-
PDF
- Split View
-
Views
-
Cite
Cite
Machiko Watari, Mariko Kato, Romain Blanc-Mathieu, Tomohiko Tsuge, Hiroyuki Ogata, Takashi Aoyama, Functional Differentiation among the Arabidopsis Phosphatidylinositol 4-Phosphate 5-Kinase Genes PIP5K1, PIP5K2 and PIP5K3, Plant and Cell Physiology, Volume 63, Issue 5, May 2022, Pages 635–648, https://doi.org/10.1093/pcp/pcac025
- Share Icon Share
Abstract
Phosphatidylinositol 4-phosphate 5-kinase (PIP5K) is involved in regulating various cellular processes through the signaling function of its product, phosphatidylinositol (4,5)-bisphosphate. Higher plants encode a large number of PIP5Ks forming distinct clades in their molecular phylogenetic tree. Although biological functions of PIP5K genes have been analyzed intensively in Arabidopsis thaliana, it remains unclear how those functions differ across clades of paralogs. We performed comparative functional analysis of the Arabidopsis genes encoding PIP5K1, PIP5K2 and PIP5K3, of which the first two and the last belong to closely related but distinct clades, to clarify their conserved and/or differentiated functions. Genetic analysis with their single and multiple mutants revealed that PIP5K1 and PIP5K3 have non-overlapping functions, with the former in total plant growth and the latter in root hair elongation, whereas PIP5K2 redundantly functions in both phenomena. This pattern of functional redundancy is explainable in terms of the overlapping pattern of their promoter activities. In transformation rescue experiments, PIP5K3 promoter-directed PIP5K1-YFP completely rescued the short-root-hair phenotype of pip5k3. However, PIP5K3-YFP could substitute for PIP5K1-YFP only partially in rescuing the severe dwarfism of pip5k1pip5k2 when directed by the PIP5K1 promoter. Phylogenetic analysis of angiosperm PIP5Ks revealed that PIP5K3 orthologs have a faster rate of diversification in their amino-acid sequences compared with PIP5K1/2 orthologs after they arose through a eudicot-specific duplication event. These findings suggest that PIP5K3 specialized to promote root hair elongation and lost some of the protein-encoded functions retained by PIP5K1 and PIP5K2, whereas PIP5K1 differentiated from PIP5K2 only in its promoter-directed expression pattern.
Introduction
Phosphatidylinositol (4,5)-bisphosphate [PtdIns(4,5)P2], a phosphoinositide serving as not only a lipid signal interacting with its effector proteins but also a substrate for the phospholipase C signaling, modulates various cellular processes including cytoskeletal organization, membrane trafficking and signal transduction for gene expression (Di Paolo and De Camilli 2006, Krauss and Haucke 2007a, Michell 2008, Sun et al. 2013, Munnik 2014, Kolay et al. 2016, Bill and Vines 2020). The spatiotemporal existence of PtdIns(4,5)P2 is critical for its signaling function and established basically via its metabolization on specific membrane compartments. Although the metabolic pathways of phosphoinositides are elaborately linked to one another, phosphatidylinositol 4-phosphate 5-kinase (PIP5K), which produces PtdIns(4,5)P2 by phosphorylating PtdIns(4)P, is thought to be a key enzyme responsible for the spatiotemporal pattern of PtdIns(4,5)P2 in higher plant cells, where some of the phosphoinositide metabolic pathways found in animal or fungal cells are missing (Loijens et al. 1996, Oude Weernink et al. 2004, Krauss and Haucke 2007b, van den Bout and Divecha 2009, Colin and Jaillais 2020).
Higher plants encode a large number of PIP5Ks compared with animals and fungi (Mueller-Roeber and Pical 2002, Zhang et al. 2020). Molecular phylogenetic analysis has revealed that plant PIP5Ks are divided into subfamily I and II (Zhang et al. 2020). Whereas subfamily I is not preserved throughout higher plants, subfamily II includes a plant-specific class of PIP5Ks, which are designated as type B PIP5Ks and characterized by the presence of membrane occupation and recognition nexus (MORN) motif repeats at their N termini (Supplementary Fig. S1A) (Mueller-Roeber and Pical 2002, Zhang et al. 2020). Type B PIP5Ks have diversified especially in angiosperms to form distinct clades (Mueller-Roeber and Pical 2002, Zhang et al. 2020), implying that they have evolved different functions in this lineage.
The model plant Arabidopsis thaliana encodes 11 subfamily II PIP5Ks. Of these, nine can be classified into three subgroups—PIP5K1–3, PIP5K4–6 and PIP5K7–9—belonging to different type B clades (Supplementary Fig. S1B) while PIP5K10 and PIP5K11, which lack the MORN motif repeat, are classified as type A PIP5Ks (Mueller-Roeber and Pical 2002). Arabidopsis PIP5K genes are involved in various physiological phenomena, often redundantly within a clade (Heilmann 2016, Gerth et al. 2017). For example, mutant studies of PIP5K4 and PIP5K5 have revealed their redundant involvement in promoting pollen germination and pollen tube growth (Ischebeck et al. 2008, Sousa et al. 2008). RNA interference of PIP5K6 also impairs pollen tube growth (Zhao et al. 2010). Fluorescence protein fusions of PIP5K4–6 have been found to localize to the subapical plasma membrane of growing pollen tubes (Ischebeck et al. 2008, Sousa et al. 2008, Zhao et al. 2010). These findings indicate that the PIP5K4–6 subgroup genes redundantly function in pollen tube growth. Apart from this redundant function, PIP5K4 and PIP5K6 have been shown to be involved in signal transduction for light-induced stomatal opening and the perception of the pathogen-associated molecular pattern flg22, respectively (Lee et al. 2007, Menzel et al. 2019). As for the PIP5K7–9 subgroup, PIP5K9 physically interacts with the cytosolic invertase CINV1, and its overexpression suppressed sugar-mediated root growth (Lou et al. 2007). Recently, it was reported that the pip5k7pip5k9 double mutant and pip5k7pip5k8pip5k9 triple mutant exhibited defects in root growth adaptation to polyamine treatment and hyperosmotic conditions, respectively, but did not exhibit any defects in plant growth and development under non-stressful conditions (Zarza et al. 2020, Kuroda et al. 2022). Fluorescence protein fusions of PIP5K7–9 localized uniformly to the plasma membrane of root epidermal cells (Kuroda et al. 2022). These findings indicate that the PIP5K7–9 subgroup genes are redundantly involved in cell adaptation to stressful conditions but not developmental processes involving cell polarization-related events. PIP5K10 and PIP5K11, both of which are exclusively expressed in pollen, are thought to be redundantly involved in actin cytoskeletal reorganization during pollen tube growth because their double mutant pollen tubes were hypersensitive to latrunculin B, an inhibitor of actin polymerization (Ischebeck et al. 2011).
