-
PDF
- Split View
-
Views
-
Cite
Cite
Yvonne Joho, Vanessa Vongsouthi, Chloe Gomez, Joachim S Larsen, Albert Ardevol, Colin J Jackson, Improving plastic degrading enzymes via directed evolution, Protein Engineering, Design and Selection, Volume 37, 2024, gzae009, https://doi.org/10.1093/protein/gzae009
- Share Icon Share
Abstract
Plastic degrading enzymes have immense potential for use in industrial applications. Protein engineering efforts over the last decade have resulted in considerable enhancement of many properties of these enzymes. Directed evolution, a protein engineering approach that mimics the natural process of evolution in a laboratory, has been particularly useful in overcoming some of the challenges of structure-based protein engineering. For example, directed evolution has been used to improve the catalytic activity and thermostability of polyethylene terephthalate (PET)-degrading enzymes, although its use for the improvement of other desirable properties, such as solvent tolerance, has been less studied. In this review, we aim to identify some of the knowledge gaps and current challenges, and highlight recent studies related to the directed evolution of plastic-degrading enzymes.
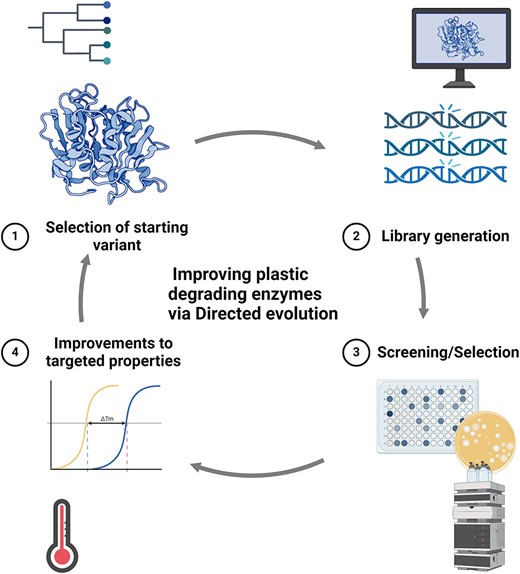
Developments in the field of enzymatic plastic degradation
The increasing amount of plastic waste has become a global issue and enzymatic plastic recycling could facilitate the transition to a circular plastic economy. Economic studies have highlighted the potential benefits of using enzymatic recycling in industrial applications (Singh et al. 2021). Nonetheless, naturally occurring PET-degrading enzymes are generally not suitable for such purposes, as they require improvement to both stability and catalytic efficiency. Rational protein engineering has been successfully applied to design enhanced PET-hydrolysing enzymes previously (Son et al. 2019, Cui et al. 2021, Jerves et al. 2024). For example, Tournier et al. achieved over 90% PET depolymerisation in 10hours with an improved variant of leaf-branch compost cutinase (LCC) (Tournier et al. 2020).
Directed evolution can complement rational approaches by screening a large number of randomly, or semi-randomly generated variants to enhance various enzyme properties, extending beyond catalytic activity and thermal stability. However, the lack of effective high-throughput screening methods has been the main obstacle to applying directed evolution to plastic-degrading enzymes (Zhu et al. 2021). Recent efforts have made significant advances in overcoming those challenges, particularly in the field of PET-degrading enzymes. However, similar advancements for evolving enzymes that degrade other prominent plastics, such as polyurethane (PU) and polyamides, remain relatively limited.
This review provides an overview of the current research and emerging trends in applying directed evolution to plastic degrading enzymes and will address outstanding engineering challenges for plastic-degrading enzymes. The review provides a holistic overview of the field, evaluating recent directed evolution studies of plastic hydrolases, and discusses opportunities, gaps, and future approaches in the following sections: (i) limitations of current plastic-degrading enzymes; (ii) searching for the optimal starting point for directed evolution; (iii) successful directed evolution of plastic degrading enzymes; (iv) advances in methods that will accelerate directed evolution of plastic-degrading enzymes; and (v) future perspectives.
Limitations to current plastic-degrading enzyme engineering
The potential for utilising enzymes in plastic recycling has started to emerge over the last decade. In 2005, cutinases were the first enzymes discovered to hydrolyse PET (Müller et al. 2005). To date, the most active and industrially suitable enzyme for PET degradation is an engineered variant of a cutinase isolated from a leaf-branch compost metagenome (LCC) in 2012 (Sulaiman et al. 2012). Additional enzymes with PETase activity have been discovered since, including a PETase recently isolated from the bacterium Ideonella sakaiensis (IsPETase) (Yoshida et al. 2016). For the consideration of such enzymes in industrial applications, it is essential to assess crucial parameters that affect the scalability of the enzymatic PET degradation process. These parameters include: i) PET crystallinity, ii) surface exchange, iii) reaction temperature, iv) enzymatic efficiency, v) PET concentration, vi) PET depolymerisation yield, vii) product composition, and viii) enzyme expression yields (Arnal et al. 2023). Considering these parameters, the scalability of engineered variants of IsPETase (FAST-PETase and HotPETase) is limited by the comparatively low depolymerisation rates compared to that observed for an engineered variant of LCC (LCCICCG) that converts PET into the monomers terephthalic acid (TPA) and ethylene glycol (EG) to 98% completion in 24 hours, or PES-H1L92F/Q94Y, a variant of a thermophilic PET hydrolase derived from a compost metagenome that converts PET to 80% completion in the same time frame (Arnal et al. 2023). Despite these promising candidates, further engineering of existing enzymes is required to enhance their scalability and efficiency for industrial use.
Although rational protein engineering, sometimes in combination with directed evolution, has been successfully applied to PET hydrolases (PETases) (Fig. 1), there is still work to be done to improve these enzymes further towards industrially applicable enzymes. Notably, most research efforts have predominantly focused on enhancing their enzymatic activity and thermal stability, while aspects such as protein solubility, substrate specificity, pH adaptation, and solvent tolerance have received limited attention. Additionally, concerns have arisen regarding issues of (enzyme) concentration dependence (Avilan et al. 2023), or product inhibition (Wei et al. 2016), and the selectivity of certain enzymes, which mainly target amorphous rather than highly crystalline components when used for post-consumer plastics (Mueller 2006).
