-
PDF
- Split View
-
Views
-
Cite
Cite
Hsiang-Chia Lu, Chiao-Wen Huang, Tetsuro Mimura, Dewi Sukma, Ming-Tsair Chan, Temperature-Regulated Flowering Locus T–Like Gene Coordinates the Spike Initiation in Phalaenopsis Orchid, Plant and Cell Physiology, Volume 65, Issue 3, March 2024, Pages 405–419, https://doi.org/10.1093/pcp/pcad166
- Share Icon Share
Abstract
Phalaenopsis aphrodite can be induced to initiate spike growth and flowering by exposure to low ambient temperatures. However, the factors and mechanisms responsible for spike initiation in P. aphrodite remain largely unknown. In this study, we show that a repressor Flowing Locus T-like (FTL) gene, FTL, can act as a negative regulator of spike initiation in P. aphrodite. The mRNA transcripts of PaFTL are consistently high during high ambient temperature, thereby preventing premature spike initiation. However, during low ambient temperature, PaFTL expression falls while FT expression increases, allowing for spike initiation. Knock-down of PaFTL expression through virus-inducing gene silencing promoted spike initiation at 30/28°C. Moreover, PaFTL interacts with FLOWERING LOCUS D in a similar manner to FT to regulate downstream flowering initiation genes. Transgenic P. aphrodite plants exhibiting high expression of PaFTL do not undergo spike initiation, even when exposed to low ambient temperatures. These findings shed light on the flowering mechanisms in Phalaenopsis and provide new insights into how perennial plants govern spike initiation in response to temperature cues.
Introduction
Orchids (Orchidaceae) are incredibly diverse and make up one of the largest families of flowering plants, which are estimated to have more than 24,000 species (Zhang et al. 2018). Phalaenopsis is a well-known genus within the Orchidaceae family, with 63 species (Christenson 2001, Tsai 2011). Phalaenopsis orchids are commonly called moth orchids, and they are popularly used as ornaments in the home due to their long-lasting blooms and attractive appearance. Phalaenopsis aphrodite is a native species that originates from the southern part of Taiwan and the Philippines (Christenson 2001). It is a day-neutral perennial plant with two distinct vegetative growth stages: a long juvenile and a mature phase. During the juvenile stage, axillary buds produced from the meristem at the axil of subtending leaves remain dormant under high–ambient temperature conditions. After transitioning from the juvenile to the mature phase, the axillary buds break dormancy when temperatures fall below the ambient temperature. This phenomenon of floral initiation and the onset of reproductive development is referred to as spike initiation (Lee 1987, Blanchard and Runkle 2006, Chen et al. 2008).
The application of low ambient temperatures to induce spike initiation and flowering in Phalaenopsis has been a long-standing practice in the orchid industry (Wang et al. 2013). However, the molecular mechanisms underlying this process have not been extensively studied, and our current understanding remains rudimentary compared to that of the model plant system, Arabidopsis. Arabidopsis is a facultative long-day annual plant with a short life cycle. When Arabidopsis transitions to the reproductive phase, shoot apical meristems (SAM) convert to inflorescence meristems, and the elongating shoot subsequently produces floral organs continuously until plant death. This process differs from the separate spiking and flowering processes observed in Phalaenopsis. Furthermore, lower ambient temperatures (16°C) delay flowering compared to 23°C by upregulating the expression of Flowering Locus C in Arabidopsis, and the effect is most apparent under long-day growth conditions, suggesting the presence of an antagonistic mechanism that inhibits the photoperiod flowering pathway at lower ambient temperatures (Blazquez et al. 2003). Although the exact mechanism is still poorly understood, several genes have been implicated, including FCA, FVE (Blazquez et al. 2003), SHORT VEGETATIVE PHASE (SVP) (Lee et al. 2007), TERMINAL FLOWER 1 (TFL1), EARLY FLOWERING3 (ELF3) (Strasser et al. 2009) and FLOWERING LOCUS T (FT; (Kim et al. 2013). In addition, PHYTOCHROME INTERACTING FACTOR4 (PIF4) has been found to accelerate flowering at high ambient temperatures (Kumar et al. 2012). Importantly, both the delay and acceleration of flowering are mediated via the differential regulation of FT expression (Lee et al. 2007, Kumar et al. 2012).
The FT protein is a member of the phosphatidylethanolamine-binding protein (PEBP) family (Kardailsky et al. 1999). In Arabidopsis, FT is primarily expressed in the leaf vascular tissues, where it is then transported to the SAM to act as a floral initiation signal (Corbesier et al. 2007). At the SAM, FT directly interacts with 14-3-3 proteins and next with bZIP transcription factor FLOWERING LOCUS D (FD) to form an FD/FT/14-3-3 protein complex (Taoka et al. 2011, Collani et al. 2019). Then, the FD proteins have been identified to interact with promoters of LEAFY (LFY), SUPPRESSOR OF OVEREXPRESSION OF CONSTANS 1 (SOC1) and APETALA1 (AP1) to initiate flowering (Weigel et al. 1992, Dennis and Bowman 1993, Abe et al. 2005, Wigge et al. 2005, Yoo et al. 2005, Jaeger et al. 2013, Collani et al. 2019, Romera-Branchat et al. 2020, Zhu et al. 2020).
A hypothetical model for P. aphrodite flowering at low temperatures was proposed by Wang et al. (2019). In this model, low temperature activates GIGANTEA (Luo et al. 2011), which promotes the CONSTANS-like gene COL (Zhang et al. 2011). COL promotes FT1 in leaves (Jang et al. 2015), and the FT1 protein is then transported to the axillary buds (juvenile or mature), where it interacts with FD. In mature axillary buds, FT1 forms a complex with FD, possibly activating the downstream genes required for floral induction (Jang et al. 2015), such as AP1 (Sawettalake et al. 2017). Moreover, H1 (Knox) (Yu et al. 2000) blocks the repressor MADS1 (Yu and Goh 2000) and allows SOC1 expression that can provoke LFY expression (Jang et al. 2015). On the other hand, at low temperatures, FVE (Sun et al. 2012, Koh et al. 2018) promotes SOC1 expression (Koh et al. 2018). This model suggests that at ambient temperature, the regulated mechanism promotes the downstream flowering genes in mature axillary buds. While this model suggests that the low temperature may induce FT expression in leaves, it does not fully explain the induction of flowering solely by FT induction in leaves. In fact, we do not know when FT moves to the SAM and why flowering induction does not occur in plants before maturity.
