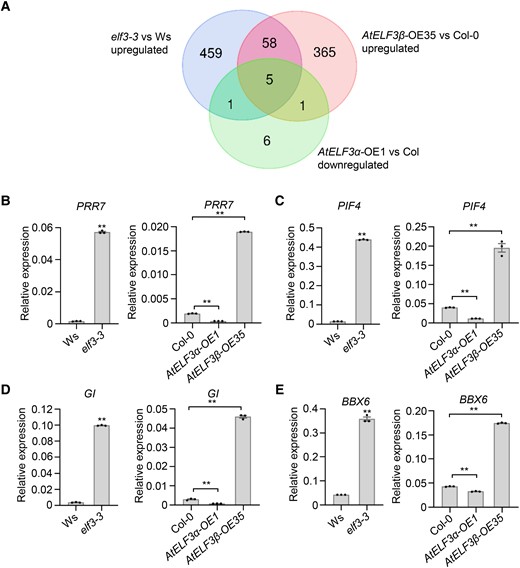
AtELF3β and AtELF3α play opposite roles in regulating the expression of PRR7, PIF4, GI, and BBX6. A) Analysis of the upregulated genes in elf3-3 and AtELF3β-OE35 plants and downregulated genes in AtELF3α-OE1 plants relative to their control plants. B)–E) RT-qPCR analysis of relative PRR7(B), PIF4(C), GI(D), and BBX6(E) transcript levels in Ws, elf3-3, Col-0, AtELF3α-OE, and AtELF3β-OE plants. Data are means ± s.e.m. (n = 3). P-values are from two-sided Student's t-tests; ** indicates P < 0.01.
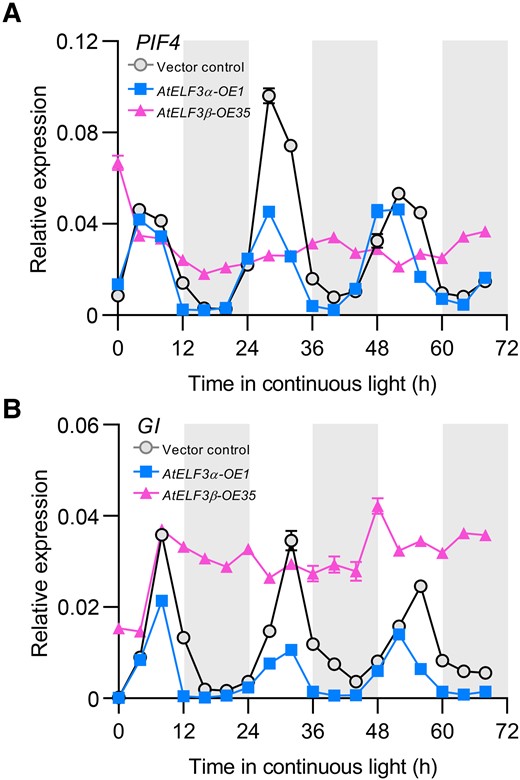
AtELF3β overexpression leads to the arrhythmic expression of PIF4 and GI. A), B) Arabidopsis seedlings were grown under 12-h-light/12-h-dark conditions for 7 d and then transferred into continuous light conditions to test the transcript levels of PIF4(A) and GI(B) in the vector control (circle), AtELF3α-OE1 (square), and AtELF3β-OE35 (triangle). PIF4 and GI have a shorter expression period in AtELF3α-OE1 compared to the vector control, while an arrhythmic expression pattern is observed in AtELF3β-OE35. Data are means ± s.e.m. (n = 3). Two independent experiments were performed, and the results were similar.
To gain a biochemical understanding of how AtELF3β regulates flowering time, we tested for a direct interaction between AtELF3α and AtELF3β in a yeast two-hybrid assay. Indeed, we determined that AtELF3α and AtELF3β can form homodimers and heterodimers (Fig. 8A). In firefly luciferase (LUC) complementation imaging (LCI) assays, we detected luminescence from the reconstitution of LUC when the cLUC-AtELF3α and AtELF3β-nLUC constructs were co-infiltrated in Nicotiana benthamiana leaves (Fig. 8B). We also performed co-immunoprecipitation (Co-IP) assays by transiently infiltrating N. benthamiana leaves with GFP-AtELF3β and AtELF3α-FLAG constructs, using the GFP and AtELF3α-FLAG pair of constructs as a negative control. GFP-AtELF3β was immunoprecipitated by the anti-FLAG antibody only from leaves co-infiltrated with GFP-AtELF3β and AtELF3α-FLAG, but not with GFP and AtELF3α-FLAG (Fig. 8C; Supplemental Fig. S17A). These observations indicate that AtELF3α physically interacts with AtELF3β.
