-
PDF
- Split View
-
Views
-
Cite
Cite
Kunpeng Liu, Chunmei Yin, Wenjing Ye, Min Ma, Yuanda Wang, Pan Wang, Yuda Fang, Histone Variant H3.3 Controls Arabidopsis Fertility by Regulating Male Gamete Development, Plant and Cell Physiology, Volume 65, Issue 1, January 2024, Pages 68–78, https://doi.org/10.1093/pcp/pcad119
- Share Icon Share
Abstract
Reprograming of chromatin structures and changes in gene expression are critical for plant male gamete development, and epigenetic marks play an important role in these processes. Histone variant H3.3 is abundant in euchromatin and is largely associated with transcriptional activation. The precise function of H3.3 in gamete development remains unclear in plants. Here, we report that H3.3 is abundantly expressed in Arabidopsis anthers and its knockout mutant h3.3–1 is sterile due to male sterility. Transcriptome analysis of young inflorescence has identified 2348 genes downregulated in h3.3–1 mutant, among which 1087 target genes are directly bound by H3.3, especially at their 3ʹ ends. As a group, this set of H3.3 targets is enriched in the reproduction-associated processes including male gamete generation, pollen sperm cell differentiation and pollen tube growth. The function of H3.3 in male gamete development is dependent on the Anti-Silencing Factor 1A/1B (ASF1A/1B)-Histone regulator A (HIRA)-mediated pathway. Our results suggest that ASF1A/1B-HIRA-mediated H3.3 deposition at its direct targets for transcription activation forms the regulatory networks responsible for male gamete development.
Introduction
Nucleosomes are the basic building blocks of chromatin, the genetic material of eukaryotes. Nucleosome core particles are composed of about 146 bp (base pairs) of DNA entwined with histone octamers. The histone octamer consists of two copies of each core histone H3, H4, H2A and H2B organized into an (H3–H4)2 tetramer flanked by two (H2A–H2B) dimers (Luger et al. 1997). The assembly of nucleosome arrays into chromatin facilitates the compression of the genome and influences all the DNA-based processes, including replication, transcription, recombination and repair. Therefore, a proper control of the dynamics of chromatin organization ensures accurate genome function. In addition to specific post-translational modifications on N-terminal extremities of histones, the histone variants offer another way to regulate the structure and function of chromatin (Hake and Allis 2006).
The histone H3 family in Arabidopsis contains four major histone variants: H3.1, H3.3, AtMGH3/H3.10 and CenH3/HTR12 (Okada et al. 2005). CenH3 differs from other H3 histones significantly in sequence and is specifically enriched in the kinetochore region of chromosomes, which is essential for the assembly of mitoses and the correct segregation of chromosomes during cytokinesis (Talbert et al. 2002). AtMGH3 is a pollen-specific variant, yet its complete deletion almost does not affect Arabidopsis development and fertility (Okada et al. 2005, Borg et al. 2020, Zhu et al. 2023). Arabidopsis histones H3.1 and H3.3 differ in sequence by only four amino acid residues, but their distribution and function are significantly different (Shi et al. 2011, Stroud et al. 2012). H3.1 is enriched in heterochromatin regions, and its loading into chromatin is DNA replication–dependent (Stroud et al. 2012, Jiang and Berger 2017). In contrast, H3.3 is mainly enriched at the transcribed genes and positively correlated with mRNA content (Stroud et al. 2012, Wollmann et al. 2012). Arabidopsis contains three genes encoding H3.3 (HTR4, HTR5 and HTR8) (Okada et al. 2005), and H3.3 mutants exhibit abnormal leaf development, fertility defects, early flowering and abnormal post-embryonic development (Wollmann et al. 2017, Zhao et al. 2021, 2022). Xenopus H3.3 is essential for gastrulation development (Szenker et al. 2012, Sitbon et al. 2020). Drosophila H3.3 knockout mutants had reduced viability and were sterile in both males and females (Hodl and Basler 2009, Sakai et al. 2009). Complete deletion of the two H3.3-coding genes in mouse results in early embryonic lethality, while knockout one of them causes reduced viability and fertility (Jang et al. 2015).
The few amino acids different between H3.1 and H3.3 are distinguished by specific molecular chaperones to load the corresponding histone into nucleosomes. In human cells, the Chromatin Assembly Factor-1 (CAF-1) complex loads histone H3.1 in a DNA synthesis–coupled manner, while the deposition of H3.3 is mediated by the Histone regulator A (HIRA) complex or Death domain-associated protein (Daxx)/Alpha Thalassemiamental Retardation X-linked (ATRX) heterodimer (Tagami et al. 2004, Lewis et al. 2010). Another histone chaperone ASF1 cooperates with CAF-1 and HIRA complexes to load H3.1 and H3.3, respectively (Tagami et al. 2004). Daxx has not been identified in plants, and it was known that Arabidopsis genome encodes the HIRA complex, ATRX and ASF1. The HIRA complex consists of three subunits, HIRA, Ubinuclein 1/Ubinuclein 2 (UBN1/UBN2) and Calcineurin Binding protein 1 (CABIN1), which regulate the root length, cotyledon morphology and reproductive development in Arabidopsis (Nie et al. 2014, Duc et al. 2015). ATRX acts as a complementary pathway to the HIRA complex and is involved in the deposition of histone H3.3 (Duc et al. 2017, Wang et al. 2018). Unlike ASF1 in human cells, ASF1 in Arabidopsis prefers to interact with the HIRA complex to participate in H3.3 loading (Zhong et al. 2022).