With regard to the PIP5K1–3 subgroup genes, a function common to all genes has not been found so far. The loss of PIP5K2 function affects auxin-related phenomena including root gravitropism and lateral root development (Mei et al. 2012). The pip5k1pip5k2 double mutation causes severe dwarfism accompanied by sieve-element defects (Ischebeck et al. 2013, Tejos et al. 2014, Marhava et al. 2020). PIP5K1 and PIP5K2 are localized to the plasma membrane with an apicobasal bipolar pattern and redundantly contribute to the polar localization of PIN-FORMED auxin transporters, presumably through the PtdIns(4,5)P2 functions of promoting clathrin-mediated membrane recycling and plasma membrane domain patterning (Mei et al. 2012, Ischebeck et al. 2013, Tejos et al. 2014, Marhava et al. 2020). In contrast, PIP5K3, which produces a protein localized to the plasma membrane at elongating root hair apices and sites where root hair bulges are about to form, is involved in root hair elongation (Kusano et al. 2008, Stenzel et al. 2008). Furthermore, PIP5K3 contributes to root hair elongation together with PIP5K4, especially under phosphate-starved conditions (Wada et al. 2015). Although PIP5K3 belongs to a clade closely related with but distinct from that of PIP5K1 and PIP5K2 in the molecular phylogenetic tree of plant PIP5Ks (Zhang et al. 2020), it is unknown how the PIP5K1–3 subgroup genes differ in their expression patterns and protein functions.
In this study, we performed comparative analyses of the PIP5K1–3 subgroup genes to determine their conserved and/or differentiated functions. Genetic analysis revealed that PIP5K1 and PIP5K3 have distinct functions—total plant growth and root hair elongation, respectively—whereas PIP5K2 redundantly has both functions. This pattern of functional redundancy well coincides with the overlapping pattern of their promoter activities. In transformation rescue experiments with promoter-swapped transgenes, PIP5K1 could completely substitute for PIP5K3 in terms of protein functions, but only partial substitution could be achieved in the reverse case. Phylogenetic analysis of angiosperm type B PIP5Ks revealed that PIP5K3 orthologs have evolved faster than have PIP5K1/2 orthologs. These findings suggest that during the evolution of the PIP5K1–3 subgroup genes, PIP5K3 differentiated to specialize in promoting root hair elongation and lost some of the ancestral protein-encoded functions conserved in PIP5K1 and PIP5K2, whereas PIP5K1 and PIP5K2 differentiated from each other only in their promoter-directed expression patterns.
Results
The PIP5K1 and PIP5K3 promoters exhibited activities in mutually exclusive cell types, both of which were included in those containing the PIP5K2 promoter activity
For the comparative analysis of the PIP5K1–3 subgroup genes, we first investigated tissue-specific patterns of their promoter activities using a common experimental condition. The expression patterns of β-glucuronidase (GUS) reporter genes, PIP5K1p-GUS, PIP5K2p-GUS and PIP5K3p-GUS, each consisting of the upstream intergenic region of each gene followed by the GUS-coding sequence, were histochemically examined in young seedlings. Under the condition we used (see, Materials and methods), GUS activity from PIP5K1p-GUS and PIP5K2p-GUS was obviously detected in expanding leaves and the vascular systems of hypocotyls and cotyledons in aerial organs (Fig. 1A–C, F–H). Adding to these loci, the apical meristematic region and the distal parts of cotyledon blades exhibited strong GUS activity from PIP5K2p-GUS (Fig. 1F–H). In contrast, obvious GUS activity from PIP5K3p-GUS was not detected in aerial organs (Fig. 1K–M). In roots, GUS activity from PIP5K1p-GUS and PIP5K3p-GUS was observed in the vascular system and root hair cells, respectively, and that from PIP5K2p-GUS in both loci (Fig. 1D, E, I, J, N, O). These results are basically consistent with those obtained in previous studies while detection levels of the GUS activity are different from one another (Kusano et al. 2008, Stenzel et al. 2008, Mei et al. 2012, Ischebeck et al. 2013), and indicate that, in young seedlings, cell types with obvious activities of the PIP5K1 and PIP5K3 promoters are mutually exclusive whereas the PIP5K2 promoter is active in cell types including both of them.