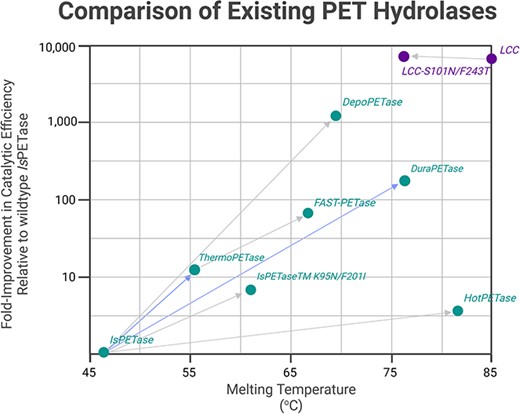
The arrows show the fold-improvement in catalytic activity (compared to the wildtype sequence unless otherwise stated) and melting temperature changes of some engineered PET hydrolases from directed evolution experiments - HotPETase (Bell et al. 2022), DepoPETase (Shi et al. 2023), TS-ΔIsPETase (Pirillo et al. 2022), SR-PETase (Zurier and Goddard, 2023), IsPETaseTMK95N/F201I (Brott et al. 2021), FAST-PETase (ML approach) (Lu et al. 2022), LCC-S101N/F243T(Pirillo et al. 2023)
Rational protein engineering faces limitations due to its low experimental throughput of engineered variants and often high failure rate. It often focuses on residues that are near the catalytic or binding site, while neglecting distal mutations, despite their potential in protein engineering (Wilding et al. 2019). For PETase engineering, rational design is hindered by the absence of any crystallographic structure with a fully occupied PET-substrate binding site (Chen et al. 2018), although short PET analogues such as 1-(2-hydroxyethyl) 4-methyl terephthalate, HEMT) and p-nitrophenol (pNP) have been successfully co-crystallized with a catalytically inactive mutant of PETase from Ideonella sakaiensis (IsPETase) (Han et al. 2017). To overcome this, computational docking studies have been employed to model PET substrate-binding modes (Austin et al. 2018, Son et al. 2019, Jerves et al. 2021), resulting in multiple binding modes being observed (Pfaff et al. 2022). Joo et al. proposed a PET degradation mechanism based on a docking study using 2-hydroxyethyl-(mono-hydroxyethyl terephthalate)4 2-HE(MHET)4 (Joo et al. 2018). However, this has been critiqued by Wei et al. who argued that the stiff polymer chain can hardly fit into the suggested docking conformation of the distal subsites IIb and IIc (Wei et al. 2019, Seo et al. 2019).
Notably, caution must be taken in interpreting the results of docking studies as the structures of short substrates bound to PETases do not represent the interaction of PETases with an extended PET chain and molecular dynamic simulations continue to give further insights in the molecular mechanism (Aboelnga and Kalyaanamoorthy 2022, Falkenstein et al. 2023).
Directed evolution (DE) is often applied to engineer proteins in a high throughput manner, bypassing the need for detailed structural information. However, structural data and mechanism-guided information can be instrumental in guiding mutagenesis in semi-rational approaches (Sun et al. 2019, Li et al. 2023). In a directed evolution experiment, a selective pressure is applied to a protein of interest with the aim to evolve beneficial properties. It has been used extensively for enhancing the activity, thermostability, solubility, solvent tolerance, and pH adaptation of biocatalysts with interest for industrial applications (Sanchez and Ting 2020, Wang et al. 2021, Wang et al. 2023). It is not within the scope of this review to explore all the uses of DE in enzymology; rather, we have focused on PET-degrading enzymes. Broad reviews of the field can be found elsewhere (Zeymer and Hilvert 2018, Packer and Liu 2015, Wang et al. 2021). A classical DE experiment generally consists of 1) selection of a starting variant, 2) mutagenesis of the gene of interest to create a variant library, and 3) screening or selection for protein properties of interest, and 4) identification of variants that exhibit improvements to the targeted properties (Fig. 2).
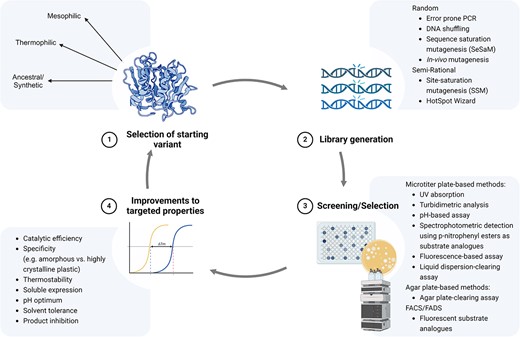
Overview of a directed evolution experiment for plastic-degrading enzymes consisting of 1) selection of a starting variant, 2) library generation, 3) screening or selection, and 4) improvements to the targeted properties of the enzyme
The application of directed evolution to PET-degrading enzymes, however, faces specific challenges. One of the main hurdles is the necessity for efficient high-throughput screening methods, a requirement that becomes particularly demanding due to the complex and insoluble nature of plastics (Zhu et al. 2021). These characteristics of plastics often lead to precipitation and heterogeneous solutions, creating difficult assay conditions for effective screening (Pirillo et al. 2021). However, there have been significant advancements in selection and screening methods, which are crucial for overcoming these challenges, as will be discussed in the subsequent sections.
Methods of screening and selection for plastic-degrading enzymes
Identifying a suitable screening or selection method is critical to the successful directed evolution of plastic-degrading enzymes. Screening-based methods analyse every enzyme for a biophysical or biochemical property of interest, whereas selection-based methods aim to extract only the variants that are improved within a library, often by linking traits to survival or sequence recovery rates. Recently, studies have explored higher throughput options for plastic-degrading enzymes (Wei et al. 2022). Fig. 3 shows an overview of the screening and selecting methods based on increased order of throughput.