Male germline development in flowering plants involves two distinct phases, microsporogenesis and microgametogenesis, which include one meiosis followed by two rounds of mitosis in anthers (Huang et al. 2021). The entire process of anther development starts from the emergence of the stamen primordium to pollen release and has been artificially divided into 14 periods (Sanders et al. 1999). In short, microspore mother cells undergo meiosis between stages 5 and 7 within each of the four locules and generate tetrads of haploid microspores. Then, microspores are released from the tetrads at stage 8 and differentiate into three-celled pollen grains between stage 9 and stage 12 (Sanders et al. 1999). Numerous factors that involved anther development have been identified (Ge et al. 2010, Zhu et al. 2011a, Huang et al. 2021). For example, AtMGH3 regulates the establishment of H3K27me3 on some genes in pollen (Borg et al. 2020, Zhu et al. 2023). AGL66 regulates pollen maturation and pollen tube growth through interacting with AGL104 (Adamczyk and Fernandez 2009). The MYB transcription factor DUO1 plays an important role in the formation of spermatocytes (Rotman et al. 2005) and promotes the transcription of MAPKKK20 and OPT8 (Borg et al. 2011).
H3.3-coding genes were known to be expressed in Arabidopsis pollen (Okada et al. 2005, Ingouff et al. 2010, Borg et al. 2020). However, as the H3.3 deletion mutant was speculated to be embryo lethal due to abnormal germination (Wollmann et al. 2017, Zhao et al. 2022), little is known about the specific function of H3.3 in plant reproductive development. Here, we identified a new H3.3 knockout mutant h3.3–1 and found that h3.3–1 mutant plants are viable but sterile through male sterility. H3.3 is abundantly expressed in anthers and required for the expression of a set of genes in inflorescence, which are H3.3 targets enriched in reproductive processes including male gamete generation, pollen sperm cell differentiation and pollen tube growth. In addition, the function of H3.3 on male gamete development is dependent on its deposition through the ASF1A/1B-HIRA pathway. Our data uncovered a regulatory pathway for the male gamete–specific development related to the transcription and deposition of the histone variant H3.3.
Results
Loss-of-function mutant of H3.3 displays pleiotropic phenotypes
In Arabidopsis, H3.3 is encoded by HTR4, HTR5 and HTR8 three genes (Fig. 1A), where HTR4 and HTR5 are arranged in tandem on chromosome 4 (Supplementary Fig. S1A). To gain more insight into the role of H3.3 in plant development, we identified two T-DNA inserted mutants, SALK_042781 and SALK_087850 (Supplementary Fig. S1A). The T-DNA insertion of SALK_042781 is located in the first exon of HTR5 gene, interrupting both HTR5 and the downstream gene HTR4 (Supplementary Fig. S1A, B). The T-DNA insertion of SALK_087850 is located in the first exon of HTR8 (Supplementary Fig. S1A). To determine the effect of the T-DNA insertion in HTR5 on HTR4, we designed primer RB1.1 located near the RB end of the T-DNA (pBIN-pROK2) and the paired primers HTR4-F, F1, F2, F3 and F4 (Supplementary Fig. S1A). The PCR products were amplified when RB1.1 was paired with F3 or F4 using htr5-4 genomic DNA as the template (Supplementary Fig. S1C). The PCR products of RB1.1 and F3 were cloned in the pMD-19 vector and sequenced. The sequencing results indicate that the insertion of T-DNA in HTR5 leads to a replacement of the HTR4 region (from the RB of the inserted T-DNA to site A) by another inverted T-DNA sequence (Supplementary Fig. S1A). Thus, the sequence of HTR4 could not be detected in the htr5-4-associated mutants (Supplementary Fig. S1B). Subsequently, we named SALK_042781 and SALK_087850 as htr4htr5-1 and htr8-3, respectively. Gene transcripts in these mutants were examined by RT-PCRs. As expected, both the full-length transcripts of HTR4, HTR5 and HTR8 were undetectable in the corresponding mutants, suggesting that the mutants htr4htr5-1 and htr8-3 are loss-of-function mutants (Supplementary Fig. S1D). None of these mutants showed any obvious phenotype under our growth conditions (Fig. 1, B-D). We then crossed htr4htr5-1 with htr8-3 and obtained the triple mutant htr4htr5 htr8 (hereinafter short-named h3.3–1) (Supplementary Fig. S1C). RT-PCR analysis confirmed that all the HTR4, HTR5 and HTR8 full-length transcripts were absent in the h3.3–1 mutant (Supplementary Fig. S1D). The h3.3–1 showed delayed germination (Supplementary Fig. S2A, B) (Zhao et al. 2022), smaller in size (Fig. 1B, C), reduced rosette leaves at bolting (Supplementary Fig. S2C, D) and sterility (Fig. 1D).