Another member of the PEBP family, TFL1, has also been identified as a key negative regulator of flowering in plants (Shannon and Meeks-Wagner 1991). During the vegetative stage, TFL1 is weakly expressed in the lower part of the SAM, but it increases significantly following the transition to the reproductive stage in Arabidopsis (Bradley et al. 1997). The FT homology genes showed that antagonistic activity is widely conserved across various plant species (Klintenas et al. 2012) and many functional paralogs have been noted, such as BvFT2 and BvFT1 in sugar beet (Beta vulgaris) (Pin et al. 2010) and FT1 and FT2 in poplar (Populus spp.) (Hsu et al. 2011). Moreover, it has been found that homologs of both FT and TFL1 can also regulate first-time flowering in perennial plants. For example, overexpression of FT2 can induce first-time flowering within 1 year in poplar, which has a long juvenile phase lasting up to 7–10 years (Hsu et al. 2006). When the expression of TFL1 homologs in pear (Pyrus communis L.) and apple (Malus × domestica Borkh.) seedlings was reduced by RNA silencing, precocious flowering was observed (Flachowsky et al. 2012, Freiman et al. 2012). In Arabis alpina, AaTFL1 was found to repress flowering in juvenile plants exposed to vernalization by inhibiting AaLFY expression (Wang et al. 2011). Both TFL1 and FT are mobile proteins, but they exhibit different movement patterns. While FT moves from the leaves to the SAM, TFL1 appears to move within the SAM (Goretti et al. 2020).
In this study, we present functional evidence for the role of PaFTL, an FT homolog, in the low-temperature induction flowering of P. aphrodite. Our analysis revealed that PaFTL belongs to the FT-like clade, distinct from the TFL clade, and acts as a regulator of spike initiation. PaFTL expression patterns at low ambient temperatures were found to vary with different stages of plant development, allowing PaFTL to play an important mediatory role in spike initiation. Together, these results provide a better understanding of flowering mechanisms in Phalaenopsis, with important implications for orchid maturation and flowering management, and offer new insights into the regulation of first-time and seasonal spike initiation in perennial plants. Understanding of orchid flowering mechanisms is valuable in order to meet the constant demand worldwide for their exotic flowers. Therefore, this research may have commercial value as well as usefulness for basic research on flowering mechanisms.
Results
PaFTL expression patterns are correlated with spike initiation
To identify the genes involved in P. aphrodite spike initiation, we exposed wild-type (WT) plants to low ambient temperatures and analyzed the expression patterns of various flowering-related genes of leaves and axillary buds after a 3-day interval, based on genome annotation (Supplementary Fig. 1). We observed that at relatively high ambient temperatures, PaFTL transcript levels were most abundant in axillary buds, but remained at background levels in leaves (Fig. 1A). Following low–ambient temperature treatment, PaFTL transcription levels declined significantly in axillary buds, but remained relatively stable in leaves (Fig. 1B). In situ hybridization analysis revealed that PaFTL is primarily expressed at the apex of axillary buds, but low–ambient temperature treatment reduces its expression to background levels (Fig. 1C). These findings suggest that PaFTL may act as a repressor of spike initiation, preventing premature spike initiation until the appropriate environmental conditions are met.
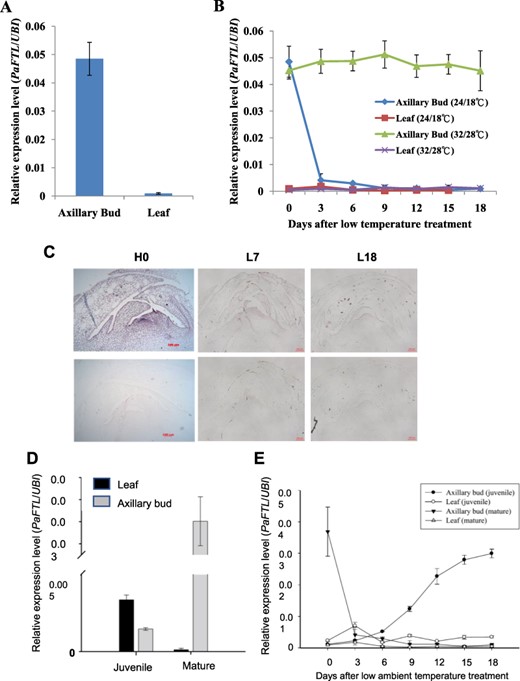
Expression patterns of PaFTL in P. aphrodite. (A) PaFTL expression levels in leaves and axillary buds. (B) PaFTL expression patterns following high– (10 h of light at 32°C/14 h of dark at 28°C) or low–ambient temperature (10 h of light at 24°C/14 h of dark at 18°C) treatment of leaves and axillary buds. Samples were harvested at 3-day intervals, and UBIQUITIN10 (UBI10) was used as an internal control. Error bars represent ±SD of three biological replicates. (C) In situ hybridization patterns of PaFTL in axillary buds subjected to high temperature (H0), 7 (L7) and 18 (L18) d of low-temperature treatment. Only FTL expression patterns appeared in H0, while background levels of PaFTL expression were detected in L7 and L18. (D) Relative expression levels of PaFTL in the leaves and axillary buds of juvenile and mature plants cultivated at high ambient temperatures (10 h of light at 32°C/14 h of dark at 28°C). The axillary buds of juvenile plants were harvested from 15 different plants and combined, and the error bars represent ±SD of three technical replicates normalized to UBI10 expression. Other samples were harvested from three different plants, and the error bars represent ±SD of three biological replicates normalized to UBI10 expression. (E) Expression patterns of PaFTL in response to low ambient temperatures (10 h of light at 24°C/14 h of dark at 18°C) in juvenile and mature plants. Plants were harvested at 3-day intervals, with samples collected at ZT8. Error bars represent ±SD of three biological replicates normalized to UBI10 expression. Standard errors were calculated from the means of three biological replicates.
Juvenile plants are unable to flower even under optimal environmental conditions, and the molecular mechanisms responsible for this phenomenon remain poorly understood in orchids. To understand if PaFTL can suppress spike initiation during the juvenile phase of P. aphrodite, we examined PaFTL expression levels in response to both high and low ambient temperatures in both juvenile and mature plants. In a high-temperature environment, PaFTL is expressed in the axillary buds and leaves of juvenile plants, while mature plants exhibited high levels of PaFTL expression only in the axillary buds (Fig. 1D). However, following 0–18 d of low-temperature incubation, PaFTL expression levels in the axillary buds of mature plants decreased dramatically, whereas leaf expression levels remained consistently low (Fig. 1E). Interestingly, PaFTL expression levels in the axillary buds of juvenile plants significantly increased after low-temperature treatment, while leaf expression levels remained unchanged (Fig. 1E). The upregulation of PaFTL in the axillary buds of juvenile plants was found to be correlated with the suppression of spiking. These observations indicated the existence of two distinct and opposing PaFTL regulatory mechanisms at different stages of plant development, which enable the control of the timing of first-time spike initiation.