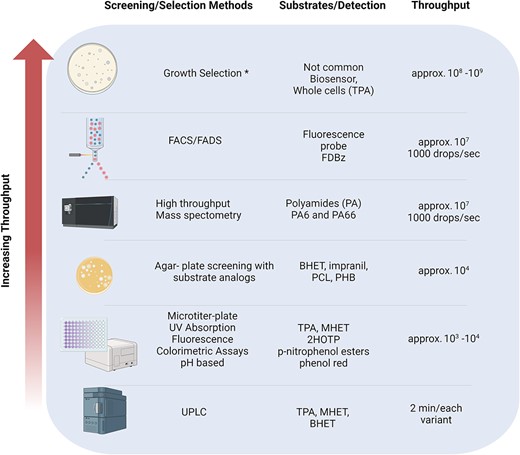
Overview of screening and selecting methods applied to the engineering of plastic degrading enzymes. Growth selection is a method commonly used within directed evolution experiments and has been used to assess plastic degrading activity of microorganisms such as I. sakaiensis (Yoshida et al. 2016) but has not yet been extensively used in engineering of PETases
High-performance liquid chromatography (HPLC) is a cornerstone in identifying PET degradation products, essential for understanding polymer hydrolysis (Pirillo et al. 2021). However, its low throughput limits its utility in directed evolution (DE) studies. Ultra-high-performance liquid chromatography (UPLC) addresses this by offering a faster alternative, as demonstrated by Bell et al. (2022), who measured the catalytic activity of PET-degrading enzymes in just two minutes per measurement, analysing over 2000 variants in two days. While UPLC is advantageous for detailed post-screening analysis, its throughput is still considered low for initial DE screenings.
UV absorption-based assays, adaptable to microtiter plates, represent a leap towards higher throughput, enabling enzyme kinetics monitoring efficiently (Zhong-Johnson et al. 2021). Microtiter plate-based assays have evolved to include a variety of techniques such as UV absorption, colorimetric, turbidimetric, titrimetric and fluorometric assays (Zhong-Johnson et al. 2021, Pfaff et al. 2021, Belisário-Ferrari et al. 2019, Pirillo et al. 2022), often utilising soluble substrate analogues for simplicity. The introduction of natural polyester substrates like PET and bis(2-hydroxylethyl) terephthalate) BHET has expanded these assays’ scope, despite challenges related to their insolubility. Notably, a colorimetric pH-based assay has been developed for PET nanoparticles and BHET degradation, marking a significant advancement in DE experiments on enzymes such as IsPETase and LCC cutinases (Pirillo et al. 2022).
Agar plate-based screening methods offer a high-throughput alternative for identifying plastic-degrading enzymes. These assays utilise substrates such as Impranil® DLN, a polyester-polyurethane dispersion which turns agar opaque and, upon hydrolysis by polyester hydrolases, forms a clear halo around the colony, indicating polyester degradation. This method’s simplicity and adaptability to various insoluble polyester substrates, like BHET, polycaprolactone (PCL), and polyhydroxybutyrate (PHB), make it invaluable for screening a wide range of enzymes (Wang et al. 2022, Brott et al. 2021, Molitor et al. 2020).
A recent study utilised high throughput mass spectrometry to screen for the enzymatic hydrolysis of polyamide (PA) enzymes using an immediate drop on demand technology (I.DOT) liquid handling systems coupled with open-port sampling interface-mass spectrometry (OPSI-MS) for high-throughput screening (8–20 sec/sample) of PA6 and PA66 hydrolysis by 6-aminohexanoate-oligomer endo-hydrolase (nylon hydrolase, NylC) (Cahill et al. 2023).
Alternatively, fluorescence-based screening methods such as fluorescent activated cell sorting (FACS) and droplet sorting (FADS) significantly enhance the throughput of directed evolution studies. These methods utilise fluorescent probes to link enzyme activity to a selectable phenotype in large libraries, facilitating the rapid identification of variants with improved activity (Alma’abadi et al. 2022, Mo et al. 2018). For example, a FADS pipeline by Qiao et al. (2022) utilised fluorescein dibenzoate (FDBz) for high-throughput screening of PET-degrading microorganisms and enzymes, showcasing the method’s potential for discovering novel PETases.
Whole-cell biosensors that emit fluorescence signals upon detecting specific analytes have been developed, suitable for FACS-based selection. For instance, Bayer et al. (2022) created a whole-cell biosensor for detecting terephthalic acid (TPA) in vivo, indicating the presence of TPA by increased bioluminescence. Similarly, Li et al. (2022) engineered a transcription factor to bind TPA and initiate the expression of a fluorescent reporter, demonstrating the potential for developing biosensors for a range of plastics.
In the context of expanding the toolkit for directed evolution, it’s noteworthy that growth selection techniques, although less commonly used due to their specificity for certain enzymes, have seen innovative applications (Molina et al. 2022). These methods, which directly link the growth of an organism to the activity of interest, represent a promising avenue for future exploration in DE studies of plastic-degrading enzymes.
Overall, the advances in high throughput techniques for especially PET-degrading enzymes has been immense. However, further methods easily applicable for various polyesters such as polyamides and polyurethanes need to be acquired to develop high throughput methods. The performance of current leading enzymes in PET degradation raises questions about the necessity for ultrahigh-throughput screening because they may have limited effectiveness in enhancing the activity of an already highly active protein. For example, the activity of the PES-H1L92F/Q94Y variant showed an increase by about two-fold compared to LCCICCG (Pfaff et al. 2022).