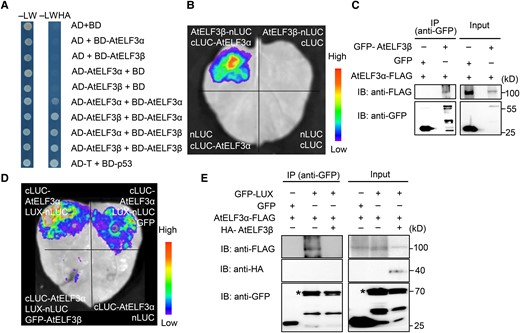
AtELF3β promotes flowering by interacting with AtELF3α to inhibit the formation of the evening complex. A) The indicated plasmids were co-transformed into yeast strain Y2HGold. Interactions between bait and prey were examined on synthetic defined (SD) medium-LW and selective medium (SD-LWHA). B) Firefly luciferase complementation imaging (LCI) assays in N. benthamiana leaves showing that AtELF3β can interact with AtELF3α. The pseudocolor bar indicates the range of luminescence intensity. C) AtELF3β interacts with and co-immunoprecipitates with AtELF3α in vivo. N. benthamiana leaves were infiltrated with 35S:GFP-AtELF3β and 35S:AtELF3α-FLAG constructs via Agrobacterium-mediated infiltration. AtELF3α-FLAG was detected in the immunoprecipitated GFP–AtELF3β complex. D) The interaction between AtELF3α and LUX decreases in the presence of AtELF3β in the LCI assays. N. benthamiana leaves were co-infiltrated with four sets of constructs at symmetric positions with equal amounts of Agrobacteria. The pseudocolor bar indicates the range of luminescence intensity. E) The interaction between AtELF3α and LUX decreases in the presence of AtELF3β in an in vivo co-immunoprecipitation. AtELF3α-FLAG was detected in the immunoprecipitated GFP–LUX complex, indicating that there is a physical association between LUX and AtELF3α in vivo. With the addition of HA-AtELF3β expression, AtELF3α-FLAG was not detected in the immunoprecipitated GFP–LUX complex. The asterisk indicates the GFP–LUX band.
ELF3α participates in the formation of the EC with ELF4 and LUX, which is then recruited to the promoter of target genes by LUX (Nusinow et al. 2011). Accordingly, the EC complex regulates the transcript levels of GI, PRR7, and PIF4. As AtELF3α and AtELF3β appeared to play opposite roles in the transcriptional regulation of GI, PRR7, and PIF4, we hypothesized that AtELF3β influenced the interaction between AtELF3α and LUX. To test this hypothesis, we employed a modified LCI assay in N. benthamiana leaves. We detected a strong luminescence signal when AtELF3α-cLUC and LUX-nLUC constructs were co-infiltrated in N. benthamiana leaves. The addition of a GFP construct during co-infiltration did not weaken the LUC activity. However, the co-infiltration of a GFP-AtELF3β construct resulted in a significant decrease in luminescence signals, indicating that AtELF3β partially disrupts the AtELF3α–LUX interaction (Fig. 8D).
We also conducted modified Co-IP assays by transiently infiltrating constructs encoding GFP-LUX, AtELF3α-FLAG, and HA-AtELF3β into N. benthamiana leaves, using the pair GFP-LUX and AtELF3α-FLAG as a positive control and the pair GFP and AtELF3α-FLAG as a negative control. In leaves expressing GFP-LUX and AtELF3α-FLAG, we detected AtELF3α-FLAG when using the anti-GFP antibody for immunoprecipitation. GFP-LUX was immunoprecipitated using the anti-GFP antibody and co-immunoprecipitated using AtELF3α-FLAG, suggesting that GFP-LUX and AtELF3α-FLAG interact in vivo. When we performed the Co-IP assay using leaves expressing GFP-LUX, AtELF3α-FLAG, and HA-AtELF3β with the anti-GFP antibody, we detected no AtELF3α-FLAG in the immunoprecipitates (Fig. 8E, Supplemental Fig. S17B). These results indicate that AtELF3β interferes with the AtELF3α–LUX interaction.
To further validate the interaction between AtELF3α and AtELF3β, we introduced the AtELF3α-OE and AtELF3β-OE constructs individually into the elf3-3 mutant to obtain elf3-3 AtELF3α-OE and elf3-3 AtELF3β-OE transgenic lines. The overexpression of AtELF3α in the elf3-3 mutant background brought the flowering time and number of rosette leaves to the level of nontransformed plants. By contrast, AtELF3β-OE had no effect on the early-flowering phenotype of elf3-3 (Supplemental Fig. S18). These results suggest that AtELF3β has no function in the absence of AtELF3α, which supports the conclusion that AtELF3β promotes flowering by blocking the function of AtELF3α.