PaFTL belongs to the FT-like family
To assess the homology between PaFTL and FT or TFL1 in other species, a detailed phylogenetic analysis was conducted. The 5′ and 3′ mRNA regions of PaFTL were first obtained via 5′ and 3′ RACE, and the full-length cDNA was then derived through RT-PCR. Phylogenetic analysis of the full-length coding region of PaFTL and other PEBP family genes from various plants revealed that PaFTL belongs to the FT-like clade (Fig. 2A). However, the presence of PaFTL in clades with other orchid FT-like genes, such as PaFT or OnFT, was not supported. Interestingly, the PaFTL gene contains a longer intron that resembles FT-like but not TFL1-like genes (Fig. 2B). Two domains in the FT protein, Y85 and segment B, are known to be critical for the promotion of flowering, but protein sequence alignments revealed that the PaFTL segment B domain is not particularly conserved (Fig. 2C). The FT homolog in maize, ZCN8, also has an unconserved segment B that differs from other FT homologs, but it nonetheless acts as a flowering activator. It is impossible to accurately predict the biological function of FT-like proteins based solely on the segment B domain sequence.
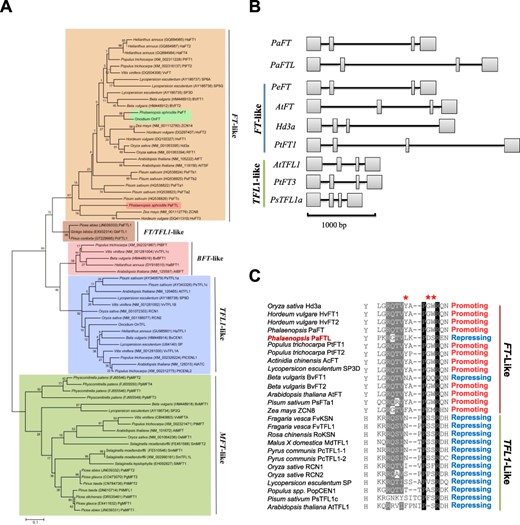
PaFTL belongs to the FT-like family. (A) Phylogenetic tree comprising PaFTL and PEBP family genes from other plant species was constructed using the ML method. An ML tree with 1,000 bootstrap replicates is shown. (B) Gene structure of PaFTL in comparison with other PEBP genes. Rectangular boxes represent exons, and lines represent introns. (C) Alignment of residue 85 in exon 2 and segment B for PaFTL and other members of the PEBP protein family. Asterisks indicate the three most important residues in segment B.
PaFTL acts as a flowering repressor
To examine the potential role of PaFTL in regulating flowering time, we ectopically expressed PaFTL, driven by the cauliflower mosaic virus 35S promoter, in Arabidopsis. The 35S::PaFTL was introduced into the WT and early-flowering tfl1-1 mutant Arabidopsis in the Columbia (Col) background, and the phenotype for flowering was observed. As shown in Fig. 3, tfl1-1 mutants flowered earlier than WT and vector control (p1390), with fewer rosette leaves (6 ± 0.4 vs. 8.8 ± 0.7 and 8.7 ± 0.6). However, 35S::PaFTL overexpression in both WT and tfl1-1 mutants resulted in delayed flowering, with significantly more leaves (28.4 ± 2.5 vs. 27.6 ± 6.6) (Fig. 3A, B). These results indicated that PaFTL has a strong repressive effect on flowering. We further evaluated PaFTL function in P. aphrodite, using a Cymbidium mosaic virus (CymMV)–based gene silencing system to conduct loss-of-function analysis (Lu et al. 2007). Two different partial fragments of PaFTL, PaFTL 5′and PaFTL 3′, were, respectively, inserted into the CymMV-based vector. Both CymMV-PaFTL 5′ and CymMV-PaFTL 3′-infected plants showed earlier spiking than the control plants under 30/28°C treatment. Out of 30 CymMV-PaFTL 5'-infected plants, 11 showed earlier spiking, and similarly, nine out of 30 CymMV-PaFTL 3'-infected plants displayed earlier flowering. Zero out of 20 CymMV empty vector infected displayed earlier flowering. This suggested that PaFTL also functions as a negative regulator of flowering in P. aphrodite (Fig. 3C, D). In general, inducing spike initiation in Phalaenopsis orchids requires temperatures below 25°C. To substantiate that PaFTL indeed functions to suppress spike initiation in Phalaenopsis orchids, our initial experiments were conducted at a high-temperature regime (32/30°C) known to inhibit spike initiation. However, it is possible that the virus-induced gene silencing (VIGS) efficiency was compromised at this temperature, as elevated temperatures can also inhibit virus expression, leading to a lack of observable spike initiation. Therefore, we adjusted the temperature to a constant (30/28°C) for the experiments.
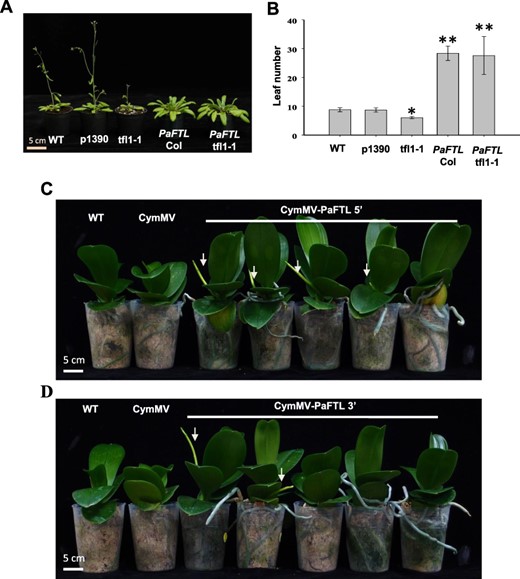
PaFTL is a flowering repressor. (A) Morphology and (B) flowering time of WT, p1390, tfl1-1, 35S::PaFTL in Col and 35S::PaFTL in tfl1-1 Arabidopsis plants. Plants were photographed 40 d after germination. More than 30 individual T3 plants for each construct were scored. Early spiking was observed in P. aphrodite plants infected with (C) CymMV-PaFTL 5′ or (D) CymMV-PaFTL 3′, both of which resulted in PaFTL gene silencing. These treated plants were cultured at 10 h of light at 30°C/14 h of dark at 28°C. WT and CymMV vector–inoculated plants were used as controls. Arrows indicate the flower spikes.