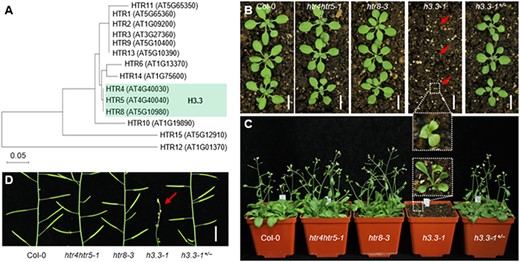
The phenotypes of H3.3 homozygous mutant plants. (A) Phylogenetic tree of Arabidopsis histone H3. The protein sequences of 14 histone H3 family proteins were analyzed by Molecular Evolutionary Genetics Analysis-X (MEGA-X). (B) Morphology of representative 17-day-old plants grown under LD conditions at 22°C. Scale bar, 5 cm. (C) Morphology of 28-day-old plants grown under LD conditions at 22°C. (D) Morphology of plant inflorescence stems grown under LD at 22°C. Scale bar, 1 cm.
Impaired function of the male gametophyte controls sterility in the h3.3-1 mutant
The most remarkable phenotype of the h3.3–1 mutant plants is their sterility. To investigate functionality of male and female gametophytes in h3.3–1, we first examined their fecundity by reciprocal crosses between wild-type (Col-0) and h3.3–1. Unlike pollination of Col-0 and h3.3–1 ovules with Col-0 pollen that can produce seeds, pollination of Col-0 and h3.3–1 ovules with pollen from h3.3–1 plants leads to failure of producing seeds (Fig. 2A, B). Further phenotypic characterization revealed that compared with the Col-0 plants, h3.3–1 displayed severely defective flowers, including smaller flower size (Fig. 2C, D), shorter filaments (Fig. 2E, F), defective anther dehiscence (Fig. 2G, H), reduced number of pollen grains (Fig. 2I, J) and defective pollen germination (Fig. 2K, L).
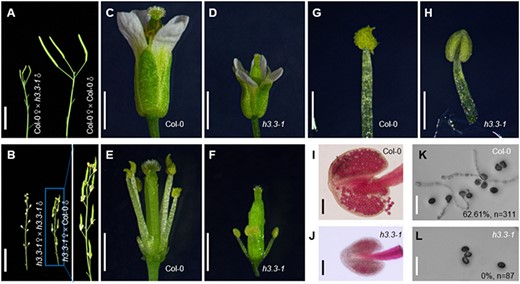
The sterility of h3.3–1 is caused by the male sterility. (A) Phenotypes of reciprocal cross\-siliques. Scale bar, 1 cm. (B) Phenotypes of reciprocal cross-siliques. Scale bar, 1 cm. (C) Morphology of representative flowers. Scale bar, 1 mm. (D) Morphology of representative flowers. Scale bar, 1 mm. (E) Morphology of representative stamens and pistils. Scale bar, 1 mm. (F) Morphology of representative stamens and pistils. Scale bar, 1 mm. (G) Morphology of representative stamens. Scale bar, 500 μm. (H) Morphology of representative stamens. Scale bar, 500 μm. (I) Morphology of representative anthers. Scale bar, 100 μm. (J) Morphology of representative anthers. Scale bar, 100 μm. (K) Representative images showing in vitro germination of pollen from Col-0. % indicates the ratio of pollen germination rate after 5 h of incubation on appropriate culture medium. n represents total numbers of analyzed pollens. Scale bars, 50 μm. (L) Representative images showing in vitro germination of pollen from h3.3–1. % indicates the ratio of pollen germination rate after 5 h of incubation on appropriate culture medium. n represents total numbers of analyzed pollens. Scale bars, 50 μm.
To examine the gametophytic effect of the male fertility problem of h3.3–1 mutants, we performed reciprocal crosses between the htr4htr5 htr8+/–/h3.3–1+/— mutant and Col-0 and tested the inheritance of h3.3 mutant alleles in heterozygous mutant plants under normal sporophytic growth. Genotyping of plants from these crosses showed that the transmission efficiency of the mutant allele is drastically impaired in the male gametophyte, for example, it was 0.21:1 compared with the expected 1:1 of normal transmission (Supplementary Table S1), while the female gametophyte transmission efficiency of the mutant allele was almost normal (Supplementary Table S1). These data indicate that the complete sterility phenotype of the knockout mutant h3.3-1 is mainly controlled by male sterility.
H3.3 is abundantly expressed in anthers and regulates microgametogenesis
To dissect the role of H3.3 in regulating male sterility, we examined the expression pattern of H3.3 genes in Arabidopsis using pHTR4-HTR4-GUS, pHTR5-HTR5-GUS and pHTR8-HTR8-GUS reporters (Fig. 3A). In 5-day-old seedlings, we found that HTR4, HTR5 and HTR8 are broadly expressed and enriched in root tips and leaf veins (Fig. 3B). In inflorescences, HTR4, HTR5 and HTR8 are abundantly expressed in anthers including sporogenous cells and pollen, while HTR4 is also expressed in filaments and stigmas (Fig. 3B, C). The results showed that H3.3 is abundantly expressed in anthers, which is in line with its effects on anther development.