Identifying the best starting points for directed evolution of plastic-degrading enzymes
The rapid discovery of potential plastic-degrading enzymes presents a challenge in selecting the optimal starting point for directed evolution (DE) experiments. The question of what constitutes the best initial enzyme for a successful DE experiment has been raised (Trudeau and Tawfik 2019), highlighting the importance of protein evolvability, which depends on a protein’s tolerance to mutations and its capacity to develop new functions (Tokuriki and Tawfik 2009). Existing PET-degrading enzymes are generally categorised as either thermophilic or mesophilic, with thermostable cutinases, such as LCC, being considered more industrially relevant (Arnal et al. 2023) and thus likely providing a better starting point for DE experiments. Ancestral enzymes, reconstructed through ancestral sequence reconstruction (ASR), offer another promising avenue due to their robustness and inherent evolvability (Spence et al. 2021). This approach, combining ancestral starting points with DE, remains largely unexplored in the realm of plastic-degrading enzymes. Given that ancestral proteins have shown higher promiscuity than their modern counterparts, utilising ancestral starting points for DE could potentially evolve promiscuous ancestors towards novel specificity for various plastics. An emerging method to identify suitable PET-degrading enzymes for DE leverages bioinformatics and machine learning. A recent study applied these techniques to mine 74 putative thermotolerant PET hydrolases from natural diversity, successfully identifying and characterising enzymes from seven distinct phylogenetic groups with PET hydrolysis activity (Erickson et al. 2022). This approach not only expands the diversity of thermotolerant scaffolds for enzymatic PET deconstruction but also highlights the potential of bioinformatics and machine learning in uncovering novel biocatalysts.
Directed evolution: Pioneering advances in plastic-degrading enzymes
Directed evolution (DE) studies of plastic-degrading enzymes has had a particular focus on enhancing thermostability and catalytic activity (Table 1). These efforts have yielded remarkable successes, as evidenced by several pioneering studies. For example, in a directed evolution study on IsPETase, Brott et al. (2021) screened 49 000 clones through a plate-clearing assay and identified a variant, IsPETase K95N/F201I. This variant exhibited a 5°C increase in melting temperature and a 6°C shift in optimal temperature, showcasing DE’s capability to significantly improve enzyme stability for industrial applications. In another study, Shi et al. (2023) screened a library of 10 000 clones generated by error-prone PCR, identifying ‘DepoPETase,’ a variant that not only exhibited a 23.3°C higher melting temperature but also demonstrated a 1407-fold increase in product formation compared to the wild type. This variant, incorporating mutations T88I/D186H/D220N/N233K/N246D/R260Y/S290P, highlighted the effectiveness of iterative rounds of DE in significantly improving enzyme performance. Interestingly, the D186H, N246D and N233K were noted in previous engineering studies with IsPETase (Son et al. 2019, Son et al. 2020, Lu et al. 2022). Finally, Wang et al. used IsPETase and SbPETase (PET hydrolase from Schlegelella brevitalea sp. nov.) as starting genes for DNA shuffling and established a high-throughput screening method using a signal peptide PelB and colicin release protein Kil for PETase secretion and identified a variant, IsPETaseS139T with moderately increased activity (Wang et al. 2022).
Enzyme property . | Enzyme . | Library approach . | Substrate . | Screening/Selection Method . | Outcome . | Ref. . |
---|---|---|---|---|---|---|
PET-degrading enzymes | ||||||
Enzymatic activity | IsPETase | Error-prone PCR | FDL (fluorescein dilaurate) probed PET film | Dual fluorescence high throughput screening assay, 2850 clones | Six variants with 1.3–4.9-fold increased activity | (Liu et al. 2023) |
Enzymatic activity and stability | IsPETase | Error-prone PCR | BHET-OH | Novel BHET-OH assay, screening of 10′000 clones | DepoPETase with 1407-fold more products towards amorphous PET film and 23.3°C higher Tm value | (Shi et al. 2023) |
Enzymatic activity | IsPETas SbPETase | DNA shuffling | BHET PET film | Agar-plate based BHET, SecHTS (secretion-based) & HPLC, 1000 clones | IsPETaseS139T with increased activity (located near binding subsite II) | (Wang et al. 2022) |
Enzymatic activity | IsPETase W159H/ F238S | Site-directed mutagenesis | PET nano-particles | Colorimetric, pH-based, screening of approx. 3000 clones | W159H/F238A-ΔIsPETase possessed a higher hydrolytic activity | (Pirillo et al. 2022) |
Enzymatic activity | IsPETase | Semi-rational site-directed mutagenesis | PET fibers | YebF-mediated PETase secretion ferric iron oxidation of degradation products (TPA, MHET, BHET) creates fluorophores. | Semi-rational (SR) SR-PETase up to 7.4-fold increased enzymatic activity | (Zurier and Goddard, 2023) |
Enzymatic activity and stability | IsPETase TM plus N233C, S282C | Rational targeted site-directed mutagenesis | PET film is amorphous (6.7% cryst.) PET powder (29.8% crystallinity) | UPLC detection of TPA and MHET | HotPETase with 21 mutations and increased Tm up to 82.5°C | (Bell et al. 2022) |
Thermo- stability | IsPETase | Error-prone PCR | Impranil | 49′000 Impranil agar-plate screen | Increase of Tm by 5.0 °C IsPETaseTM K95N/F201I | (Brott et al. 2021) |