Discussion
ELF3 has previously been reported to be an important flowering suppressor in Arabidopsis (Zagotta et al. 1996; Lu et al. 2017; Ridge et al. 2017; Bouche et al. 2021; Ronald et al. 2022); however, we report here that the ELF3 locus produces an additional transcript that promotes flower induction. The present study had three main findings. First, we showed that the two transcripts, full-length ELF3α and the shorter ELF3β, were both produced from the ELF3 locus by alternative promoter usage. Second, in contrast to ELF3α, ELF3β played a positive role in flower induction by binding to ELF3α to disturb the formation of the ELF3α–LUX complex, thus relieving the transcriptional repression of downstream target genes. Third, we identified a 58-bp sequence in the 2nd intron of PbELF3 that was closely related to the expression of PbELF3β. The absence of the 58-bp sequence was genetically associated with fewer flower buds in pear. Our results uncover a unique complexity for ELF3 in flowering regulation.
Production of ELF3β from the ELF3 locus by alternative promoter usage
Alternative promoter usage increases the complexity of the transcriptome and proteasome landscapes (Landry et al. 2003; Davuluri et al. 2008). About 30% to 50% of human (Homo sapiens) genes and about half of mouse (Mus musculus) transcripts are estimated to arise from alternative promoter usage (Baek et al. 2007). The use of variable TSSs may represent a widespread mechanism for producing spatial- and temporal-specific transcripts to regulate developmental programs and responses to environmental events: e.g. SYNAPTIC1 (SYN1) in Arabidopsis, whose encoded protein functions in chromosome condensation and pairing in meiosis (Bai et al. 1999); OsMADS1 in rice, whose encoded protein functions in floral development (Jeon et al. 2008); BLAST- AND WOUNDING-ACTIVATED MAP KINASE1 (OsBWMK1) in rice, whose encoded protein functions in the regulation of defense responses (Koo et al. 2009); and GLYCERATE 3-KINASE (GLYK) in Arabidopsis and potato (Solanum tuberosum), whose encoded proteins function in responses to changes in the light environment (Ushijima et al. 2017) and immune recognition (Gao et al. 2020), respectively. Biochemical and genetic analyses of ELF3β revealed that the alternative promoter usage mechanism also contributed to flower induction. We detected the shorter ELF3β transcript isoform in multiple species, indicating that the mechanism of alternative promoter usage at ELF3 is probably conserved in plants (Fig. 2, A and B; Fig. 3, A and B; Supplemental Fig. S9).
The second intron of ELF3 is important for ELF3β expression
Compared to the nontransformed control plants, the heterologous expression of PbELF3α in Arabidopsis resulted in delayed flowering, indicating that PbELF3α displays the similar function as Arabidopsis ELF3α (Fig. 3, F to H). Notably, the constitutive overexpression of PbELF3β and AtELF3β in Arabidopsis led to earlier flowering relative to nontransformed control plants, indicating that ELF3β and ELF3α have opposite functions in flowering regulation (Fig. 3, F to H and Fig. 4, C to E). The fewer flower buds produced by the KKJJ, KL, and LJJ pear varieties relative to the other five varieties (Fig. 1) were associated with a 58-bp deletion in the 2nd intron of PbELF3 (Fig. 1, B and C), which resulted in decreased PbELF3β transcript levels (Fig. 3D). The 58-bp deletion resulted in fewer flower buds (Fig. 1A; Supplemental Fig. S1) and co-segregated with this phenotype in BCF1 progeny (Fig. 1D). We conclude that PbELF3β likely has a major role in promoting flower bud induction. We also noticed that KKJJ, KL, and LJJ pear varieties produced fewer lateral flower buds than DS, YL, CG, JCXL, and HS, but terminal flowering seems identical between all these varieties, indicating that the mechanisms of flower bud induction in terminal and lateral sites are potentially different (Fig. 1A; Supplemental Fig. S1). In pome fruit trees, such as apple and pear, a particularly high proportion of terminal buds differentiated into floral buds (Ito et al. 1999; Costes 2003). It had been suggested that flower bud induction is associated with local hormone levels (Ito et al. 1999). Thus, the potential crosstalk between PbELF3 and hormone signaling in flower bud induction need further exploration. As the number of flower buds is a key agronomic characteristic of pear trees, the 58-bp sequence may be developed as a molecular marker to drive improvements in pear breeding.