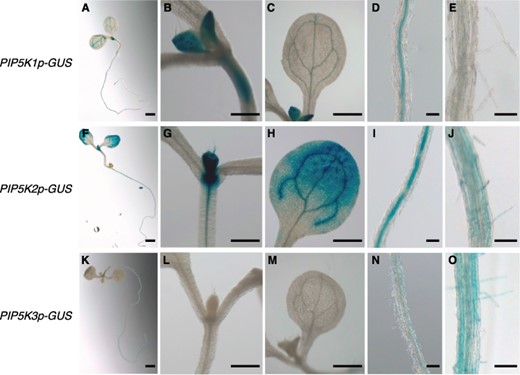
Cell type-specific pattern of promoter activity for each PIP5K1–3 subgroup gene. Promoter activities of the PIP5K1, PIP5K2, and PIP5K3 genes were histochemically analyzed using transgenic seedlings harboring the reporter genes PIP5K1p-GUS (A–E), PIP5K2p-GUS (F–J), and PIP5K3p-GUS (K–O), respectively. GUS expression patterns are shown in whole seedlings (A, F, K), shoot apices (B, G, L), cotyledons (C, H, M), and mature regions of main roots (D, I, N) at 5 d after germination, and cell differentiation regions of main roots (E, J, O) at 7 d after germination. Bars, 1 mm (A, F, K); 0.2 mm (B–E, G–J, L–O).
The loss-of-function mutation of PIP5K2 caused a moderate short-root-hair phenotype
We next comparatively investigated the functions of the PIP5K1–3 subgroup genes using their null mutants. In previous studies, the T-DNA insertion lines SALK_146728 and SALK_012487, designated here as pip5k1-1 and pip5k2-1, respectively, were analyzed as null mutants (Mei et al. 2012, Ischebeck et al. 2013, Tejos et al. 2014, Marhava et al. 2020). However, these lines were found to contain second T-DNA insertions that were co-segregated with those in the PIP5K1 and PIP5K2 genes, respectively (Supplementary Fig. S2). Hence, instead of these lines, we used the line SALK_067073, which contains T-DNA insertion in the third intron of PIP5K1 (1736 bp from the initiation codon), and the line SALK_114257, which contains T-DNA insertion in the sixth intron of PIP5K2 (2466 bp from the ATG) (Supporting Information Supplementary Fig. S3). They were confirmed to be null mutant alleles via expression analysis employing real-time RT-PCR (Supplementary Fig. S3), and designated as pip5k1-2 or pip5k1 and pip5k2-2 or pip5k2, respectively, in this study. With regard to PIP5K3, the line SALK_26683, the mutant product of which is catalytically inactive (Kusano et al. 2008, Stenzel et al. 2008), was used as the null mutant designated as pip5k3-4 or pip5k3 in this study.
From the phenotype analysis with single mutants, although no abnormalities in primary root elongation and leaf expansion were detected at the young seedling stages (Fig. 2I–L), mildly retarded growth in both pip5k1 and pip5k2 mature plants (Fig. 2Q), and reduced root hair length in pip5k3 (Fig. 3D, E) were observed as previously reported (Kusano et al. 2008, Stenzel et al. 2008, Ischebeck et al. 2013). Additionally, a mild short-root-hair phenotype was observed in pip5k2, but not in pip5k1 (Fig. 3B, C, E). The short-root-hair phenotype was rescued by the introduction of the transgene PIP5K2p-PIP5K2-YFP, which expressed the PIP5K2-YFP fusion protein using the PIP5K2 promoter, confirming that it is caused by the defect of PIP5K2 gene function (Fig. 3H, I). PIP5K2-YFP localized to the apical plasma membrane of developing root hairs in rescued lines (Fig. 3N, O) and was co-localized with PIP5K3-mCherry directed by the PIP5K3 promoter (Supplementary Fig. S4C, D), suggesting that PIP5K2 and PIP5K3 promote root hair elongation through a common molecular mechanism.
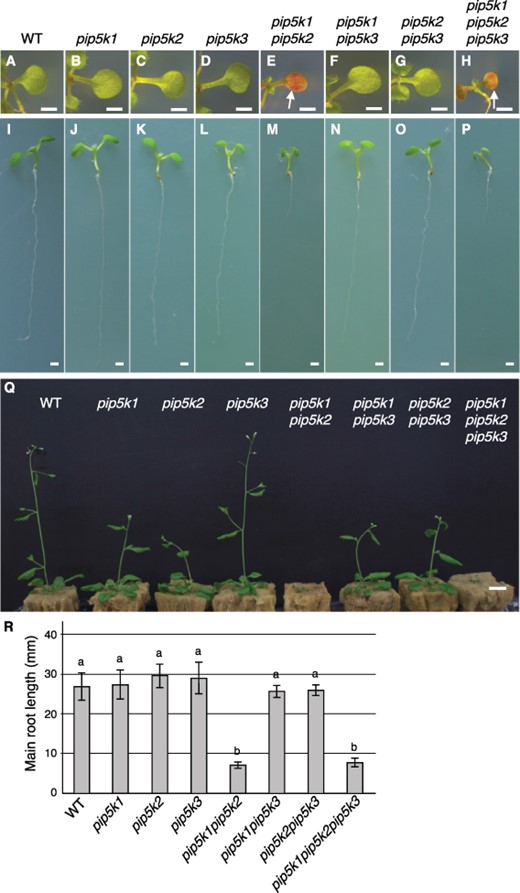
Plant growth phenotypes in single and multiple mutants of the PIP5K1–3 subgroup genes. (A–Q) Cotyledons (A–H) and whole seedlings (I–P) at 10 d after germination and mature plants (Q) at 5 weeks after germination are shown for the wild type (WT), pip5k1, pip5k2, pip5k3, pip5k1pip5k2, pip5k1pip5k3, pip5k2pip5k3, and pip5k1pip5k2pip5k3. Arrows in (E, H) indicate cotyledons accumulating anthocyanin. (R) Primary root lengths (mean ± standard deviation; n = 10) at 10 d after germination are shown for the seedlings presented in (I–P). Different letters indicate statistically significant differences (P < 0.05, ANOVA and Tukey’s test). Bars, 1 mm (A–P); 1 cm (Q).