PaFTL interacts with PaFD
To identify the interaction between PaFTL and PaFD, we conducted bimolecular fluorescence complementation (BiFC) in Arabidopsis protoplast and yeast two-hybrid (Y2H) assays. The results obtained from the BiFC assay confirmed the interaction between PaFTL and PaFD, consistent with the interaction observed between PaFT and PaFD (Fig. 4A). The yeast containing both prey and bait vectors can grow on synthetically defined (SD) medium lacking Trp and Leu (SD-Trp-Leu) for the first screening (Fig. 4B, first row). The yeast strain is unable to synthesize His, and it is unable to grow on media that lack His. When bait and prey proteins interact, Gal4-responsive His3 expression allows the cell to biosynthesize the amino acids and grows on SD without His minimal medium. As shown in Fig. 4B, second row, the transformants expressing interacting pairs of pDEST32-PaFT (PaFT) or pDEST32-PaFTL and pEST22-PaFD (PaFD) in yeast cells could grow on synthetically defined (SD) medium lacking Trp, Leu and His (SD-Trp-Leu-His). Furthermore, as depicted in Fig. 4B, third row, yeast cells co-transformed with PaFTL and PaFD exhibited a blue color upon α-galactosidase activity, resembling the reaction observed with PaFT and PaFD. These results indicated that PaFTL could interact with PaFD as the interaction between PaFT and PaFD.
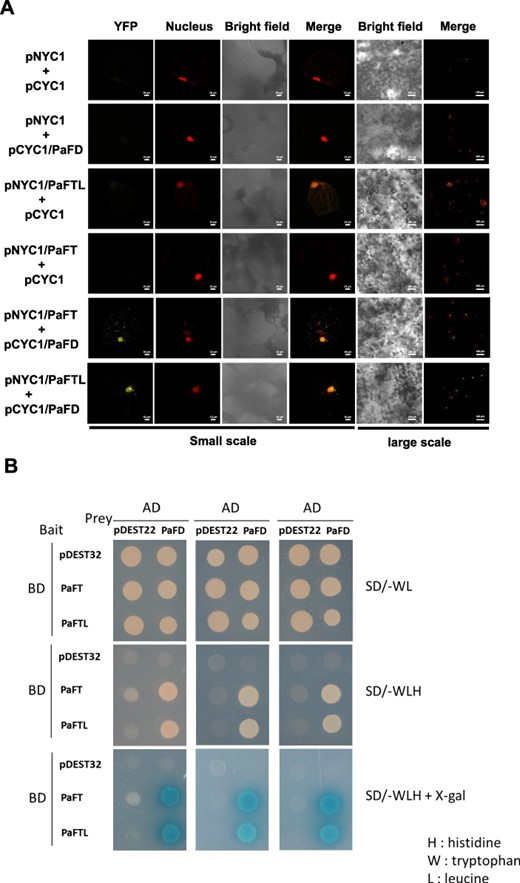
PaFTL physically interacts with PaFD. (A) PaFTL and PaFT interact with PaFD in BiFC assays. The bar is 10 μm in a small-scale field of view. The bar is 100 μm in a large-scale field view. (B) Yeast two-hybrid assay showing the interaction between PaFTL and PaFD. Yeast cells co-transformed with pDEST32 and pDEST22 (both were control), pDEST32 and pDEST22-PaFD (PaFD), pDEST32-PaFT (PaFT) and pDEST22, PaFTL and PaFD, pDEST32-PaFTL (PaFTL) and pEDST22, or PaFTL and PaFD, grown on SD/−WL, SD/−WLH or SD/−WLH + X-gal. pDEST22 is the prey empty vector, and pDEST32 is the bait empty vector.
PaFTL overexpression represses the flowering of P. aphrodite
Since PaFTL functions as a flowering repressor and its expression is upregulated in juvenile plants following low–ambient temperature treatment, we investigated whether sustained expression of PaFTL alone is sufficient to maintain the juvenile phase. We introduced the maize ubiqutin::PaFTL construct into P. aphrodite. In five out of 13 independent transgenic mature P. aphrodite plants, flowering was suppressed after low–ambient temperature treatment (Fig. 5A and Supplementary Fig. S2). We examined the expression levels of PaFTL in each transgenic plant, and the results showed that all flowering plants had low PaFTL expression, while the plants with suppressed flowering exhibited high levels of PaFTL expression (Fig. 5B, C). These results demonstrate that in Phalaenopsis plants that are exposed to low ambient temperatures during the juvenile phase, the significant induction of PaFTL expression is indeed sufficient to suppress flowering and maintain the plants in their juvenile phase. Furthermore, we further subjected the non-spiked transgenic plants, as shown in Fig. 5A, to high-temperature treatment for a month. Subsequently, we analyzed the expression patterns of PaFT, PaAP1 and PaFD in these overexpression plants using real-time RT-PCR in the axillary buds following low–ambient temperature treatment. In PaFTL transgenic plants, the expression of PaFTL remained high even after 18 d of low-temperature treatment (Fig. 6A). In WT plants, the expression level of PaFT increased with the duration of low-temperature treatment. However, in PaFTL transgenic plants, although the expression level of PaFT initially increased after 6 d of low-temperature treatment, it subsequently decreased after 18 d (Fig. 6B). The expression level of PaFD showed no significant changes (Fig. 6C). The expression pattern of PaAP1 resembled that of PaFT, and the transgenic plants showed lower expression of PaAP1 compared to WT (Fig. 6D).