Because of the 58-bp deletion in the 2nd intron, the expression of PbELF3β was lower in PbELF3D-HE lines compared to PbELF3N-HE lines in transgenic Arabidopsis, while the expression of PbELF3α was comparable in these lines (Supplemental Fig. S5, D and E). The expression of AtELF3β was also lower in AtELF3-Δ60 and AtELF3-Δ53 gene-edited lines harboring a partial deletion of the 2nd intron of AtELF3 (Fig. 5), suggesting that the 2nd intron is important for ELF3β expression. A sequence alignment indicated that 24 bp of the 58-bp region was conserved in Arabidopsis and pear (Supplemental Fig. S19). A cis-element analysis identified MYB transcription factor-binding sites that are shared at this region by AtELF3 and PbELF3 (Supplemental Dataset S2). This analysis provided potentially conserved regulators of ELF3β, but further research is needed to uncover the underlying mechanism.
ELF3β promotes flowering through blocking ELF3α function
Previous studies have demonstrated that ELF3α assembles the EC complex by interacting with ELF4 and LUX (Nusinow et al. 2011; Silva et al. 2020), which in turn represses the expression of the flowering time regulators GI, PRR7, and PIF4 (Mizuno et al. 2014b). The dual overexpression of AtELF3α and AtELF3β blocked the late-flowering phenotype of AtELF3α-OE plants, indicating that AtELF3β can inhibit the function of AtELF3α (Fig. 3, F to H). Our RNA-seq results established that the flowering time regulator genes GI, PRR7, PIF4, and BBX6 were upregulated in both AtELF3β-OE and elf3-3 mutant seedlings relative to their respective control plants (Fig. 6). Moreover, AtELF3β had no additive effects on the early-flowering phenotype of the elf3-3 mutant when overexpressed (Supplemental Fig. S18), further indicating that the flowering promotion activity of AtELF3β depends on the function and presence of AtELF3α. The antagonistic relationship between AtELF3α and AtELF3β is reminiscent of the key clock gene CIRCADIAN CLOCK ASSOCIATED1 (CCA1). CCA1 produces two transcript isoforms, CCA1α and CCA1β, due to alternative splicing; CCA1α physically interacts with CCA1β to disrupt the interaction between CCA1β and LATE ELONGATED HYPOCOTYL (LHY) (Seo et al. 2012). AtELF3α could form homodimers and heterodimers with AtELF3β, as demonstrated using a yeast two-hybrid assay (Fig. 8A). We confirmed the interaction between AtELF3α and AtELF3β by LCI assays (Fig. 8B) and Co-IP assays (Fig. 8C). We propose that AtELF3β is a direct modulator of AtELF3α through physical interaction. AtELF3α is a scaffold component of the EC complex, which it forms together with ELF4 and LUX (Nusinow et al. 2011). The transcript levels of GI, PRR7, and PIF4 are regulated by the EC complex (Mizuno et al. 2014b). AtELF3β disrupts the AtELF3α–LUX interaction (Fig. 8, D and E), thereby alleviating the transcriptional inhibition of GI, PRR7, and PIF4 by disrupting the assembly of the EC complex (Fig. 9).

Schematic model illustrating the role of ELF3β. As a transcriptional repressor, the evening complex is recruited to the promoters of the target genes (including GI, PRR7, PIF4, and BBX6) via LUX. Full-length ELF3α serves as a scaffold to bring together ELF4 and LUX. ELF3β inhibits the function of ELF3α by forming a nonfunctional ELF3α–ELF3β heterodimer. Consequently, elevating ELF3β expression leads to early flowering, long hypocotyls, and an arrhythmic circadian clock, which is similar to the elf3-3 mutant phenotype. Without functional ELF3α, expressing ELF3β in the elf3-3 mutant does not significantly affect its early-flowering phenotype, suggesting that the function of ELF3β depends on ELF3α.
Moreover, as an important hub protein, ELF3α also directly interacts with other factors for the assembly of protein complexes. The ELF3β isoform may thus affect other signaling pathways or developmental programs, in addition to flowering time. For example, AtELF3 could interact with PIF4 to block the transcriptional activation of its downstream target genes and regulate hypocotyl elongation in an EC-independent manner (Nieto et al. 2015). Indeed, the constitutive expression of both AtELF3β and PbELF3β promoted the elongation of hypocotyls in Arabidopsis (Supplemental Fig. S12). AtELF3 also interacts with PIF7 to repress its DNA-binding activity in the shade avoidance response (Jiang et al. 2019). In addition, we noted that the interaction between AtELF3α and PbELF3β was weaker than between AtELF3α and AtELF3β (Supplemental Fig. S20), indicating that ELF3β from various species may have different affinities for their protein partners. This result might partially explain the weaker phenotype of PbELF3β-HE (Fig. 3, F to H) compared to AtELF3β-OE in Arabidopsis (Fig. 4, F to H).