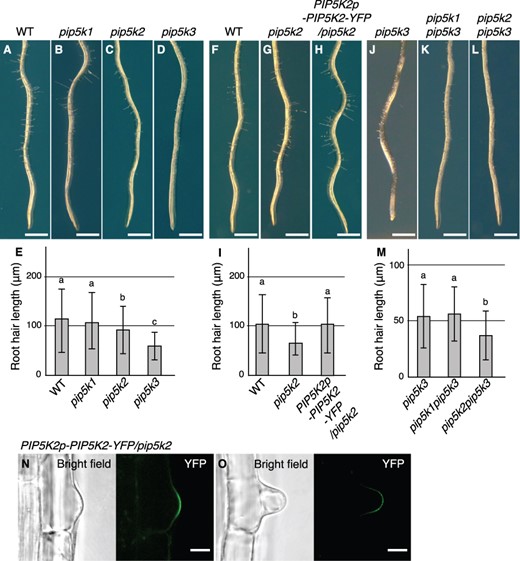
Root hair phenotypes in single and multiple mutants of the PIP5K1–3 subgroup genes. (A–D, F–H, J–L) Primary roots at 5 d after germination are shown for the wild type (WT), pip5k1, pip5k2 and pip5k3 (A–D), for the wild type, pip5k2, and a line harboring the transgene PIP5K2p-PIP5K2-YFP in the pip5k2 background (F–H), and for pip5k3, pip5k1pip5k3 and pip5k2pip5k3 (J–L). (E, I, M) Root hair lengths (mean ± standard deviation; n = 100) at 5 d after germination for the lines presented in (A–D), (F–H), and (J–L) are shown in (E), (J), and (M), respectively. Different letters indicate statistically significant differences (P < 0.05, ANOVA and Tukey’s test). (N, O) Subcellular localization of PIP5K2-YFP was observed in root hair cells harboring PIP5K2p-PIP5K2-YFP in the pip5k2 background at the bulge (N) and root hair elongation stages (O). Bright-field and YFP (green) images are shown. Bars, 1 mm (A–D, F–H, J–L); 10 μm (N, O).
PIP5K3 exhibited functional redundancy in root hair elongation with PIP5K2, but not in total plant growth with PIP5K1 or PIP5K2
To investigate functional redundancy among the PIP5K1–3 subgroup genes, we analyzed phenotypes of their multiple mutants. The pip5k1pip5k3 and pip5k2pip5k3 double-mutant mature plants exhibited mildly retarded growth with a severity similar to that observed in pip5k1 and pip5k2 single-mutant plants (Fig. 2Q). Moreover, the severe dwarf phenotype of pip5k1pip5k2, which has been reported previously (Ischebeck et al. 2013, Tejos et al. 2014), was not enhanced in the pip5k1pip5k2pip5k3 triple mutant (Fig. 2M, P, Q). Increased levels of anthocyanins, which were previously reported in pip5k1pip5k2 cotyledons and leaves (Ugalde et al. 2016), were observed in both pip5k1pip5k2 and pip5k1pip5k2pip5k3 cotyledons to a similar extent (Fig. 2E, H), and the degree of growth defects in the main roots was also similar in both mutants (Fig. 2M, P, R). These results indicate that PIP5K3 does not have redundant functions in total plant growth with PIP5K1 or PIP5K2. In contrast, the root hairs of pip5k2pip5k3, but not those of pip5k1pip5k3, were even shorter than those of pip5k3 (Fig. 3J–M), indicating that PIP5K2 and PIP5K3 are functionally redundant in promoting root hair elongation. All these results together indicate that PIP5K1 and PIP5K3 are involved in different phenomena—total plant growth and root hair elongation, respectively—whereas PIP5K2 is redundantly involved in both phenomena.
The PIP5K1-YFP fusion protein directed by the PIP5K3 promoter completely rescued the short-root-hair phenotype of pip5k3
Among the PIP5K1–3 subgroup genes, the redundant pattern of gene functions well coincides with the overlapping pattern of tissue-specific promoter activities. With regard to both their gene functions and promoter activities, PIP5K1 and PIP5K3 act in a mutually exclusive manner, whereas the activity of PIP5K2 overlaps with those of PIP5K1 and PIP5K3. This suggests that the differences in their gene functions may simply reflect the differences in their gene expression patterns, and the gene products may not necessarily differ in their protein functions. To verify this possibility, we constructed promoter-swapped genes, PIP5K3p-PIP5K1-YFP and PIP5K1p-PIP5K3-YFP, which expressed PIP5K1-YFP and PIP5K3-YFP using the PIP5K3 and PIP5K1 promoters, respectively, and examined whether the biological functions of PIP5K1 and PIP5K3 could be mutually substituted.
First, we created transgenic plants harboring PIP5K3p-PIP5K3-YFP or PIP5K3p-PIP5K1-YFP (Fig. 4A) in the pip5k3 background. In the transgenic seedlings, both PIP5K3p-PIP5K3-YFP and PIP5K3p-PIP5K1-YFP rescued the short-root-hair phenotype of pip5k3 completely (Fig. 4D–H). Moreover, PIP5K1-YFP was localized to the apical plasma membrane of developing root hairs, similar to PIP5K3-YFP in the pip5k3 background (Fig. 4I–L), and co-localized with PIP5K3-mCherry in the wild-type background (Supplementary Fig. S4E, F). These results indicate that PIP5K1 can functionally substitute for PIP5K3 in root hair elongation.