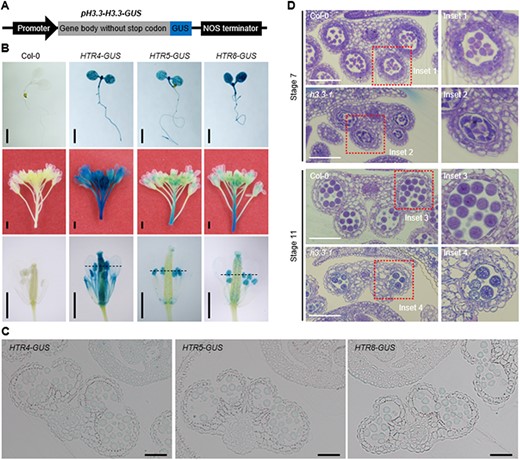
H3.3 is abundantly expressed in anther and regulates microgametogenesis. (A) Schematic representation of the pH3.3-H3.3-GUS constructs, including pHTR4-HTR4-GUS, pHTR5-HTR5-GUS and pHTR8-HTR8-GUS. (B) Histochemical localization of HTR4-GUS, HTR5-GUS and HTR8-GUS activities in transgenic plants. Top, 5-day-old seedlings; middle, inflorescences; bottom, flowers. Scale bars, 1 mm. (C) Transverse sections of anther lobes in pHTR4-HTR4-GUS, pHTR5-HTR5-GUS and pHTR8-HTR8-GUS transgenic plants indicated in Fig. 3B. Scale bars, 50 μm. (D) Transverse sections of Col-0 and h3.3–1 anther lobes at stage 7 and stage 11. Scale bars, 50 μm.
To further examine the anther developmental defects in h3.3–1 mutant plants, we prepared transverse sections of anthers from h3.3–1 and Col-0. At stage 7, the tetrads of four haploid microspores were normally organized, while the tapetal cells were abnormally organized in h3.3–1 anthers (Fig. 3D). At stage 11, one or more of the four locules were lost and the pollen was reduced in the phenotypically abnormal h3.3–1 anthers (Fig. 3D). These data indicate that the defects in the male gametes in h3.3–1 mutants occur mainly during microgametogenesis, after meiosis.
The downregulated genes in the h3.3-1 mutant are bound by H3.3 in the inflorescences
To elucidate the molecular mechanisms by which H3.3 regulates male gamete development, we first examined the relationship between H3.3 and gene expression levels in inflorescences. We divided protein-coding genes into eight classes according to their expression levels in Col-0 inflorescence (Supplementary Fig. S3A) and analyzed the relationship between H3.3 and gene expression levels in inflorescences using RNA-seq (this study) and ChIP-seq data (Zhong et al. 2022). Unlike H3.1, which distributes over gene bodies and did not correlate with the gene expression level (Supplementary Fig. S4), H3.3 is mostly highly enriched at the 3ʹ end of genes and largely correlated positively with gene expression (Fig. 4A). Moreover, a total of 3345 genes are differentially expressed in h3.3–1, with 2348 genes downregulated including HTR4, HTR5 and HTR8 and 997 genes upregulated (fold change >2 and adjusted P-value < 0.05) (Fig. 4B, Supplementary Fig. S3B). Given that H3.3 predominantly correlates positively with gene expression and the majority of misexpressed genes in h3.3–1 are downregulated, we asked if these 2348 downregulated genes in the h3.3–1 mutant are true targets of H3.3. To this end, we analyzed the ChIP-seq data. We uncovered 15,495 genes bound by H3.3. Among these H3.3-enriched genes, 1087 genes are also downregulated in h3.3–1 mutant (Fig. 4C). These 1087 H3.3 target genes overlap significantly (P-value < 0.05 by hypergeometric distribution) with genes downregulated in the h3.3–1 mutant or genes bound by H3.3 (Fig. 4C), and one of H3.3 direct targets is representatively shown in Fig. 4D. We then focus on these 1087 H3.3-bound and downregulated genes in h3.3–1 in the following analysis.
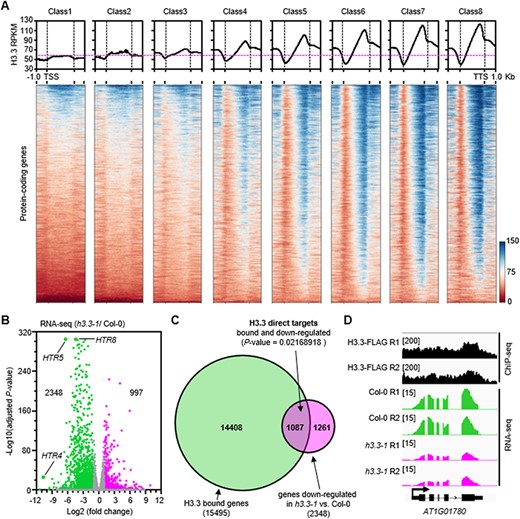
H3.3 binds to the downregulated genes in h3.3–1 mutants. (A) The correlation of H3.3 enrichment and gene expression levels in Arabidopsis inflorescences. Metaplots and heatmaps of Arabidopsis H3.3 levels centered on protein-coding genes according to the classification of genes Supplementary Fig. S3A). (B) Volcano plot presenting gene expression changes following the knockout of H3.3. Differentially expressed genes were defined as a fold of change>2 and an adjusted P-value < 0.05. (C) Intersection of H3.3-bound genes and the downregulated genes in h3.3–1 versus wild-type Col-0. Statistical significance was determined by hypergeometric distribution. (D) A gene example AT1G01780 where the H3.3 locates at the 3ʹ end of the gene-coding region and its expression is downregulated in h3.3–1.