In conclusion, this work reveals a transcript, ELF3β, produced by alternative promoter usage at the ELF3 locus. The encoded protein ELF3β promotes flowering by binding to ELF3α and disturbing the formation of the EC, thus releasing the repression of EC target genes and promoting flower induction (Fig. 9). The EC integrates the signals of photoperiod and temperature for plants to adapt to seasonal changes and a fluctuating environment (Albani and Coupland 2010). It would be worth exploring how ELF3β senses and responds to changes in the external photoperiod and temperature (Jung et al. 2020). The ELF3α- and ELF3β-mediated molecular network described in this study might represent a conserved regulatory module in the control of plant development in response to ever-changing external and internal signals.
Materials and methods
Plant materials and growth conditions
The pear tissue samples were collected from the Nanjing Agricultural University Fruit Experimental Yard, Nanjing, China. All pear varieties were cultivated for more than 8 yr. Pyrus sinkiangensis cv. Kuikejuju (KKJJ), Pyrus sinkiangensis cv. Korla pear (KL), Pyrus sinkiangensis cv. Lvjuju (LJJ), Pyrus bretschneideri cv. Dangshansuli (DS), Pyrus bretschneideri cv. Yali (YL), Pyrus pyrifolia cv. Cuiguan (CG), Pyrus pyrifolia cv. Jinchuanxueli (JCXL), and Pyrus pyrifolia cv. Housui (HS) were used in this study.
The trees of BCF1 hybrid offspring population were cultivated for 5 yr. We chose pear trees that blossom on the 4th and 5th years for this study, which indicated that they had reached their reproductive adult phase. Arabidopsis seeds were surface sterilized with 75% (v/v) ethanol for 1 min and with 2.5% (w/v) sodium hypochlorite for 5 min and washed multiple times with sterilized water. The surface-sterilized seeds were sown on Murashige and Skoog (MS) medium [containing 2% (w/v) sucrose, 0.7% (w/v) agar, and pH adjusted to 5.7 with KOH] and stratified in darkness at 4 °C for 3 d. The plates were transferred into a culture chamber set to 22 °C under long-day conditions (16 h light/8 h dark). The LED light source (PAK-LED-T5-14WF-865) was provided by PAK Corporation Co. Ltd. with a correlated color temperature of 6,500 K. During plant culture, the photosynthetic photon flux density was maintained at 100 μmol m−2 s−1, with a far-red:red:blue ratio of 21:46:33. Seven-d-old seedlings were then transplanted to soil for further cultivation. The number of days required for each plant to flower was recorded, and the number of rosette leaves of Arabidopsis was counted at the time of first flower opening.
The elf3-3 mutant was obtained from the Arabidopsis Biological Resource Center (www.arabidopsis.org). The elf3-3 allele contains a single nucleotide change (GAA to TAA) that results in a premature stop codon (E281stop). The elf3-3 mutant shows an early-flowering phenotype (Hicks et al. 1996; Zagotta et al. 1996; Hicks et al. 2001).
Constructs and transformation
To generate the PbELF3N-HE and PbELF3D-HE constructs, the genomic sequences of PbELF3N and PbELF3D were amplified with primers PbELF3-GFP-F and PbELF3-GFP-R. The products were cloned into the XbaI/BamHI sites of pCAMBIA1300-35S:GFP. To generate PbELF3N−D-HE and PbELF3D+D-HE constructs, the PbELF3N and PbELF3D genes were modified by multifragment homologous recombination. The PbELF3N DNA fragment was used as template, and primers PbELF3-GFP-F/PbELF3-N-D-R1 and PbELF3-N-D-F2/PbELF3-GFP-R were used to generate PbELF3N−D. Likewise, the PbELF3D DNA fragment was used as template, and primers PbELF3-GFP-F/PbELF3-D + d-R1 and PbELF3-D + d-F2/PbELF3-GFP-R were used to obtain PbELF3D+D. The PCR products were cloned into the XbaI/BamHI sites of pCAMBIA1300-35S:GFP.