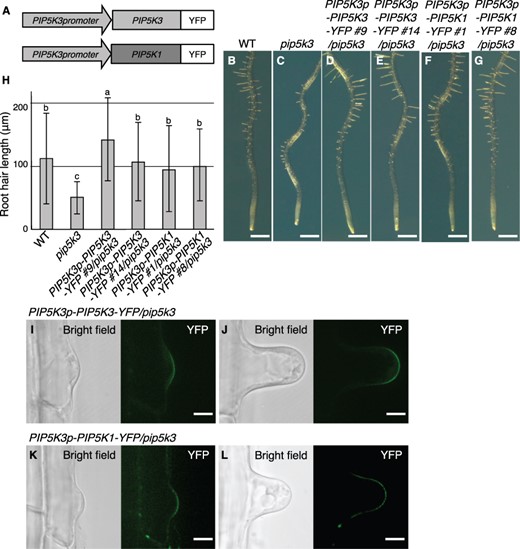
Rescue of the short-root-hair phenotype in pip5k3 by PIP5K3p-PIP5K1-YFP. (A) Structures of the transgene PIP5K3p-PIP5K3-YFP and the promoter-swapped transgene PIP5K3p-PIP5K1-YFP are schematically illustrated. (B–G) Primary roots at 5 d after germination are shown for the wild type (WT), pip5k3, and the transformation lines harboring PIP5K3p-PIP5K3-YFP (lines #9 and #14) or PIP5K3p-PIP5K1-YFP (lines #1 and #8) in the pip5k3 background. (H) Root hair lengths (mean ± standard deviation; n = 100) at 5 d after germination for the lines presented in (B–G) are shown. Different letters indicate statistically significant differences (P < 0.05, ANOVA and Tukey’s test). (I–L) Subcellular localization of PIP5K3-YFP (I, J) and PIP5K1-YFP (K, L) was observed in root hair cells harboring PIP5K3p-PIP5K3-YFP and PIP5K3p-PIP5K1-YFP, respectively, in the pip5k3 background at the bulge (I, K) and root hair elongation stages (J, L). Bright-field and YFP (green) images are shown. Bars, 1 mm (B–G); 10 μm (I–L).
The PIP5K3-YFP fusion protein directed by the PIP5K1 promoter only partially rescued the severe dwarf phenotype of pip5k1pip5k2
Next, to investigate the ability of the PIP5K3 protein to substitute for PIP5K1, we created transgenic plants harboring PIP5K1p-PIP5K1-YFP or PIP5K1p-PIP5K3-YFP (Fig. 5A) in the pip5k1 or pip5k1pip5k2 background. Both PIP5K1p-PIP5K1-YFP and PIP5K1p-PIP5K3-YFP transgenes could rescue the pip5k1 mutant phenotype of mildly retarded growth. (Supplementary Fig. S5A). However, in the pip5k1pip5k2 background, the PIP5K1p-PIP5K3-YFP transgene relieved the severe dwarfism only partially, whereas PIP5K1p-PIP5K1-YFP could rescue the phenotype completely (Fig. 5H–M and Supplementary Fig. S5B–F). The expression level of PIP5K1p-PIP5K3-YFP was comparable to that of PIP5K1p-PIP5K1-YFP in the pip5k1pip5k2 background as estimated using real-time RT-PCR (Supplementary Fig. S6). The partially rescued lines harboring PIP5K1p-PIP5K3-YFP accumulated anthocyanin in cotyledons to relatively lower extents than did pip5k1pip5k2 (Fig. 5C, F, G). These lines were intermediate between the wild type and pip5k1pip5k2 also in terms of total plant growth and main root lengths (Fig. 5H–N and Supplementary Fig. S5B–F). At a later stage, the plants exhibited a bushy phenotype with abnormal inflorescence but eventually produced a small number of viable seeds in contrast to pip5k1pip5k2 plants, which are infertile (Supplementary Fig. S5G, H). In the wild-type background, PIP5K3-YFP directed by the PIP5K1 promoter was localized to the plasma membrane of protophloem cells with a polar localization pattern similar to that of PIP5K1-YFP (Fig. 5O, P), and co-localized there with PIP5K1-mCherry (Supplementary Fig. S7C). These results suggest that PIP5K3 contains a part of PIP5K1 protein function including that related to subcellular localization.

Partial rescue of the severe dwarf phenotype in pip5k1pip5k2 by the promoter-swapped transgene PIP5K1p-PIP5K3-YFP. (A) Structures of the transgene PIP5K1p-PIP5K1-YFP and the promoter-swapped transgene PIP5K1p-PIP5K3-YFP are schematically illustrated. (B–M) Cotyledons (B–G) and whole seedlings (H–M) at 10 d after germination are shown for the wild type (WT), pip5k1pip5k2, and the transformation lines harboring PIP5K1p-PIP5K1-YFP (lines #8 and #7) or PIP5K1p-PIP5K3-YFP (lines #2 and #4) in the pip5k1pip5k2 background. Arrows in (C, F, G) indicate cotyledons accumulating anthocyanin. (N) Primary root lengths (mean ± standard deviation; n = 10) at 10 d after germination are shown for the seedlings presented in (H–M). Different letters indicate statistically significant differences (P < 0.05, ANOVA and Tukey’s test). (O, P) Localization patterns of PIP5K1-YFP (O) and PIP5K3-YFP (P) were observed in PI-stained root meristematic cells harboring PIP5K1p-PIP5K1-YFP and PIP5K1p-PIP5K3-YFP, respectively, in the wild-type background. YFP (green) and its merged images with PI (red) are shown. Asterisks and arrows indicate protophloem cell files and polarly localizing YFP fluorescence in protophloem cells, respectively. Bars, 1 mm (B–M); 10 μm (O, P).