Enzymatic activity | LCC cutinase ICCG | Site-directed mutagenesis | BHET or PET nano-particles | pH-based assay with phenol red colour changes (pH 8 to 6.2) to yellow | Evolved S101N/F243T ΔLCC has increased activity on PET | (Pirillo et al. 2023) |
Enzymatic activity | LCC cutinase | Error-prone PCR | Fluorescent PET mimic conjugate | FACS: Yeast surface display platform, surface-displayed enzymes can be screened for their activity to cleave synthetic probe | Identified H218Y mutation improving binding to PET | (Cribari et al. 2023) |
Other plastic-degrading enzymes | ||||||
Enzymatic activity | PHB deploy- merase | Error-prone PCR | p-NPC4 pNCPn (n = 2–6) PHB | Surface-displayed, colorimetric p-NPC4 screen, 5000 clones | Increase activity for p-NPCs but 3.5-fold lower activity than wildtype | (Tan et al. 2013) |
Enzymatic activity | Polyamidase (Nylon- Depolymerising enzyme) | Error-prone PCR | Amorphous PA 6 film PA6,6 Granulates (Polyamide) | Detection of nylon-6, nylon-6,6 (Polyurethane product) labelling with amine-reactive chromogen MAFC to quantify degradation products (1700 clones) | Polyamidase with 1.9-fold activity (NylCTSP27Q/F301L) | (Puetz et al. 2023) |
Enzymatic activity | LCC | - | FPAP probe | FADS pipeline fluorogenic probe (FPAP) explore novel PUR-catalysts | Novel polyurethane-degrading fluorescent probe (FPAP), high throughput pipeline | (Xu et al. 2023) |
pH Adaption | Penicillium cyclopium lipase I (PCL) | Error-prone PCR | p-nitrophenyl esters | Screen of p-nitrophenyl laurate, 5100 clones | N157F variant displayed 23% more retained activity at pH 11 at 70°C. | (Huang et al. 2019) |
Enzyme property . | Enzyme . | Library approach . | Substrate . | Screening/Selection Method . | Outcome . | Ref. . |
---|---|---|---|---|---|---|
PET-degrading enzymes | ||||||
Enzymatic activity | IsPETase | Error-prone PCR | FDL (fluorescein dilaurate) probed PET film | Dual fluorescence high throughput screening assay, 2850 clones | Six variants with 1.3–4.9-fold increased activity | (Liu et al. 2023) |
Enzymatic activity and stability | IsPETase | Error-prone PCR | BHET-OH | Novel BHET-OH assay, screening of 10′000 clones | DepoPETase with 1407-fold more products towards amorphous PET film and 23.3°C higher Tm value | (Shi et al. 2023) |
Enzymatic activity | IsPETas SbPETase | DNA shuffling | BHET PET film | Agar-plate based BHET, SecHTS (secretion-based) & HPLC, 1000 clones | IsPETaseS139T with increased activity (located near binding subsite II) | (Wang et al. 2022) |
Enzymatic activity | IsPETase W159H/ F238S | Site-directed mutagenesis | PET nano-particles | Colorimetric, pH-based, screening of approx. 3000 clones | W159H/F238A-ΔIsPETase possessed a higher hydrolytic activity | (Pirillo et al. 2022) |
Enzymatic activity | IsPETase | Semi-rational site-directed mutagenesis | PET fibers | YebF-mediated PETase secretion ferric iron oxidation of degradation products (TPA, MHET, BHET) creates fluorophores. | Semi-rational (SR) SR-PETase up to 7.4-fold increased enzymatic activity | (Zurier and Goddard, 2023) |
Enzymatic activity and stability | IsPETase TM plus N233C, S282C | Rational targeted site-directed mutagenesis | PET film is amorphous (6.7% cryst.) PET powder (29.8% crystallinity) | UPLC detection of TPA and MHET | HotPETase with 21 mutations and increased Tm up to 82.5°C | (Bell et al. 2022) |
Thermo- stability | IsPETase | Error-prone PCR | Impranil | 49′000 Impranil agar-plate screen | Increase of Tm by 5.0 °C IsPETaseTM K95N/F201I | (Brott et al. 2021) |
Enzymatic activity | LCC cutinase ICCG | Site-directed mutagenesis | BHET or PET nano-particles | pH-based assay with phenol red colour changes (pH 8 to 6.2) to yellow | Evolved S101N/F243T ΔLCC has increased activity on PET | (Pirillo et al. 2023) |
Enzymatic activity | LCC cutinase | Error-prone PCR | Fluorescent PET mimic conjugate | FACS: Yeast surface display platform, surface-displayed enzymes can be screened for their activity to cleave synthetic probe | Identified H218Y mutation improving binding to PET | (Cribari et al. 2023) |
Other plastic-degrading enzymes | ||||||
Enzymatic activity | PHB deploy- merase | Error-prone PCR | p-NPC4 pNCPn (n = 2–6) PHB | Surface-displayed, colorimetric p-NPC4 screen, 5000 clones | Increase activity for p-NPCs but 3.5-fold lower activity than wildtype | (Tan et al. 2013) |
Enzymatic activity | Polyamidase (Nylon- Depolymerising enzyme) | Error-prone PCR | Amorphous PA 6 film PA6,6 Granulates (Polyamide) | Detection of nylon-6, nylon-6,6 (Polyurethane product) labelling with amine-reactive chromogen MAFC to quantify degradation products (1700 clones) | Polyamidase with 1.9-fold activity (NylCTSP27Q/F301L) | (Puetz et al. 2023) |
Enzymatic activity | LCC | - | FPAP probe | FADS pipeline fluorogenic probe (FPAP) explore novel PUR-catalysts | Novel polyurethane-degrading fluorescent probe (FPAP), high throughput pipeline | (Xu et al. 2023) |
pH Adaption | Penicillium cyclopium lipase I (PCL) | Error-prone PCR | p-nitrophenyl esters | Screen of p-nitrophenyl laurate, 5100 clones | N157F variant displayed 23% more retained activity at pH 11 at 70°C. | (Huang et al. 2019) |
Enzyme property . | Enzyme . | Library approach . | Substrate . | Screening/Selection Method . | Outcome . | Ref. . |
---|---|---|---|---|---|---|
PET-degrading enzymes | ||||||
Enzymatic activity | IsPETase | Error-prone PCR | FDL (fluorescein dilaurate) probed PET film | Dual fluorescence high throughput screening assay, 2850 clones | Six variants with 1.3–4.9-fold increased activity | (Liu et al. 2023) |