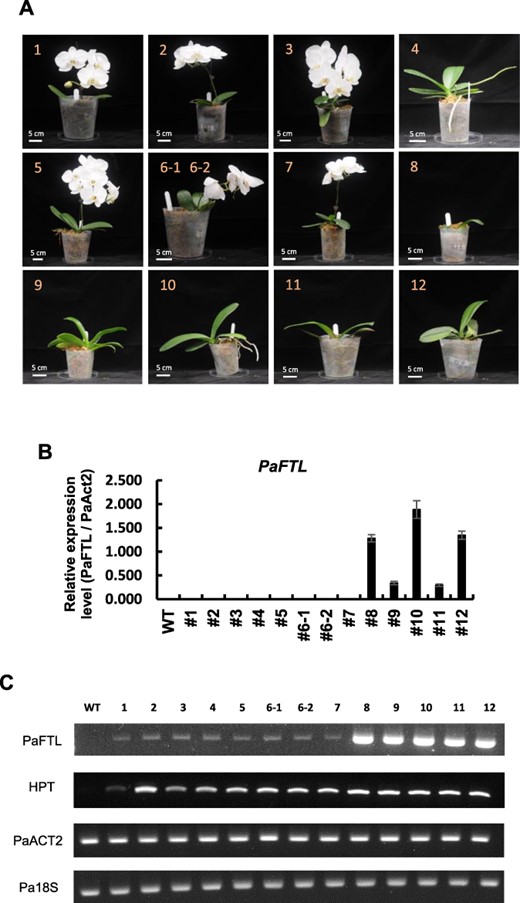
PaFTL overexpression represses flowering in P. aphrodite. (A) Representative phenotypes of transgenic P. aphrodite with maize ubiqutin:PaFTL construct. (B) Quantitative real-time PCR to detect PaFTL expression from leaves of WT or various PaFTL-OX lines. Error bars represent ±SD of three biological replicates, normalized to UBI10 expression. (C) Semi-quantitative RT-PCR to detect relative expression of PaFTL, hygromycin phosphotransferase II, act2 and 18S of WT and various transgenic plants by RT-PCR analysis.
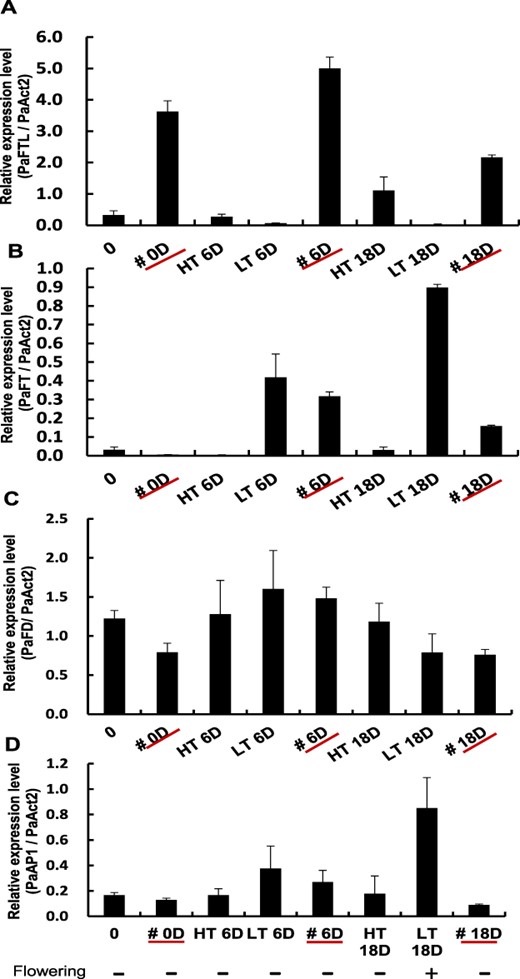
The relative expression levels of PaAP1 was reduced in transgenic PaFTL overexpression plant at low ambient temperatures. (A) Relative expression level of PaFTL in various plants. (B) Relative expression level of PaFT in various plants. (C) Relative expression level of PaFD in various plants. (D) Relative expression level of PaAP1 in various plants. # represents the PaFTL transgenic plant. HT: high ambient temperature. LT: low ambient temperature. 0: WT plants grown at 32°C/30°C. #0D: PaFTL transgenic plants grown at 32°C/30°C. HT6D: WT plants grown at 32°C/30°C for 6 d. LT6D: WT plants grown at 24°C/18°C for 6 d. HT18D: WT plants grown at 32°C/30°C for 18 d. LT18D: WT plants grown at 24°C/18°C for 18 d. #6D and #18D: PaFTL transgenic plants grown at low temperature for 6 and 18 d, respectively. Abbreviations: HT, high ambient temperature; LT, low ambient temperature.
Discussion
Spiking induction and dormancy
While low ambient temperature–induced spike initiation has been successfully applied in the orchid industry for many years, the underlying factors and mechanisms involved have not been extensively studied. In this study, we show that PaFTL plays a significant role in spiking repression in P. aphrodite. However, it can be challenging to differentiate between spiking repression and dormancy maintenance in the axillary buds. In 2011, Kalgren et al. proposed that plant PEBP gene function is associated with growth arrest and/or dormancy. Hence, we speculated that PaFTL may repress spiking by preserving the dormancy of axillary buds. It should be noted that a PEBP gene, Pa (Picea abies) FTL2, also controls growth cessation in the Norway spruce (P. abies) (Karlgren et al. 2013), suggesting that the mechanisms underlying seasonal dormancy in woody plants may share some similarities with apical dominance in P. aphrodite.
PaFTL acts as a floral repressor
The genes FT and TFL1 in Arabidopsis encode a pair of flowering regulators downstream of the flowering pathways. Their proteins are homologs of the PEBPs. While they have amino acid sequence similarity up to ∼60%, they play opposite roles (Hanzawa et al. 2005, Imamura et al. 2011). Furthermore, exchanging a single amino acid is enough to switch the functions of FT and TFL1 proteins (Hanzawa et al. 2005). In P. aphrodite, a FT homolog (PaFT1) promotes flowering, and its ectopic expression recovers late flowering in Arabidopsis ft mutant (Jang et al. 2015). Moreover, the TFL1 suppression spike initiation phenomenon has already been observed in other species such as Oncidium Golden Ramsey (Orchidaceae) (Hou and Yang 2009) and Gentiana (Imamura et al. 2011). The conservation of key amino acid residues, such as histidine in TFL1 and tyrosine in FT, suggests that the FT/TFL1 gene family evolution has been a major target in nature (Wickland and Hanzawa 2015). In this study, we showed that PaFTL functions as a floral repressor, a function similar to TFL1, as evidenced by its high expression levels in juvenile plants under conditions that initiate spiking. The evidence presented in this study supports this prediction, as shown by the segment B domain in PaFTL, which is not particularly conserved as compared to other FT.