The H3.3-targeted genes are enriched with male gamete development–related genes
To investigate the functional enrichment of the 1087 targets of H3.3, a Gene Ontology (GO) term analysis was performed using agriGO (Tian et al. 2017). With the exception of 23 genes that are not annotated in agriGO (Fig. 5A), the remaining 1064 target genes displayed a significant enrichment (P-value ≤ 0.01) (Supplementary Table S2) of 18 biological process categories associated with reproductive processes, especially male gamete development processes (108 genes), including (i) pollen tube growth, (ii) pollen tube development, (iii) pollen germination, (iv) regulation of pollen tube growth, (v) pollen sperm cell differentiation, (vi) pollen development, (vii) microgametogenesis and (viii) male gamete generation (Fig. 5B). For example, seven of the H3.3 target genes were shown to be the targets of DUO1, a male germ cell–specific R2R3 MYB transcription factor necessary for male gamete development (Rotman et al. 2005). These genes include AtMGH3, DAZ1, GCS1/HAP2, MAPKKK20, OPT8, TIP5;1 and PCR11 (Borg et al. 2011), and several of them have been shown to be essential for male gamete development (Okada et al. 2005, Borg et al. 2014, 2020, Zhu et al. 2023). Consistent with this, the deletion of H3.3 in the h3.3–1 mutant indeed caused a reduced number of pollen grains (Fig. 2I, J) and defective pollen germination (Fig. 2K, L). Four representative microgametogenesis-associated genes are shown in Fig. 5C. These results indicated that the transcription activity related to histone variant H3.3 safeguards the male gamete development through promoting the expression of its direct targets.
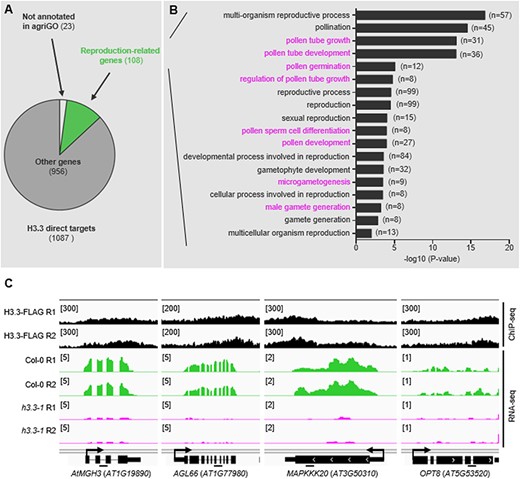
Biological processes of the H3.3 targets. (A) GO analysis of 1087 H3.3 direct targets. Among them, 108 genes were associated with reproductive processes. (B) Eighteen reproductive processes associated terms are listed. Male gamete–associated processes were highlighted. (C) Four microgametogenesis-associated H3.3 direct targets are represented. IGV snapshots presenting the normalized read densities of AtMGH3, AGL66, MAPKKK20 and OPT8 based on ChIP-seq and RNA-seq data. Bars under the gene diagram represent regions amplified by ChIP-qPCRs in Fig. 6G.
Histone H3.3 and ASF1A/1B-HIRA functionally associate during male gamete development
Histone variants contribute to chromatin dynamics through the timing and sites of their incorporation, promoted by dedicated histone chaperones (Filipescu et al. 2013). In Arabidopsis, the deposition of H3.3 is related to histone chaperones ASF1, HIRA and ATRX (Duc et al. 2015, 2017, Zhong et al. 2022). To determine whether the regulation of male gamete development is dependent upon histone variant H3.3 chaperone–dependent H3.3 deposition, we identified and analyzed the phenotype of asf1a1b, hira-1 and atrx-1 mutants (Supplementary Fig. S5A, B). The siliques of asf1a1b and hira-1 were shorter than that of Col-0 (Fig. 6A, Supplementary Fig. S5B), and the seed setting rates were reduced in asf1a1b and hira-1 mutants, while atrx-1 showed no obvious abnormalities compared with Col-0 (Fig. 6A, B). The floret organ structures of mutants were similar to that in Col-0 (Fig. 6C), while the pollen number within anthers in asf1a1b and hira-1 was obviously reduced as revealed by Alexander staining (Fig. 6D). We then performed quantitative RT-PCRs to test the expression of several random selected H3.3 targets in atrx-1, hira-1 and asf1a1b mutants. Consistent with the phenotype, the expression of all the four tested H3.3 target genes (Fig. 5C), including AtMGH3, AGL66, MAPKKK20 and OPT8, is reduced significantly in asf1a1b and hira-1, while only the expressions of AtMGH3 and AGL66 are affected in atrx-1 (Fig. 6E). To test the effects of hira-1 and asf1a1b on the deposition of H3.3, we analyzed HTR4-GFP occupancy by GFP-ChIP in asf1a1b and hira-1 mutant and found that the HTR4-GFP abundances were decreased at AtMGH3, AGL66, MAPKKK20 and OPT8 (Fig. 6F, G). These results indicated a role for ASF1A/1B-HIRA-dependent H3.3 deposition in the regulation of male gamete development.