To generate PbELF3α-HE and PbELF3β-HE, the coding sequences of PbELF3α and PbELF3β were amplified with primers PbELF3α-GFP-F/PbELF3α-GFP-R and PbELF3β-GFP-F/PbELF3β-GFP-R, respectively. The products were cloned into the XbaI/BamHI sites of pCAMBIA1300-35S:GFP.
To generate AtELF3α-OE and AtELF3β-OE, the coding sequences of AtELF3α and AtELF3β were amplified with primers AtELF3α-GFP-F/AtELF3α-GFP-R and AtELF3β-GFP-F/AtELF3β-GFP-R, respectively. The products were cloned into the XbaI/BamHI sites of pCAMBIA1300-35S:GFP.
To generate AtELF3-full and AtELF3-Δintron, the full-length genomic sequence of the AtELF3 locus was amplified with primers gAtELF3α-107F(XbaI) and gAtELF3α-107R(AscI) with 2,969 bp of native promoter and cloned into pMDC107. The 1,080-bp region in the 2nd intron of AtELF3 was deleted by recombining the 5′-terminal and 3′-ternimal sequences with the ClonExpress MultiS One Step Cloning Kit (Vazyme, Nanjing, China). The full-length sequence of the 2nd intron of AtELF3 is 1,180 bp; thus, the deletion of 1,080 bp leaves 50 bp at both the 5′ and 3′ ends of the 2nd intron for splicing and AtELF3β PCR analysis.
To delete the regulatory region in the 2nd intron of AtELE3 efficiently, the esgRNA scaffold and Cas9 cassette were assembled into the pCAMBIA1300 backbone, driven by the AtU6 and RPS5A promoters, respectively, generating pH-RPS5A-Cas9 (Xing et al. 2014; Li et al., 2018). A pair of single-guide RNAs (sgRNA1 and sgRNA4) were then designed in PAM-in orientation, with another pair (sgRNA5 and sgRNA6) in PAM-out orientation. To simplify cloning steps of the paired sgRNAs, an esgRNA-AtU6 module was also generated as a PCR template. Each sgRNA was cloned at the 5′ end and 3′ end of esgRNA-AtU6 by PCR, and then the PCR products were assembled into pH-RPS5A-Cas9 by Golden Gate cloning. The fragment deletion vector was transformed into Agrobacterium (Agrobacterium tumefaciens) strain GV3101 and used to transform Arabidopsis accession Col-0 by the floral dip method (Clough and Ben 1998). The transformants were selected on solid half-strength MS medium containing hygromycin (30 mg mL−1). Homozygous mutants in the T1 generation were screened based on hygromycin resistance and identified by Sanger sequencing. Homozygous and Cas9-free T2 generation mutants were used for analysis. All primers are listed in Supplemental Dataset S3.
Microscope imaging
Expression vector (pCAMBIA1300-35S:GFP) containing genes fused with the GFP gene was transformed into Agrobacterium strain GV3101, which was used for Arabidopsis transformation. Confocal microscopy images were captured from roots of transformed seedlings. Seedlings were stained with DAPI (4′,6-diamidino-2-phenylindole) to label nucleus as marker. Images were acquired with a ZEISS LSM800 confocal microscope in multichannel mode. GFP was excited with a 488 nm laser, and fluorescence emission was detected at 495 to 530 nm. DAPI was excited with a 405 nm laser, and fluorescence emission was detected at 420 to 480 nm.
RNA isolation and RT-qPCR analysis
For single time points, the samples were collected at Zeitgeber time 16 (ZT16, with ZT being lights on) under long-day conditions (16 h light/8 h dark). For Arabidopsis, about 100 mg of 7-d-old seedlings was harvested and frozen in liquid nitrogen. For pear trees in the experimental field, at least six leaves each from three individual trees were harvested at dusk. For pear plants in the laboratory, plants were cultivated in long-day conditions (16 h light/ 8 h dark) for at least 1 mo in the chamber, and at least six leaves were collected at ZT16 as the bulk sample. Plant samples were frozen in liquid nitrogen and stored in −80 °C.
Total RNA was extracted from pear tissues and Arabidopsis samples using a polysaccharide polyphenol plant TOTAL RNA extraction kit (Foregene, Chengdu, China). Total RNA samples were treated with RNase-free DNase I (NEB, Ipswich, MA, USA) to remove contaminating genomic DNA. First-strand cDNA was synthesized from 3 μg total RNA using reverse transcription with a RevertAid First Strand cDNA Synthesis Kit (Thermo Fisher Scientific, Waltham, MA, USA). Quantitative PCR was performed to quantify the cDNA using 2× SYBR Green Master Mix (Roche, Rotkreuz, Switzerland) on a LightCycler 480 Instrument II (Roche, Rotkreuz, Switzerland). PbUBQ (XM_009359822.3, Wang et al. 2017) and AtACT (NM_121018.4, Cao et al. 2008) were used as the internal reference genes for pear and Arabidopsis, respectively. Expression values were calculated using the cycle threshold method. Three technical replicates and at least two biological replicates were performed for each sample.