Functional structure of repressor proteins
Inducer FTs and repressor FTs have been previously reported from several species such as Arabidopsis (Kardailsky et al. 1999), Norway-spruce (Karlgren et al. 2011), onion (Lee et al. 2013), sugar beet (Pin et al. 2010), soybean (Nan et al. 2014, Zhai et al. 2014), tobacco (Harig et al. 2012) and sugarcane (Coelho et al. 2014). Amino acid alignment on FT and FTL genes from these species by Wickland and Hanzawa (2015) revealed that all inducer FTs have tyrosine at position 134, except GmFT5a from Glycine max, while repressor FTs have non-tyrosine amino acids, except FT-/TFL1-like such as P. abies PaFTL1 and PaFTL2 (Wickland and Hanzawa 2015). Also, all inducer FTs have tryptophan at position 138, while repressor FTs, except DlFT2 and GmFT4, contain non-tryptophan amino acids. Our results showed that three amino acid residues in the B segment of PaFT and PaFTL (Fig. 2C) may be the key determinants of their opposite function in P. aphrodite. Ho and Weigel (2014) reported the effects of mutations of FT in Arabidopsis to explore the important residues all over the FT protein (Ho and Weigel 2014). They found that FT in Arabidopsis was robust to a wide range of mutations, even in highly conserved residues. However, when specific mutations occurred in at least four different residues, they were adequate to convert inducer FT into a complete repressor TFL1. However, Wang et al. (2017) discovered that substitutions of key amino acid residues, specifically Tyr-85 and Gln-140, which are essential for FT function, in TFL1 homologs from Rosaceae species did not promote flowering, suggesting that homologs of FT/TFL1 with high sequence similarity and crystal structures may have diverse functions (Wang et al. 2017).
FT family and plant architecture
Moraes et al. (2019) aimed to interpret the role of two homolog proteins, FT and TFL1, in regulating plant architecture and growth rhythm. They described plant architecture as being determined by the balance between FT and TFL1, which in turn modulate the formation of vegetative and reproductive structures in the apical and axillary meristems. In monopodial orchids such as Phalaenopsis, the apical meristem remains in a vegetative state to support the growth of new leaves. Reproductive structures, such as the spike, are differentiated from the meristem of the axillary bud. Therefore, the axillary bud or meristem is an important element in completing plant architecture and ensuring reproductive success (Wang and Jiao 2018). The FT/TFL1 balance also influences the flexibility of the axillary meristem activity (McGarry and Ayre 2012). A decrease in the FT/TFL1 ratio results in an increase in vegetative growth and overall vegetativeness, while an increase in FT expression and a low FT/TFL1 ratio promote generative growth and early flower initiation, which results in the conversion of the apical meristem into a terminal flower (Moraes et al. 2019).
This prediction could be further explored in P. aphrodite, which contains PaFT1 as a key flowering regulator and for which upregulation has been observed during flowering inductive cooling treatment (Jang et al. 2015). Investigating the PaFT1 and PaFTL ratio under inductive (low ambient temperature) and non-inductive (high–ambient temperature) flowering conditions in P. aphrodite could be a topic worthy of further research.
FTL and juvenile plants
In our previous experiments, we conducted VIGS experiments during the juvenile stage, but the results were not satisfactory, so we chose not to include them in the article. The reason for not observing early flowering during the juvenile stage may be that solely reducing PaFTL is insufficient to promote flowering at this stage. The ineffectiveness of VIGS during the juvenile stage might be attributed to the inability to decrease PaFTL to a threshold that induces flowering. Goretti et al. (2020) analyzed the functions of flowering repressor TFL1 in Arabidopsis by using a constitutively expressed Glucocorticoid Receptor (GR) fusion protein (TFL1-GR). The results showed that TFL1-GR interacted with other genes, including those related to cellular and metabolic processes, as well as stimulant responses. Moreover, genes that are promoters of circadian clock regulators, flowering time and flower development were found enriched in TFL1. Furthermore, TFL-GR was also observed to significantly downregulate several floral identity genes, including LFY, CAULIFLOWER and FRUITFULL, which suggests that TFL1 functions as a co-transcription factor that regulates the expression of hundreds of genes in Arabidopsis. We have demonstrated that PaFTL plays a crucial role in regulating the timing of first spike initiation in mature P. aphrodite. This is supported by the significant upregulation of PaFTL expression in the axillary buds of juvenile plants under flowering induction conditions, which involve low ambient temperature. Furthermore, it would be worthwhile exploring the potential role of PaFTL as a co-transcription factor, similar to TFL1 in Arabidopsis, in regulating other flowering genes in P. aphrodite in future research.
The juvenile phase is the early stage of vegetative development in plants. During the juvenile phase, spike initiation does not occur even under favorable environmental conditions as flowering competency has not been achieved. This feature is related to the age-dependent response to environmental signals, although the details remain poorly understood. In woody plants such as Populus deltoides, FT2 acts as a flowering activator and its mRNA levels increase three-fold during the mature phase compared to the juvenile phase (Hsu et al. 2006). In A. alpina, AaTFL1 functions as a flowering repressor that appears to block the flowering of juvenile plants (Wang et al. 2011). During the vernalization of mature plants, AaTFL1 expression gradually declines, while the expression of AaLFY increases to promote flowering. In contrast, the vernalization of the juvenile plant does not alter AaTFL1expression levels, and AaLFY was not expressed (Wang et al. 2011). Here, we show similar age-dependent responsiveness to low ambient temperatures in P. aphrodite. Following exposure to low ambient temperatures, PaFTL was highly expressed in the axillary buds of juvenile plants (Fig. 7A), preventing premature flowering. On the other hand, PaFTL expression levels dramatically declined in the axillary buds of mature plants exposed to low ambient temperatures (Fig. 7B). These opposing mechanisms allow PaFTL to regulate spike initiation, which may have critical implications for orchid maturation and flowering management. These results also provide new insights into the flowering regulatory mechanisms of perennial plants. Juvenile plants exposed to environmental conditions that stimulate flowering (such as a cold treatment or long days) do not flower as a well-known phenomenon. This phenomenon suggests that plants exhibit different gene expressions in response to the same environmental factors at different stages of growth; however, the regulatory mechanisms behind this remain a mystery. Our data showed that low temperature has opposing regulatory effects on PaFTL during the juvenile and mature stages of plant development. One of the possibilities for this lies in the differential regulation of upstream genes of PaFTL induced by low temperature during different developmental stages. Further research is needed to confirm the key genes that control this phenomenon.
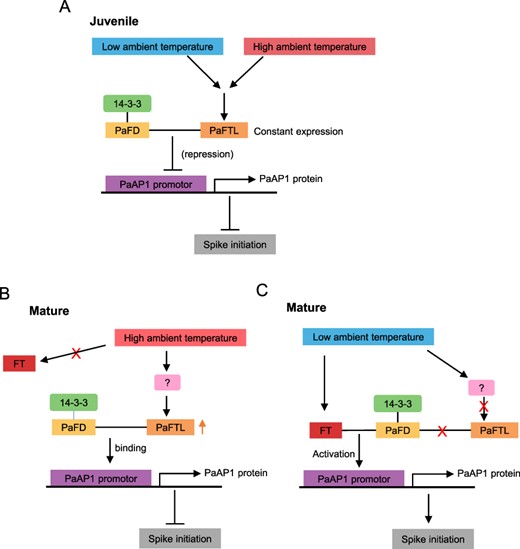
Models illustrating the proposed role of PaFTL and temperature in flowering regulation in juvenile and mature plants. (A) In the juvenile phase, the abundance of the flowering repressor PaFTL increases in response to low ambient temperature and flowering is consequently suppressed. (B) In the mature phase, the abundance of the flowering repressor PaFTL decreases in response to low ambient temperature, and flowering is consequently accelerated. (C) In the mature phase, the abundance of the flowering repressor PaFTL increases in response to high ambient temperature and flowering is consequently suppressed. The positive and negative controls are represented by arrows and perpendicular lines, respectively.