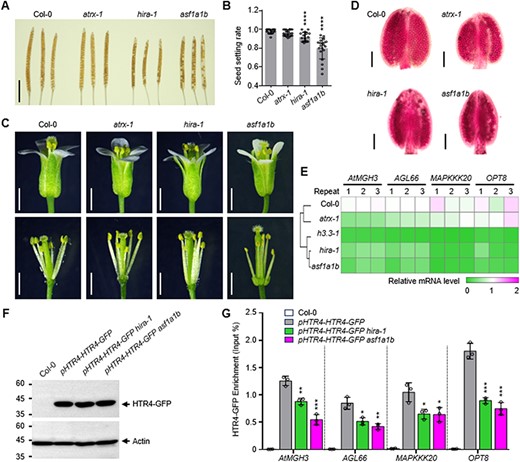
The effects of H3.3 chaperone ASF1A/1B, HIRA and ATRX on Arabidopsis microgamete development. (A) Mature siliques collected prior to dehiscence and cleared in 70% ethanol for several days to reveal seeds. Scale bar: 5 mm. (B) Seed setting rate per silique. n ≥ 16 siliques from ≥3 plants for Col-0, atrx-1, hira-1 and asf1a1b mutants. Statistical significance relative to Col-0 was determined by two-tailed Student’s t test (****P < 0.01). (C) Morphology of representative flowers, stamens and pistils of Col-0, atrx-1, hira-1 and asf1a1b mutants. Scale bar, 1 mm. (D) Morphology of representative anthers of Col-0, atrx-1, hira-1 and asf1a1b mutants. Scale bar, 200 μm. (E) The transcription levels of several microgametogenesis-associated genes in Col-0 and atrx-1, hira-1 and asf1a1b mutants. (F) Global levels of HTR4-GFP in Col-0, pHTR4-HTR4-GFP, pHTR4-HTR4-GFP hira-1 and pHTR4-HTR4-GFP asf1a1b plants. Total protein extracts from young inflorescences were analyzed by protein immunoblots using specific antibodies that recognize GFP or actin as indicated. (G) ChIP analysis of HTR4-GFP deposition at specific genes in young inflorescences of Col-0, pHTR4-HTR4-GFP, pHTR4-HTR4-GFP hira-1 and pHTR4-HTR4-GFP asf1a1b plants. ChIP samples were analyzed by quantitative PCRs on the region represented in Fig. 5C of each gene. Statistical significance of the HTR4-GFP level in pHTR4-HTR4-GFP was determined by two-tailed Student’s t test (*P < 0.05, **P < 0.01, ***P < 0.001).
Discussion
Based on the data presented herein and previous studies (Zhu et al. 2011b, Nie et al. 2014, Zhong et al. 2022), we propose a model in which the cytoplasmic synthesized H3.3 in inflorescence is translocated into the nucleus with the help of histone chaperone ASF1A/1B, and then H3.3 was incorporated into the 3ʹ end of its target genes by histone chaperone HIRA and promotes the expression of the target genes, thus safeguarding microgamete development (Fig. 7).
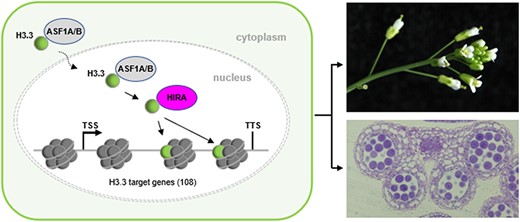
A model for the role of H3.3 in governing Arabidopsis microgamete development. The cytoplasmic synthesized H3.3 in inflorescence is translocated into the nucleus with the help of histone chaperone ASF1A/1B, and then H3.3 is deposited to the 3ʹ end of its target genes by histone chaperone HIRA and promotes the expression of the target genes, thus safeguarding the microgamete development.
Gametogenesis gives rise to highly specialized cells that function to shuttle a haploid paternal genome for the offspring, and epigenetic marks play important roles in this process (Filipescu et al. 2013). In Arabidopsis, three genes (HTR4, HTR5 and HTR8) encode the exact same protein H3.3 that differs from the replicative H3.1 by only four amino acids. H3.3 is necessary for fertility because h3.3–1 mutant plants are viable but sterile (Fig. 1D). Our study indicated that at least four defects contribute to h3.3–1 sterility: first, short stamen filaments that affect pollination of the stigma; second, anther dehiscence defects; third, the drastically decreased number of pollens and fourth, the defect in pollen germination. Consistent with this, the inheritance of h3.3 mutant alleles is lower in heterozygous mutant plants (Supplemental Table 1) (Wollmann et al. 2017). In animals, the deletion of Xenopus H3.3 affects late gastrulation development (Szenker et al. 2012, Sitbon et al. 2020). Without H3.3, Drosophila can survive, but both males and females are sterile (Hodl and Basler 2009, Sakai et al. 2009), while, H3.3 deficiency in mouse leads to early embryonic lethality (Jang et al. 2015). The viable but sterile phenotype of h3.3–1 in Arabidopsis appears to be similar to that in Drosophila, but the ovules of h3.3–1 mutant plants are fertile when pollinated with wild-type pollens. In summary, H3.3 has evolved relatively conserved but not identical functions in different species.