Rapid amplification of cDNA ends
Total RNA was extracted from pear and Arabidopsis leaves using the method described above and treated with DNase I for RACE analysis. First-strand cDNA was synthesized using reverse transcription with a RevertAid First Strand cDNA Synthesis Kit (Thermo Fisher Scientific). The sequences for the 5′ and 3′ ends of the transcripts were obtained using a SMARTer RACE 5′/3′ Kit (Takara Bio, Kusatsu, Japan). The gene-specific primer for cloning ELF3β was based on a known sequence in the transcript identified in a PCR test. For PbELF3β, the primer was designed based on the 58-bp D region. The resulting PCR products were separated using agarose gel electrophoresis and sent for Sanger sequencing. The sequences of PbELF3β (OM640140) and AtELF3β (OM640139) are available in the NCBI GenBank.
Transcriptome deep sequencing (RNA-seq) and expression analysis
Total RNA was extracted from Ws, elf3-3 (in the Ws background), Col-0, AtELF3α-OE1, and AtELF3β-OE35 (in the Col-0 background) seedlings as described above, with three independent replicates. RNA-seq library preparation was performed using a VAHTS mRNA-seq V2 Library Prep Kit (Nanjing Vazyme Biotech, Nanjing, China) according to the manufacturer's instructions. First-strand cDNA was reverse-transcribed from total RNA and amplified. An Illumina Novaseq 6000 instrument (San Diego, CA, USA) was used to perform paired-end sequencing (PE150). Clean reads were obtained using fastp software (https://github.com/OpenGene/fastp) and were aligned to the pear reference genome (http://www.peargenome.njau.edu.cn) with HiSat2 (http://daehwankimlab.github.io/hisat2/). Gene transcript levels were calculated based on raw counts and normalized using DESeq2 (Love et al. 2014).
Yeast two-hybrid assay
The full-length coding sequences of AtELF3α and AtELF3β were cloned into pGBKT7 and pGADT7 vectors, respectively. Pairs of plasmids were transformed into the yeast strain AH109 using the LiAC-PEG method. Positive transformants were selected on synthetic defined (SD) medium lacking Leu and Trp (SD-LW), and protein interaction was tested on SD medium lacking His, Ade, Leu, and Trp (SD-LWHA).
Firefly LUC complementation assay
The full-length coding sequence of AtELF3α was cloned into the pCAMBIA1300-cLUC vector. The coding sequences of AtELF3β and LUX were cloned into the pCAMBIA1300-nLUC vector. Primers used for vector construction are listed in Supplemental Dataset S3. N. benthamiana leaves were infiltrated with the appropriate pairs of Agrobacterium cultures (strain GV3101), each carrying one plasmid. The plants were cultured for 48 h under long-day conditions (16 h light/8 h dark) at 22 °C before spraying infiltrated leaves with 0.5 mM D-luciferin. Luciferase activity was captured with a low-light, cooled, charge-coupled device imaging apparatus (Berthold Technologies, Bad Wildbad, Germany) with Indigo software (Chen et al. 2008).
In vivo co-immunoprecipitation
The coding sequence of AtELF3α was cloned into pW1211 to generate 35S:AtELF3α-FLAG. The coding sequence of AtELF3β was cloned into pMDC43 to generate 35S:GFP-AtELF3β. The primers used for construction are listed in Supplemental Dataset S3. N. benthamiana leaves were infiltrated with Agrobacterium cultures (strain GV3101) harboring each resulting plasmid. After 48 h, total proteins were extracted from the infiltrated leaves and incubated with GFP-Trap-A to immobilize GFP-AtELF3β or GFP (negative control). The immunoprecipitates were probed by immunoblotting with anti-GFP (Abmart, Berkeley Heights, NJ, USA) and anti-FLAG (MBL, Woburn, MA, USA) antibodies.
Statistical analysis
Two-group comparisons were analyzed using Student's t-test, while multiple samples were analyzed using one-way ANOVA followed by Duncan's test to determine significant differences between groups. The details of statistical analysis are provided as Supplemental Dataset S4.
Accession numbers
RNA-seq data from this publication are available from the National Genomics Data Center (https://ngdc.cncb.ac.cn/gsa/browse/CRA006204; CRA006204). Sequences of PbELF3β (OM640140) and AtELF3β (OM640139) are available at NCBI.