At high temperatures, the expression of PaFTL was higher in the axillary buds of mature plants than that of juvenile plants, as shown in Figs. 1 and 7C. Conversely, at a lower temperature, the expression of PaFTL in the axillary buds of mature plants decreased 3 d after treatment with low ambient temperature, while it constantly increased in juvenile plants. This pattern revealed that, under inductive flowering conditions, juvenile P. aphrodite suppresses spike initiation through the high expression of PaFTL in the axillary buds.
Materials and Methods
Plant materials and growth conditions
Phalaenopsis aphrodite subsp. formosana (M1904) seedlings were purchased from Chain Port Orchid Co., Ltd. (Ping Tung, Taiwan) and were grown in 1.5-inch (juvenile stage) and 3-inch (mature stage) diameter plastic pots. All plants were grown at a high ambient temperature of 32°C/30°C, with a 10-h photoperiod, to maintain vegetative growth. To induce spiking, plants were shifted to the low ambient temperature of 24°C/18°C with a 10-h photoperiod.
Arabidopsis thaliana ecotype Columbia-0 was used in all experiments. The Arabidopsis plants were grown in environment-controlled growth chambers at 24°C, 70% relative humidity, with a 16-h photoperiod (approximately 120 μmol m–2 s–1) on 0.5 × Murashige–Skoog agar media, with or without hygromycin (20 mg l−1). Young seedlings were later transplanted to soil and kept under the same environmental conditions for continuous growth. The flowering time was defined as the number of rosette leaves formed on the main shoot when the main stem had bolted 1 cm.
RNA extraction and reverse transcription reaction
Samples of P. aphrodite were collected and ground in liquid nitrogen, and RNA was extracted using TEN buffer (7.8 mM Tris, 15.6 mM Na2EDTA, 156 mM NaCl, 4% SDS and 16 mM DTT, pH 9.0) following phenol–chloroform extraction. The RNA was precipitated using a final concentration of 2 MLiCl after overnight incubation at 4°C. For A. thaliana, total RNA was extracted from leaf samples using Trizol reagent (Invitrogen, Carlsbad, CA, USA) according to the manufacturer’s instructions. To synthesize cDNA, 1 μg of RNA was reverse transcribed in 30 μl of reverse transcription reaction using a First-strand cDNA Synthesis kit (Promega, Madison, WI, USA) and oligo-dT primer. Then, 70 μl of ddH2O was added to the synthesized cDNAs.
Real-time quantitative RT-PCR and RACE-PCR
Real-time RT-PCR was carried out using SYBR Green Master Mix (Applied Biosystems, Foster City, CA, USA) and monitored using an ABI 7500HT sequence detection system (Applied Biosystems) or CFX96 Real-Time PCR detection system (Bio-Rad, Hercules, California, USA). The quantification analysis was carried out using the ABI SDS 1.4 software (Applied Biosystems) or CFX Manager Software (Bio-Rad) according to the manufacturer’s instructions. The relative transcript levels were normalized to the expression of both endogenous control genes, Ubi10 and 18S rRNA, using the comparative cycle threshold (Ct) method. Twenty microliters of qPCR reaction contained 3 μl of cDNA, 0.25 mM of primers and 10 μl of KAPA SYBR FAST master mix (KAPA Biosystems, Wilmington, Massachusetts, USA). qPCR was performed in triplicate technical repeats, and the experiments were repeated at least twice with RNA isolated from independent samples. The primers used for qPCR and RACE-PCR are listed in Supplementary Table S1. The 5ʹ and 3ʹ RACE-PCR was carried out using the SMARTer RACE cDNA amplification kit according to the manufacturer’s instructions (Clontech, Palo Alto, California, USA).
Phylogenetic tree construction
The protein sequences were aligned by ClustalW, and the resulting alignments were used to construct phylogenetic trees in Mega 5.05 (Tamura et al. 2011). The maximum-likelihood (ML) method was employed to generate phylogenetic trees, and bootstrapping was performed with 1,000 replicates. Bootstrap values of 50% or higher are shown for each clade. The gene identification accession numbers used to generate the phylogenetic trees are shown in Fig. 2.
In situ hybridization
Axillary buds of Phalaenopsis orchids were collected and immediately fixed in a fixation solution (containing 4% paraformaldehyde, 0.25% glutaraldehyde, 0.1% Tween 20 and 0.1% Triton X-100 in diethyl pyrocarbonate-treated H2O) at 4°C overnight. The tissues were dehydrated, infiltrated with Paraplast (Leica, Teaneck, NJ, USA) and sectioned vertically into 10-µm thickness using a microtome (Leica). The sections were mounted onto a poly-l-lysine-coated slide (Matsunami, Osaka, Japan), deparaffinized with xylene, rehydrated in decreasing concentrations of ethanol and then treated with 2 mg ml−1 proteinase K at 37°C for 40 min. To prepare the digoxigenin-labeled RNA probes, the gene-specific region of PaFTL was amplified using the primers listed in Supplemental Table S1. Sense and antisense probes were synthesized using a SP6/T7 digoxigenin RNA labeling kit according to the manufacturer’s instructions (Roche, Indianapolis, IN, USA). In situ hybridization was performed as previously described (Lin et al. 2013) with some modifications. Briefly, hybridization was carried out at 53°C for PaFTL in the presence of 40 ng of digoxigenin–anti-digoxigenin DIG-labeled RNA probes. Hybridization signals were detected using the NBT/BCIP detection kit (Roche). Tissue sections were photographed on a Zeiss Axio Scope A1 microscope equipped with an Axio-Cam HRc camera (Zeiss, Oberkochen, Germany) after in situ hybridization.