Developmental transition and cell fate decision are key processes of a lifecycle and are accompanied by a large number of changes in gene expression. Nuclear transfer experiments revealed that H3.3 is important for maintaining the expression of genes that do not undergo reprograming and the induction of pluripotency genes in these processes (Ng and Gurdon 2008, Nashun et al. 2011, Jullien et al. 2012). The transcriptome analysis indicated that H3.3 is associated with transcriptional activation in inflorescences and seedlings (Wollmann et al. 2017), especially peaking at the 3ʹ end of genes (Stroud et al. 2012, Wollmann et al. 2012, Zhao et al. 2022). A recent study showed that H3.3 was enriched at both the 5ʹ end and the 3ʹ end of genes in mature seeds, and the majority of the misexpressed genes were upregulated in h3.3ko (Zhao et al. 2022), suggesting that H3.3 has different distributions and functions at different developmental periods, tissues or cell types in plants. The replacement variant H3.3-containing nucleosome has been proposed to be more dynamic compared to nucleosomes with replication-dependent histone H3.1, thereby providing DNA accessibility to the transcription machinery at the regulatory elements. Indeed, H3.3 is enriched at active regulatory elements such as promoters and enhancers in mammalian genomes and loss of H3.3 results in reduced chromatin accessibility and transcription factor binding at promoters of expressed genes in mouse embryonic stem cells (Tafessu et al. 2023). However, in h3.3ko mature seeds and in the inflorescences of asf1a1b and hira-1 mutants, the chromatin accessibility at the 3ʹ-end was increased (Zhao et al. 2022, Zhong et al. 2022). Further studies are still needed to clarify the cell type–specific function of H3.3 in the regulation of gene expression in plants.
H3.3 is conserved in rice and maize (Shi et al. 2011). Knockout of H3.3 caused Arabidopsis male sterility, while transmission of the mutant allele was impaired in the male gametophyte in the h3.3–1+/— mutant (Supplemental Table 1) (Wollmann et al. 2017). Male sterility provides powerful tools in hybrid technologies and is of longstanding interest in crop breeding (Ouyang and Zhang 2013, Chen and Liu 2014). Although the loss of function of H3.3 has effects on seed germination, flowering time and plant size, the crucial function of H3.3 in male sterility in Arabidopsis makes it a candidate target for crop breeding.
Materials and Methods
Plant materials and growth conditions
All the Arabidopsis (Arabidopsis thaliana) plants used in this study are in the Columbia ecotype background (Col-0). The htr5-4 (SALK_042781), htr8-3 (SALK_087850), hira-1 (WiscDsLox362H05) and atrx-1 (SALK_025687) mutants were obtained from the Arabidopsis Biological Resource Center stock center at Ohio State University. asf1a1b double mutant (Zhu et al. 2011b) was a gift from Prof. Aiwu Dong. htr4htr5 htr8 (h3.3–1) mutants were generated by crossing htr4htr5-1 with htr8-3. All the mutants were confirmed by PCR with primers listed in Supplementary Table S3. Plant materials were grown on agar plates containing 1/2 Murashige and Skoog medium supplemented with 1% sucrose and 0.9% agar or on soil under long-day (LD) conditions (16 h light: 8 h dark, 22°C).
Constructs and transgenic plants
For pHTR4-HTR4-GUS, pHTR5-HTR5-GUS and pHTR8-HTR8-GUS, the 1457-bp, 3265-bp and 2006-bp sequences of HTR4, HTR5 and HTR8 including the promoter and gene body without stop codon were fused to the GUS coding sequence and introduced into the pBI101 vector to generate the pHTR4-HTR4-GUS, pHTR5-HTR5-GUS and pHTR8-HTR8-GUS constructs. For pHTR4-HTR4-GFP, the 1457-bp sequence of HTR4 including the promoter and gene body without stop codon was fused to the GFP coding sequence and introduced into the pC131-N1-GFP vector to generate the pHTR4-HTR4-GFP construct. Primers used for the constructs are listed in Supplementary Table S3. These constructs were confirmed by sequencing and introduced into Agrobacterium tumefaciens strain GV3101 by electroporation. All transgenic plants were generated by A. tumefaciens–mediated transformation using the floral dip method (Clough and Bent 1998).
GUS staining
The 5-day-old seedlings and inflorescences of pHTR4-HTR4-GUS, pHTR5-HTR5-GUS and pHTR8-HTR8-GUS lines were harvested and fixed in 90% acetone at 4°C overnight. Then, the materials were washed with distilled water at least three times and stained with X-Gluc substrate (5-bromo-4-chloro-3-indolyl-b-d-glucuronic acid, 2 mM final concentration) at 37°C overnight. The substrate was washed away with distilled water, and the samples were decolorated with 70% EtOH until completely white as described previously (Sun et al. 2022).