Phylogenetic analysis of angiosperm type B PIP5Ks revealed that the amino-acid sequences of PIP5K3 orthologs likely evolved more rapidly than those of PIP5K1/2 orthologs
The results from transformation rescue experiments with promoter-swapped transgenes indicate that protein functions differ somewhat between PIP5K1 and PIP5K3. To investigate how protein functions might have differentiated over the course of evolution, we performed a phylogenetic analysis using the amino-acid sequences of type B PIP5Ks encoded by representative angiosperm genomes with a main focus on core eudicots (see Materials and Methods). Sequence data from the following species were used: Amborella trichopoda for basal angiosperms; Oryza sativa and Spirodela polyrhiza for monocots; Aquilegia coerulea, Pavaver somniferum and Nelumbo nucifera for basal eudicots; and A. thaliana, Cleome violacea, Gossypium raimondii, Citrus clementina, Populus trichocarpa, Cucumis sativus, Glycine max, Beta vulgaris, Helianthus annuus and Solanum lycopersicum for core eudicots (Supplementary Dataset S1).
The constructed phylogenetic tree (Fig. 6) consists of four major clades, designated as the PIP5K1/2/3, PIP5K4/5/6, PIP5K7/8 and PIP5K9 clades based on their harboring Arabidopsis PIP5Ks. In each clade, all examined angiosperm species have at least one PIP5K, suggesting that these four clades are conserved in angiosperms. The PIP5K1/2/3 and PIP5K4/5/6 clades include the PIP5K1/2 and PIP5K3 subclades and the PIP5K4/5 and PIP5K6 subclades, respectively, which are thought to have arisen through eudicot-specific duplication events (Zhang et al. 2020). Although each of PIP5K1/2, PIP5K4/5 and PIP5K6 clades harbors at least one PIP5K from all examined core eudicot species, the PIP5K3 clade harbors PIP5Ks from only 6 of 10 examined core eudicot species. Moreover, branches in the PIP5K3 clade are relatively longer than those in the PIP5K1/2 clade, meaning that the amino-acid sequences of the PIP5K3 orthologs had undergone a faster rate of diversification compared with those of the PIP5K1/2 orthologs.
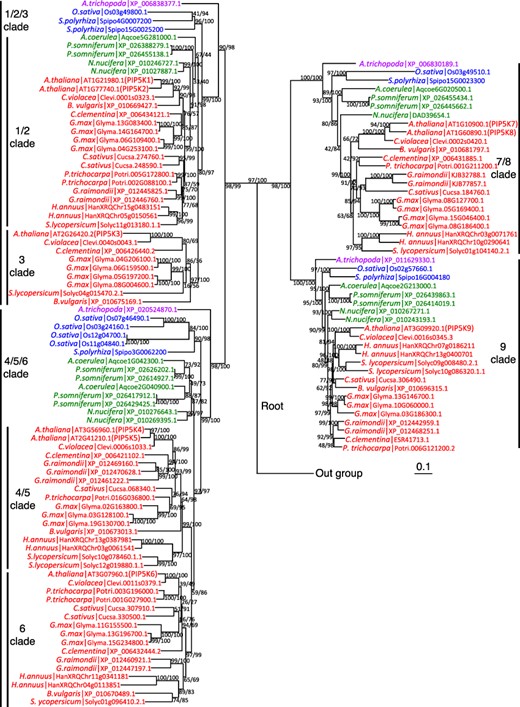
Phylogenetic tree of angiosperm type B PIP5Ks. A phylogenetic tree of type B PIP5Ks was drawn using amino-acid sequences of A. trichopoda for basal angiosperm (purple), O. sativa and S. polyrhiza for monocots (blue), A. coerulea, N. nucifera and P. somniferum for basal eudicots (green), and A. thaliana, C. violacea, G. raimondii, C. clementina, P. trichocarpa, C. sativus, G. max, B. vulgaris, H. annuus and S. lycopersicum for core eudicots (red) (for details, see Materials and Methods). Values at branches are SH-aLRT and UFBoot statistics (%) computed out of 1000 bootstrap replicates. The branch leading to the outgroup (Mamiellophyceae; B. prasinos, O. tauri and C. reinhardtii) has been cut off for representation purpose. Vertical bars indicate clades conserved in angiosperms (1/2/3, 4/5/6, 7/8 and 9) and subclades in the 1/2/3 clade (1/2 and 3) and the 4/5/6 clade (4/5 and 6). The scale bar indicates the number of substitutions per site.
Amino-acid sequences in the MORN motif repeats, first half of the catalytic domain, and activation loop are well conserved among the PIP5K1–3 subgroup proteins of core eudicots, whereas the PIP5K1/2 and PIP5K3 orthologs of core eudicots prefer different amino acids at some positions (Supplementary Fig. S8). However, in other regions, especially the variable insertion region of the catalytic domain, amino-acid sequences are well conserved in the PIP5K1/2 orthologs of core eudicots but not in the PIP5K3 orthologs. In addition, the conserved amino-acid sequences appear to be preferred by the PIP5K1–3 subgroup proteins other than those of core eudicots (Supplementary Fig. S8, Fig. 7). This suggests that PIP5K3 orthologs diversified some parts of ancestral amino-acid sequences that are conserved in PIP5K1/2 orthologs.