Enzymatic activity and stability | IsPETase | Error-prone PCR | BHET-OH | Novel BHET-OH assay, screening of 10′000 clones | DepoPETase with 1407-fold more products towards amorphous PET film and 23.3°C higher Tm value | (Shi et al. 2023) |
Enzymatic activity | IsPETas SbPETase | DNA shuffling | BHET PET film | Agar-plate based BHET, SecHTS (secretion-based) & HPLC, 1000 clones | IsPETaseS139T with increased activity (located near binding subsite II) | (Wang et al. 2022) |
Enzymatic activity | IsPETase W159H/ F238S | Site-directed mutagenesis | PET nano-particles | Colorimetric, pH-based, screening of approx. 3000 clones | W159H/F238A-ΔIsPETase possessed a higher hydrolytic activity | (Pirillo et al. 2022) |
Enzymatic activity | IsPETase | Semi-rational site-directed mutagenesis | PET fibers | YebF-mediated PETase secretion ferric iron oxidation of degradation products (TPA, MHET, BHET) creates fluorophores. | Semi-rational (SR) SR-PETase up to 7.4-fold increased enzymatic activity | (Zurier and Goddard, 2023) |
Enzymatic activity and stability | IsPETase TM plus N233C, S282C | Rational targeted site-directed mutagenesis | PET film is amorphous (6.7% cryst.) PET powder (29.8% crystallinity) | UPLC detection of TPA and MHET | HotPETase with 21 mutations and increased Tm up to 82.5°C | (Bell et al. 2022) |
Thermo- stability | IsPETase | Error-prone PCR | Impranil | 49′000 Impranil agar-plate screen | Increase of Tm by 5.0 °C IsPETaseTM K95N/F201I | (Brott et al. 2021) |
Enzymatic activity | LCC cutinase ICCG | Site-directed mutagenesis | BHET or PET nano-particles | pH-based assay with phenol red colour changes (pH 8 to 6.2) to yellow | Evolved S101N/F243T ΔLCC has increased activity on PET | (Pirillo et al. 2023) |
Enzymatic activity | LCC cutinase | Error-prone PCR | Fluorescent PET mimic conjugate | FACS: Yeast surface display platform, surface-displayed enzymes can be screened for their activity to cleave synthetic probe | Identified H218Y mutation improving binding to PET | (Cribari et al. 2023) |
Other plastic-degrading enzymes | ||||||
Enzymatic activity | PHB deploy- merase | Error-prone PCR | p-NPC4 pNCPn (n = 2–6) PHB | Surface-displayed, colorimetric p-NPC4 screen, 5000 clones | Increase activity for p-NPCs but 3.5-fold lower activity than wildtype | (Tan et al. 2013) |
Enzymatic activity | Polyamidase (Nylon- Depolymerising enzyme) | Error-prone PCR | Amorphous PA 6 film PA6,6 Granulates (Polyamide) | Detection of nylon-6, nylon-6,6 (Polyurethane product) labelling with amine-reactive chromogen MAFC to quantify degradation products (1700 clones) | Polyamidase with 1.9-fold activity (NylCTSP27Q/F301L) | (Puetz et al. 2023) |
Enzymatic activity | LCC | - | FPAP probe | FADS pipeline fluorogenic probe (FPAP) explore novel PUR-catalysts | Novel polyurethane-degrading fluorescent probe (FPAP), high throughput pipeline | (Xu et al. 2023) |
pH Adaption | Penicillium cyclopium lipase I (PCL) | Error-prone PCR | p-nitrophenyl esters | Screen of p-nitrophenyl laurate, 5100 clones | N157F variant displayed 23% more retained activity at pH 11 at 70°C. | (Huang et al. 2019) |
Enzyme property . | Enzyme . | Library approach . | Substrate . | Screening/Selection Method . | Outcome . | Ref. . |
---|---|---|---|---|---|---|
PET-degrading enzymes | ||||||
Enzymatic activity | IsPETase | Error-prone PCR | FDL (fluorescein dilaurate) probed PET film | Dual fluorescence high throughput screening assay, 2850 clones | Six variants with 1.3–4.9-fold increased activity | (Liu et al. 2023) |
Enzymatic activity and stability | IsPETase | Error-prone PCR | BHET-OH | Novel BHET-OH assay, screening of 10′000 clones | DepoPETase with 1407-fold more products towards amorphous PET film and 23.3°C higher Tm value | (Shi et al. 2023) |
Enzymatic activity | IsPETas SbPETase | DNA shuffling | BHET PET film | Agar-plate based BHET, SecHTS (secretion-based) & HPLC, 1000 clones | IsPETaseS139T with increased activity (located near binding subsite II) | (Wang et al. 2022) |
Enzymatic activity | IsPETase W159H/ F238S | Site-directed mutagenesis | PET nano-particles | Colorimetric, pH-based, screening of approx. 3000 clones | W159H/F238A-ΔIsPETase possessed a higher hydrolytic activity | (Pirillo et al. 2022) |
Enzymatic activity | IsPETase | Semi-rational site-directed mutagenesis | PET fibers | YebF-mediated PETase secretion ferric iron oxidation of degradation products (TPA, MHET, BHET) creates fluorophores. | Semi-rational (SR) SR-PETase up to 7.4-fold increased enzymatic activity | (Zurier and Goddard, 2023) |
Enzymatic activity and stability | IsPETase TM plus N233C, S282C | Rational targeted site-directed mutagenesis | PET film is amorphous (6.7% cryst.) PET powder (29.8% crystallinity) | UPLC detection of TPA and MHET | HotPETase with 21 mutations and increased Tm up to 82.5°C | (Bell et al. 2022) |
Thermo- stability | IsPETase | Error-prone PCR | Impranil | 49′000 Impranil agar-plate screen | Increase of Tm by 5.0 °C IsPETaseTM K95N/F201I | (Brott et al. 2021) |
Enzymatic activity | LCC cutinase ICCG | Site-directed mutagenesis | BHET or PET nano-particles | pH-based assay with phenol red colour changes (pH 8 to 6.2) to yellow | Evolved S101N/F243T ΔLCC has increased activity on PET | (Pirillo et al. 2023) |
Enzymatic activity | LCC cutinase | Error-prone PCR | Fluorescent PET mimic conjugate | FACS: Yeast surface display platform, surface-displayed enzymes can be screened for their activity to cleave synthetic probe | Identified H218Y mutation improving binding to PET | (Cribari et al. 2023) |