Construction of pCambia-CymMV-PaFTL plasmids and transformation for the VIGS study
To identify PaFTL function by a VIGS system, the DNA fragment flanking the 5′ and 3′ region was PCR amplified using the primers listed in Supplemental Table S1. The resulting PCR product was cloned into the pCambia-CymMV gateway vector by in vitro recombination with attB × attP BP clonase II (Invitrogen) to generate pCambia-CymMV-PaFTL 5ʹ and pCambia-CymMV-PaFTL 3' vectors. These plasmids were sequenced and subsequently transformed into Agrobacterium tumefaciens strain LBA4404 by electroporation. A. tumefaciens harboring pCambia-CymMV-PaFTL 5', pCambia-CymMV-PaFTL 3ʹ or pCymMV-pro60 empty vector, as a control, were grown and prepared as previously described (Lu et al. 2012) and infiltrated into the leaves of P. aphrodite using a 1-ml syringe with a needle. Agrobacterium-infiltrated plants were cultured at 30°C/28°C with a 10-h photoperiod. Since PaFTL is primarily expressed in the axillary buds, with inherently low expression levels in the leaves, if we removed the axillary buds from the Agrobacterium-infiltrated plants to assess a potential decrease in PaFTL expression, subsequent experiments related to spiking in the same plant would be rendered unfeasible. Therefore, we could not provide the evidence that FTL expression was downregulated in FTL-VIGS experiments. We could only compare with the control group, vector only, to observe if there are any changes in the developmental time of the flower spike.
Construction of PaFTL overexpression plasmids and performance of plant transformation
To observe the phenotype resulting from overexpression of PaFTL in orchid or Arabidopsis, the full-length cDNA was PCR amplified using the primers listed in Supplemental Table S1. The resulting PCR product was cloned into pDONR221 (Invitrogen) via the BP reaction by using Gateway BP Clonase II enzyme mix (Invitrogen), resulting in pDONR221-PaFTL. The full-length PaFTL cDNA was then subcloned into the binary vector pCambia-Maize Ubiquitin promoter gateway or pH7GW2 (adrgene) vector via the attL X attR (LR) reaction by using Gateway LR Clonase II enzyme mix (Invitrogen) according to the manufacturer’s instructions to generate the pCambia-Ubi-PaFTL (for orchid transformation) or pH7-PaFTL (for Arabidopsis transformation) vectors. These plasmids were sequenced and subsequently transformed into A. tumefaciens strain EHA105 (for orchid transformation) or GV3101 (for Arabidopsis transformation) by electroporation. A. tumefaciens harboring the pCambia-Ubi-FTL vector were grown and used to infect P. aphrodite protocorm-like bodies as described (Liao et al. 2004). A. tumefaciens harboring pH7-PaFTL vector were grown and used for floral dip transformation in Arabidopsis as described (Zhang et al. 2006).
Confirmation of protein–protein interaction by BiFC and yeast two hybridization
To demonstrate that FTL can interact with FD in a manner similar to FT interaction with FD, we cloned the full-length coding regions of FTL, FD and FT into BiFC vectors (pNYC1 or pCYC1) (Walter et al. 2004). Arabidopsis protoplasts were isolated using the tape–A. thaliana sandwich method and co-transformed with a plasmid-expressing nuclear-localizing marker (bZIP63–cyan fluorescent protein) (Walter et al. 2004) or BiFC plasmids using the polyethylene glycol method (Wu et al. 2009). After incubation at room temperature for 16 h in light, the protoplasts were observed with a Zeiss LSM510 META laser scanning confocal microscope (Carl Zeiss, Jena, Germany).
The ProQuest two-hybrid system (Invitrogen) was employed to perform a yeast two-hybrid assay. A protein of interest is fused to the DNA-binding domain (BD), and this acts as the bait construct. Then, the candidate interactor is fused to the activation domain (AD) to act as prey constructs. The pDEST22 is the prey empty vector, and the pDEST32 is the bait empty vector. FD was cloned into pDEST22 as the prey, and FT and FTL were cloned into pDEST32 as the bait. The construct pairs were co-transformed into yeast strain MaV203 according to the manufacturer’s instructions (Invitrogen). Yeast cells co-transformed with pDEST32 and pDEST22 (both were control), pDEST32 and pDEST22-PaFD (PaFD), pDEST32-PaFT (PaFT) and pDEST22, PaFTL and PaFD, pDEST32-PaFTL (PaFTL) and pEDST22, or PaFTL and PaFD, grown on SD medium lacking, were used to test essential amino acids. Positive yeast transformants were selected on SD medium without leucine, tryptophan (SD/−WL), SD medium without leucine, tryptophan and histidine (SD/−WLH) and SD/−WLH with 5-bromo-4-chloro-3-indolyl-beta-d-galacto-pyranoside (SD/−WLH + X-gal). Appropriate controls were included by co-transforming pEXP32/Krev1 with pEXP22/RalGD-WT (the WT with strong interaction), pEXP22/RalGD-m1 (a mutant with weak interaction) and pEXP22/RalGD-m2 (a mutant with no interaction).
Supplementary Data
Supplementary Data are available at PCP online.
Data Availability
All data supporting the findings of this study are available within the paper and within its Supplementary Material published online. Sequence data from this article can be found in the Orchidstra database, at https://orchidstra2.abrc.sinica.edu.tw/orchidstra2/index.php. The following are the accession numbers: PaFT (PAXXG186610), PaFTL (PAXXG182430), PaELF3 (PAXXG106330), PaFCA (PAXXG024500), PaPHY (PAXXG067160), PaGI (PAXXG103510), PaFY (PAXXG338510), PaFPA (PAXXG346200), PaACT2 (PAXXG077410), PaAP1 (PAXXG045840), PaSOC1 (PAXXG065170), PaFD (PAXXG289490), PaSVP1 (PAXXG194760) and PaLFY (PAXXG345890).
Funding
Academia Sinica and AS-KPQ-108-ITAR-03.
Acknowledgments
We would like to thank the ABRC Bio 101 core facility, advanced optical microscope core facility of academia Sinica, in situ hybridization core of the Academia Sinica Biotechnology Center in Southern Taiwan (AS-BCST) and orchid transformation core Facility of AS-BCST and the greenhouse core facilities of Academia Sinica for their supporting services and Ms Miranda Loney for suggestions, comments and English editing.
Author Contributions
M.-T.C. planned the experiments and conception; H.-C.L., C.-W.H. and D.S. conducted the experiments and did the required measurements; H.-C.L. was responsible for preparing the first draft of the manuscript. H.-C.L., C.W.H., T.M. and D.S. helped to collect and analyze the data. T.M. and M.-T.C. revised the manuscript. M.-T.C. obtained the funding and were responsible for this manuscript. All authors contributed to the article and approved the submitted version.
Disclosures
The authors have no conflicts of interest to declare.
References
Author notes
contributed equally.