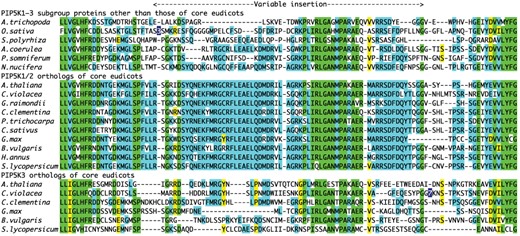
Sequence alignment of angiosperm PIP5K1–3 subgroup proteins. Amino-acid sequences of angiosperm PIP5K1–3 subgroup proteins were aligned using MAFFT L-INS-i with the BLOSUM62 scoring matrix, a gap opening penalty of 1.5, and an offset value of 0.14. The variable insertion region of the catalytic domain is shown for representative PIP5Ks: one PIP5K1/2/3 ortholog for each species other than core eudicots (A.trichopoda|XP_006838377.1, O.sativa|Os03g49800.1, S.polyrhiza|Spipo15 G0025200, A.coerulea|Aqucoe5G281000.1, P.somniferum|XP_026455138.1, N.nucifera|XP_010246727.1), one PIP5K1/2 ortholog for each core eudicot species (A.thaliana|AT1G21980.1(PIP5K1), C.violacea|Clevi.0001s0323.1, G.raimondii|XP_012445825.1, C.clementina|XP_006434121.1, P.trichocarpa|Potri.005G172800.1, C.sativus|Cucsa.248590.1, G.max|Glyma.06g109400.1, B.vulgaris|XP_010669427.1, H.annus|HanXRQChr15g0483151, S.lycopersicum|Solyc11g013180.1.1), and one PIP5K3 ortholog for each core eudicot species encoding this protein (A.thaliana|AT2G26420.2(PIP5K3), C.violacea|Clevi.0040s0043.1, C.clementina|XP_006426440.2, G.max|Glyma.08G004600.1, B.vulgaris|XP_010675169.1, and S.lycopersicum|Solyc04g015470.2.1). Amino acids conserved in more than half of the core eudicot PIP5K1/2 orthologs, those conserved in more than half of the core eudicot PIP5K3 orthologs, and those conserved in both are shaded in blue, yellow, and green, respectively. Reversed numeric characters in the amino-acid sequences indicate the number of inserted amino acids at each corresponding position. The position of the variable insertion region is indicated above the sequences.
Discussion
Differentiation of the Arabidopsis PIP5K1–3 subgroup genes in their expression patterns
This work explored functional differentiation of the PIP5K1–3 subgroup genes. Comparative analyses for gene expression and biological function revealed that the functional differentiation of the group genes well reflects their tissue-specific expression patterns deduced from their promoter activities. PIP5K1 and PIP5K3 are expressed mainly in the vascular system of various organs and specifically in root hair cells, respectively, and are involved in total plant growth and root hair elongation, respectively, whereas PIP5K2 is expressed in both loci and is involved in both phenomena. It can be said based on these findings that diversification in promoter activity significantly contributes to the functional differentiation of the PIP5K1–3 subgroup genes. During the course of evolution, the expression patterns of duplicated genes may easily differentiate through promoter diversification (Tunnacliffe et al. 2018). Moreover, the subfunctionalization model for the evolution of duplicated genes predicts that partially redundant states will occur during the process of functional divergence (Force et al. 1999, Lynch and Force 2000). Although the PIP5K2 promoter activity is apparently inclusive of both PIP5K1 and PIP5K3 promoter activities, the PIP5K1 and PIP5K3 promoters may exhibit unique activities depending on the growth conditions. Supporting this idea, the PIP5K3 promoter, but not the PIP5K2 promoter, responds to phosphate starvation to enhance root hair elongation (Wada et al. 2015).
Differentiation of the Arabidopsis PIP5K1–3 subgroup genes in their protein functions
Difference in protein function between PIP5K1 and PIP5K3 was analyzed by the transformation rescue experiments with promoter-swapped transgenes. PIP5K3p-PIP5K1-YFP completely rescued the short-root-hair phenotype of pip5K3 as well as PIP5K3p-PIP5K3-YFP. Both PIP5K1-YFP and PIP5K2-YFP exhibited the same localization pattern as PIP5K3-YFP in elongating root hairs, supporting the idea that PIP5K1 and PIP5K2 serve the same molecular function as PIP5K3 in promoting root hair elongation. Heterodimerization with endogenous PIP5K3 or a putative truncated protein product of pip5k3-4 possibly assisted with the subcellular localization of PIP5K1-YFP and PIP5K2-YFP. However, pip5k3-1 (SALK_001546), which produces transcripts encoding the dimerization domain at a much lower level than pip5k3-4 (SALK_26683), exhibited the same severity of the short-root-hair phenotype as pip5k3-4 (Kusano et al. 2008), but not as pip5k2pip5k3, implying that PIP5K2 can function in root hair elongation without the assistance of the heterodimerization. Based on these findings, it is most likely that both PIP5K1 and PIP5K2 harbor the same protein function as PIP5K3 with regard to root hair elongation.
On the other hand, PIP5K1p-PIP5K3-YFP could only partially rescue the sever dwarfism of pip5k1pip5k2, whereas PIP5K1p-PIP5K1-YFP could rescue completely. The result strongly suggests that PIP5K3 does not harbor the complete protein function of PIP5K1. PIP5Ks are thought to be different from one another in various molecular mechanisms including those for subcellular localization, protein turnover and the modulation of catalytic activity. The sequence alignment of angiosperm PIP5K1–3 subgroup proteins revealed the existence of regions where amino-acid sequences of PIP5K1/2 orthologs, but not PIP5K3 orthologs, are well conserved. These regions may be involved in molecular mechanisms contained by PIP5K1 and PIP5K2, but not by PIP5K3. In particular, the variable insertion region, which is involved in the negative regulation of catalytic activity for PIP5K6 (Hempel et al. 2017), may serve as a regulatory domain with different functions between PIP5K1 and PIP5K3. With regard to subcellular localization, although PIP5K3-YFP exhibited a polar localization pattern similar to that of PIP5K1-YFP and PIP5K2-YFP in protophloem cells in the wild-type background (Supplementary Fig. S7), the localization pattern could not be observed in the pip5k1pip5k2 background, possibly due to mutation-related abnormalities in protophloem cells. Hence, it remains possible that the polar localization of PIP5K3-YFP depends on heterodimerization with endogenous PIP5K1 or PIP5K2.