Other plastic-degrading enzymes | ||||||
Enzymatic activity | PHB deploy- merase | Error-prone PCR | p-NPC4 pNCPn (n = 2–6) PHB | Surface-displayed, colorimetric p-NPC4 screen, 5000 clones | Increase activity for p-NPCs but 3.5-fold lower activity than wildtype | (Tan et al. 2013) |
Enzymatic activity | Polyamidase (Nylon- Depolymerising enzyme) | Error-prone PCR | Amorphous PA 6 film PA6,6 Granulates (Polyamide) | Detection of nylon-6, nylon-6,6 (Polyurethane product) labelling with amine-reactive chromogen MAFC to quantify degradation products (1700 clones) | Polyamidase with 1.9-fold activity (NylCTSP27Q/F301L) | (Puetz et al. 2023) |
Enzymatic activity | LCC | - | FPAP probe | FADS pipeline fluorogenic probe (FPAP) explore novel PUR-catalysts | Novel polyurethane-degrading fluorescent probe (FPAP), high throughput pipeline | (Xu et al. 2023) |
pH Adaption | Penicillium cyclopium lipase I (PCL) | Error-prone PCR | p-nitrophenyl esters | Screen of p-nitrophenyl laurate, 5100 clones | N157F variant displayed 23% more retained activity at pH 11 at 70°C. | (Huang et al. 2019) |
The integration of rational and semi-rational design with directed evolution (DE) has markedly improved the development of enzymes for plastic degradation. These approaches utilise computational predictions and targeted mutagenesis to efficiently evolve enzymes with enhanced properties (Yu et al. 2022, Jochens and Bornscheuer, 2010). For instance, Bell et al. (2022) used protein stability prediction tools and NNK (N=C/T/G/A, K = G/T) degenerate primers to evolve a ‘HotPETase’ variant, achieving a significant increase in melting temperature to 82.5°C. This effort involved screening over 2000 variants using an automated, high-throughput UPLC platform, showcasing the effective combination of computational and experimental methods in enzyme evolution. Similarly, Pirillo et al. (2022, 2023) employed site-directed mutagenesis to target specific residues in IsPETase and LCC. Screening of 3000 clones of IsPETase using a colorimetric pH-based assay (phenol red) led to the identification of an enhanced IsPETase variant that had increased catalytic activity by 130% and thermostability by 4.9°C relative to wild-type (Pirillo et al. 2022). In another study, a LCC variant with increased activity on PET microplastics and post-consumer PET was identified by a similar approach, demonstrating the potential of semi-rational design in DE (Pirillo et al. 2023). These examples highlight the strategic use of semi-rational DE to design targeted libraries, however, the number of selected residues for site-directed mutagenesis needs to be balanced to maximise the likelihood of yielding beneficial mutations while remaining economically feasible to screen.
Notably, examples of directed evolution targeting plastics other than PET are very limited. In 2013, an error-prone PCR mutagenesis library aimed to evolve polyhydroxy butyrate (PHB) depolymerase from Ralstonia pickettii T1 (Tan et al. 2013). The study failed to improve the activity for PHB degradation; despite an increase in activity for p-nitrophenyl butyrate (the substrate analogue used in the screening step), the activity towards PHB was 3.5-fold lower than that of the wildtype. Another recent study on a polyamidase (nylon-depolymerizing enzyme) stands as a counterexample. In this study, 1700 variants generated via random mutagenesis were quickly screened for nylon-6 degradation, leading to the identification of an enzyme (NylCTSP27Q/F301L) that showed a 1.9-fold increase in catalytic activity (Puetz et al. 2023). However, DE studies on other polyesters are still limited because of non-existent high throughput methods.
Despite these advancements, the potential of DE extends beyond targeting catalytic activity and stability, particularly in addressing substrate specificity, enzyme solubility, and resistance to product inhibition (Fig. 4). For industrial use, a PET conversion rate exceeding 90% is preferred (Arnal et al. 2023). However, only cutinase LCCICCG has achieved this level, often requiring pre-treatment due to the target of all PET hydrolases being amorphous PET. HotPETase, was evolved from a variant that can use amorphous PET as a substrate (ThermoPETase) and over multiple evolution rounds, it not only became more thermostable (Tm = 82°C) but it can also accept semi-crystalline post-consumer PET (Bell et al. 2022). Further advances in machine learning have allowed for predictions of substrate specificity across several protein families and could provide helpful for further DE studies on the substrate scope of plastic-degrading enzymes (Goldman et al. 2022).
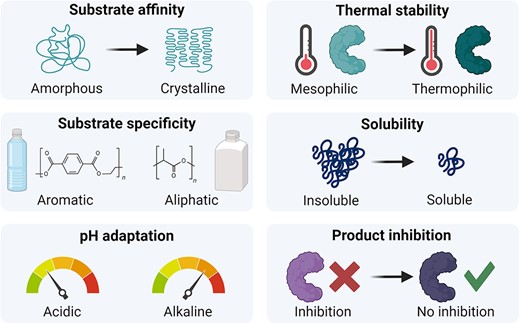
Example properties of plastic-degrading enzymes that can be engineered using directed evolution to improve current limitations
Increasing the expression levels of current plastic-degrading enzymes significantly is necessary to meet the desirable amount (>20 g/L extracellular protein) for large-scale applications (Arnal et al. 2023), which highlights the potential of directed evolution approaches. The innovative use of split-GFP screening systems, as demonstrated with DuraPETase by Weigert et al. (2021), suggests a promising avenue for enhancing soluble protein expression by DE. This method revealed a direct correlation between increased protein yield and GFP expression, indicating its potential for identifying variants with improved solubility and expression levels, which are crucial for scaling up enzyme production for industrial applications.