Semi-thin section examination
Inflorescences of Col-0 and h3.3–1 mutant plants were collected and fixed in Formalin-Aceto-Alcohol solution (38% formaldehyde:acetic acid:70% ethanol = 1:1:18) with 5 ml of glycerol overnight at 4°. Then, the samples were embedded in Technovit 7100 resin (Hereaus Kulzer, Hanau, Germany) after being dehydrated in a gradient series of ethanol (70%, 80%, 95% and 100%) and being polymerized at 45°C. The Technovit 7100 resin–embedded blocks were sectioned to a thickness of 2.0-µm transverse sections using an LKB Ultrotome III (LKB Bromma, Bromma, Sweden) with a glass knife. Sections were stained with toluidine blue O and photographed using the Eclipse Ni-E microscope after drying as described previously (Shi et al. 2022).
Real-time qPCR and RNA-seq library preparation
Total RNA was extracted using the TRIzol reagent (Ambion, Austin, Texas, USA) according to the manufacture’s instructions. For real-time qPCR, the RNA was quantitated using a Nabi UV/Vis Nano Spectrophotometer (MicroDigital, Pohang, Korea) and used to synthesize cDNA using TransScript One-Step gDNA Removal and cDNA Synthesis SuperMix (TransGen). Real-time qPCR was performed using TransStar Top Green qPCR SuperMix (TransGen) with a Real-time System (Bio-Rad, Hercules, California, USA). ACTIN2 (ACT2) was used as a reference gene to normalize the expression data. For RNA-seq, we first identified h3.3–1 mutant plants from the offspring of h3.3–1± by PCR and then collected young inflorescences from 15–20 plants at the 4- to 5-week-old stage (Zhong et al. 2022) as one sample. RNA purity and quantification were evaluated using the NanoDrop 2000 spectrophotometer (Thermo Scientific, Waltham, MA, USA). RNA integrity was assessed using the Agilent 2100 Bioanalyzer (Agilent Technologies, Santa Clara, California, USA). Then, the libraries were constructed using the VAHTS Universal V6 RNA-seq Library Prep Kit according to the manufacturer’s instructions. Transcriptome sequencing was conducted by OE Biotech Co., Ltd. (Shanghai, China).
Chromatin immunoprecipitation assays
Chromatin immunoprecipitation (ChIP) assays were performed as described previously (Saleh et al. 2008). Briefly, 2 g of young inflorescences were collected from 4- to 5-week-old plants and fixed with 1% formaldehyde-containing nucleus isolation buffer. The crossing reaction was terminated using fresh-prepared glycine. The nuclei were extracted, then sheared via Bioruptor Plus and immunoprecipitated with the Anti-GFP mAb-Magnetic beads (Medical & Biological Laboratories, Nagano, Japan) at 4°C overnight. After washing, reverse crosslinking was performed at 65°C overnight. The protein-DNA was treated with Protease K at 45°C for 1.5 h. The DNA was purified and precipitated with 3 M sodium acetate, glycogen and ethanol at −20°C overnight. The precipitated DNA was used for ChIP-qPCR.
Sequencing data analysis
For RNA-seq, reads were mapped to the TAIR10 genome using hisat2 and counted by featureCounts. The aligned reads files Binary sequence Alignment/Map (BAM) were converted to normalized coverage files (bigWig) with 5-bp bins using bamCoverage from deepTools (Ramirez et al. 2016). Snapshots of the data were constructed using the Integrative Genomics Viewer (IGV) (Robinson et al. 2011). Differential expression gene analysis was performed using DESeq2 (Love et al. 2014). For ChIP-seq of the data (Zhong et al. 2022), low-quality and adapter sequences were trimmed from the reads using trim_galore. Then, the reads were mapped to the A. thaliana TAIR10 genome using Bowtie2. Samtools was used to transfer the mapping results from Sequence Alignment/Map (SAM) to position-sorted SAM format. Next, the duplicated reads were removed by rmdup from Samtools. The aligned reads files (BAM) were converted to normalized coverage files (bigWig) with 5-bp bins using bamCoverage from deepTools. Snapshots of the data were constructed using the IGV. Peaks were called using MACS2 callpeak with default parameters.
Supplementary Data
Supplementary Data are available at PCP online.
Data Availability
Sequence data of the genes used in this article can be found at The Arabidopsis Information Resource public database under the following accession numbers: HTR4 (AT4G40030), HTR5 (AT4G40040) and HTR8 (AT5G10980). The RNA-seq datasets have been deposited to National Center for Biotechnology Information (NCBI) under accession number PRJNA980294. The data for ChIP-seq (GSE188493) were downloaded from Zhong et al. (2022).
Funding
The National Science Foundation of China (31871230 and 32170585 to Y.F.).
Acknowledgments
We thank Dr. Aiwu Dong for providing the asf1a1b mutant.
Author Contributions
K.L. and Y.F. designed the research; K.L. performed the experiments and analyzed the data; W.Y., C.Y., M.M., Y.W. and P.W. helped to identify mutants. K.L. and Y. F. wrote the paper.
Disclosures
The authors have no conflicts of interest to declare.