Acknowledgments
We thank Drs. Suomeng Dong, Jiafu Jiang, and Xiao Wu for their critical reading of and comments on the manuscript, and the Arabidopsis Biological Resource Centre for seeds. We also thank Plant Editors for English language editing.
Author contributions
J.Y.W. and P.W. designed the research; P.W., Y.L., Z.L., X.H.L., and W.Y.Z. performed the experiments; P.W., Y.L., Z.L., Y.C.W., and W.J.L. analyzed the data; X.L. and Y.T.W. created the backcrossed F1 hybrid population; J.J. H. and C. L. designed and constructed the CRISPR plasmids; and J.Y.W., P.W., Y.L., Z.L., C.Q.W., Y.C.W., S.L., T.T.G., D.Q.X., C.T., and S.L.Z. wrote and revised the paper.
Supplemental data
The following materials are available in the online version of this article.
Supplemental Figure S1. Number of flower buds per branch of the eight pear varieties analyzed in this study.
Supplemental Figure S2. Genotyping of KL, XH, YLX, and BCF1 pear trees.
Supplemental Figure S3. Longitudinal sections of leaf buds and flower buds in pear.
Supplemental Figure S4. Number of flower buds per branch of KL, XH, YLX, and the hybrid offspring population in 2022.
Supplemental Figure S5. PbELF3D causes a later flowering time than PbELF3N when heterologously expressed in Arabidopsis.
Supplemental Figure S6. Transcript levels of PbELF3α and PbELF3β in pear.
Supplemental Figure S7. Identification of PbELF3α and PbELF3β overexpression lines.
Supplemental Figure S8. Transcript levels of AtELF3α and AtELF3β in Arabidopsis.
Supplemental Figure S9. Identification of ELF3β homologous transcripts in soybean, apple, and tomato.
Supplemental Figure S10. Identification of AtbELF3α and AtELF3β overexpression lines.
Supplemental Figure S11. Subcellular localization of AtELF3α-GFP and AtELF3β-GFP proteins in the root tip cells of transgenic Arabidopsis lines.
Supplemental Figure S12. ELF3β is involved in hypocotyl elongation.
Supplemental Figure S13. Expression levels of AtbELF3α and AtELF3β in the AtELF3α-OE1 AtELF3β-OE35 co-expression line.
Supplemental Figure S14. PbELF3β-HE suppresses the later-flowering phenotype of PbELF3α-HE.
Supplemental Figure S15. AtELF3-Δintron confers a late-flowering phenotype in the elf3-3 background.
Supplemental Figure S16. Transcript level of PRR7, PIF4, GI, and BBX6 in AtELF3-Δ60 and AtELF3-Δ53.
Supplemental Figure S17. Raw data of the immunoprecipitation experiment.
Supplemental Figure S18. The overexpression of AtELF3β does not promote flowering in elf3-3.
Supplemental Figure S19. Sequence alignment of the 2nd intron from Arabidopsis and Pyrus bretschneideri.
Supplemental Figure S20. Interaction between AtELF3α-AtELF3β and AtELF3α-PbELF3β in yeast.
Supplemental Dataset S1. Differential gene expression analysis in Ws, elf3-3, Col-0, AtELF3α-OE, and AtELF3β-OE plants.
Supplemental Dataset S2. Transcription factor-binding site prediction of the 2nd intron from Arabidopsis and pear.
Supplemental Dataset S3. Primers used in this study.
Supplemental Dataset S4. Details of statistical analysis in this study.
Supplemental File S1. Pairwise alignment of PbELF3N and PbELF3D genomic DNA sequences.
Supplemental File S2. Genomic DNA sequence of AtELF3.
Funding
This work was supported by the National Key Research and Development Program of China (2022YFD1200503, 2022YFF1003100-02, and 2020YFE0202900), National Natural Science Foundation of China (32172543 and 32202457), Jiangsu Agriculture Science and Technology Innovation Fund (CX(22)3044), Natural Science Foundation of Jiangsu Province (BK20221012), the Earmarked Fund for China Agriculture Research System (CARS-28), and the Project Funded by the Priority Academic Program Development of Jiangsu Higher Education Institutions. The bioinformatic analysis was supported by the Bioinformatics Center of Nanjing Agricultural University.
References
Author notes
These authors contributed equally.
The author(s) responsible for distribution of materials integral to the findings presented in this article in accordance with the policy described in the Instructions for Authors (https://dbpia.nl.go.kr/plcell/) is: Juyou Wu ([email protected]).
Conflict of interest statement. None declared.