Efforts to minimise product inhibition or protein concentration-dependent inhibition effects in PET hydrolases have been discussed in recent reviews (Tournier et al. 2023, Zhu et al. 2021). Product inhibition concerns have been addressed by using PETase in conjugation with mono-hydroxyethyl terephthalic acid hydrolase (MHETase) and more recently bis(2-hydroxyethyl) terephthalate (BHETase) which are both enzymes produced by I. sakaiensis to break down by products of PET hydrolysis into terephthalic acid and ethylene glycol (Knott et al. 2020, Li et al. 2023). Studies have shown that using a chimera of IsPETase and MHETase can increase rates of TPA production by approximately 7.5-fold (Knott et al. 2020), and using engineered BHETase linked to IsPETase could lead to a 7-fold improvement in PET hydrolysis using a mechanism-guided approach (Li et al. 2023). Though directed evolution may not be able to directly improve these conjugative systems as a whole, it could be beneficial to shift a focus to target MHETase and BHETase to improve their properties to enhance the synergy with PETase, reducing product accumulation that inhibits the catalytic efficiency of individual components. In another approach, screening or selection of PETase-MHETase (or PETase-BHETase) chimeras, for instance where a library of MHETases is conjugated with a highly active PETase, may identify improved conjugative systems.
In summary, directed evolution has facilitated significant progress in the development of plastic-degrading enzymes, with notable advancements in thermostability and catalytic activity. However, the exploration of uncharted territories such as solubility, substrate specificity, and particularly the optimization of multi-enzyme systems to overcome product inhibition, offers promising avenues for future research. By leveraging DE to enhance the compatibility and efficiency of enzyme combinations, such as PETase and MHETase/BHETase, there is potential to significantly improve the industrial viability of enzymatic plastic degradation processes, contributing to more effective and sustainable solutions for managing plastic waste.
Concluding remarks and future perspectives
Rational protein engineering has proven to be effective in enhancing specific features such as thermostability and activity. However, its true potential is unlocked through the complementary use of directed evolution, which enables the investigation of a much larger sequence space and has recently become more feasible to use due to advances in high-throughput screening and selection methods. Despite significant progress in the applicability of directed evolution to PETases, and numerous success cases, the field still requires the development of more efficient high-throughput screening and selection methods. Methods such as FACS and FADS show significant potential for the selection of desired plastic-degrading enzymes from large libraries (Qiao et al. 2022, Xu et al. 2023, Cribari et al. 2023), hence these may be used as starting points for novel methods to address the limitations within directed evolution. Alternatively, the integration of computational methods such as machine learning or ancestral sequence reconstruction into the directed evolution workflow can enhance efficiency through in silico screening (Wittmann et al. 2021, Yang et al. 2019) and the creation of smarter, combinatorial libraries. Examples of this include the development of FAST-PETase through machine learning (Lu et al. 2022) or the development of thermostable PETases utilising ancestral reconstruction (Joho et al. 2023).
While directed evolution has been successfully applied to PETases, a recent shift towards the development of enzymes for alternative plastics is noted (Puetz et al. 2023). This is especially important if the global plastics issue is to be addressed. While it is possible to re-engineer or modify enzymes towards new plastics through traditional directed evolution workflows, machine learning emerges as a more promising solution, as algorithms assess multiple enzymes against various substrates (Goldman et al. 2022).
It is possible that we are entering a golden era for high throughput directed evolution with promising emerging in vivo screening methods (McLure et al. 2022, Zeng et al. 2020). This review mostly focused on classic directed evolution studies. Nonetheless, exciting advances have been made based on whole-cell protein sensors detecting TPA and various benzoic derivates. These advances, coupled with the directed evolution of plastic-degrading enzymes, hold the promise of moving the field towards a future where continuous evolution becomes the norm in developing more efficient enzymes (Molina et al. 2022, Bayer et al. 2022, Li et al. 2022).
Acknowledgments
The figures were created with BioRender.com.
Author contributions
Yvonne Joho (Conceptualization [Equal], Data curation [Equal], Formal analysis [Equal], Investigation [Equal], Methodology [Equal], Visualization [Equal], Writing—original draft [Equal], Writing—review & editing [Equal]), Vanessa Vongsouthi (Conceptualization [Equal], Data curation [Equal], Validation [Equal], Visualization [Equal], Writing—original draft [Equal], Writing—review & editing [Equal]), Chloe Gomez (Conceptualization [Equal], Data curation [Equal], Formal analysis [Equal], Investigation [Equal], Methodology [Equal], Visualization [Equal], Writing—original draft [Equal], Writing—review & editing [Equal]), Joachim S. Larsen (Investigation [Equal], Methodology [Equal], Writing—original draft [Equal], Writing—review & editing [Equal]), Albert Ardevol (Conceptualization [Equal], Data curation [Equal], Formal analysis [Equal], Funding acquisition [Equal], Investigation [Equal], Methodology [Equal], Project administration [Equal], Supervision [Equal], Validation [Equal], Writing—original draft [Equal], Writing—review & editing [Equal]), Colin Jackson (Conceptualization [Equal], Data curation [Equal], Formal analysis [Equal], Investigation [Equal], Methodology [Equal], Project administration [Equal], Resources [Equal], Supervision [Equal], Validation [Equal], Writing—original draft [Equal], Writing—review & editing [Equal]).
Funding
This research was partially supported by a RIoTS CoP micro-relay grant (S-00246-07) and by the Advanced Engineering Biology FSP (SynBio Novelty by Design grant) and the CSIRO Manufacturing BU.
Edited by: Dr Eva Petersen