Evolutionary differentiation of angiosperm PIP5K1–3 subgroup proteins
We constructed a molecular phylogenetic tree using amino-acid sequences of type B PIP5Ks from representative species of basal angiosperms, monocots, basal eudicots and core eudicots. In the phylogenetic tree, both of the PIP5K4/5 and PIP5K6 subclades contain PIP5Ks from all examined core eudicots, but none from basal angiosperms, monocots, or basal eudicots (Fig. 6). This implies that the gene duplication event that produced these subclades occurred in the last common ancestor of core eudicots. In contrast, the PIP5K1/2 subclade contains PIP5Ks from all examined basal and core eudicots, whereas the PIP5K3 subclade contains PIP5Ks from only 6 of the 10 examined core eudicots (Fig. 6). This suggests that the gene duplication event producing these subclades occurred around the time eudicots evolved and that PIP5K3 orthologs were lost from some species including basal eudicots. However, there is room to reconsider the tree structure because of the long-branch attraction effect (Felsenstein 1978) of the PIP5K3 clade. Hence, it remains possible that the gene duplication event resulting in the PIP5K1/2 and PIP5K3 subclades also occurred in the last common ancestor of core eudicots.
The sequence alignment of angiosperm PIP5K1–3 subgroup proteins revealed the presence of regions where amino-acid sequences are well conserved in PIP5K1/2 orthologs of core eudicots but not in PIP5K3 orthologs, whereas there are no regions where amino-acid sequences are well conserved specifically in PIP5K3 orthologs. This feature is consistent with relatively longer branches of PIP5K3 orthologs than those of PIP5K1/2 orthologs in the molecular phylogenetic tree and the asymmetrical substitution ability between PIP5K1 and PIP5K3 revealed by the transformation rescue experiments. Together with the fact that the conserved amino-acid sequences in the PIP5K1/2 orthologs of core eudicots tend to be preferred by the PIP5K1–3 subgroup proteins other than those of core eudicots, it is most likely that PIP5K3 orthologs have lost some protein functions that are preserved in PIP5K1/2 orthologs.
Conclusion and Perspectives
Genetic analysis revealed that PIP5K1 and PIP5K3 have undergone subfunctionalization such that the former is involved in total plant growth and the latter in root hair elongation, whereas PIP5K2 redundantly retains both functions. This differentiation pattern is explainable in terms of the overlapping pattern of their promoter activity. Moreover, transformation rescue analysis and protein sequence analysis consistently suggest that some protein functions common to PIP5K1 and PIP5K2 are missing in PIP5K3. These findings indicate that functional differentiation of the PIP5K1–3 subgroup genes occurred in not only their expression patterns but also their protein-encoded functions, and suggest that PIP5K3 specialized to promote root hair elongation and lost functions for other purposes from its encoded protein. However, the detailed molecular mechanisms underlying the functional differentiation of the PIP5K1–3 subgroup genes have not been fully elucidated. In particular, the molecular function of each protein still needs to be related to the structures derived from conserved or differentiated amino-acid sequences. Further experimental evidence is also required to understand the evolutionary significance of each subgroup member.
Materials and Methods
Plant materials and growth conditions
Arabidopsis thaliana ecotype Columbia-0 was used as the wild type. The T-DNA insertion lines SALK_146728, SALK_067073, SALK_012487, SALK_114257 and SALK_026683, which were designated as pip5k1-1, pip5k1-2 or pip5k1, pip5k2-1, pip5k2-2 or pip5k2 and pip5k3-4 or pip5k3, respectively, in this study, were identified based on the collection of Salk Institute Genomic Analysis Laboratory collection (Alonso et al. 2003) and obtained from the Arabidopsis Biological Resource Center. Homozygous lines for T-DNA insertions were established and used in the subsequent experiments. The pip5k1pip5k2 double and pip5k1pip5k2pip5k3 triple mutant plants, which were infertile, were obtained from the progenies of pip5k1pip5k2/+ and pip5k1pip5k2/+ pip5k3 plants, respectively, via genotype screening. Arabidopsis seeds were surface-sterilized and kept at 4°C in the dark for 3 d before sowing. Seeds were sown on vertical Murashige-Skoog (MS) agar plates [MS salts (Fujifilm Wako Pure Chemical Co., Miyazaki, Japan), B5 vitamins, 2.3 mm MES-KOH (pH 5.8), 1% (v/w) sucrose and 0.8% agar]. Seedlings were grown at 22°C under continuous light conditions unless otherwise noted.
Construction of transgenes and transgenic plants
To construct the promoter-GUS reporter genes PIP5K1p-GUS, PIP5K2p-GUS and PIP5K3p-GUS, the respective upstream intergenic regions of the PIP5K1 (2583 bp), PIP5K2 (5102 bp) and PIP5K3 (978 bp) were cloned using PCR and inserted into the binary vector plasmid pBI121 (Takara Bio Clontech USA, Mountain View, CA, USA) at positions upstream of the GUS-coding sequence such that the 35S promoter region was replaced. To construct the fluorescence protein fusion genes PIP5K1p-PIP5K1-YFP, PIP5K2p-PIP5K2-YFP, PIP5K3p-PIP5K3-YFP and PIP5K3p-PIP5K3-mCherry, genomic DNA fragments encompassing upstream intergenic regions and transcribed regions including the ends of PIP5K-coding sequences were cloned using PCR and inserted together with the EYFP- or mCherry-coding sequence (Takara Bio Clontech USA) into the binary vector plasmid pHPT121 (Kusano et al. 2008) such that the fragments were located in-frame between the PIP5K-coding and fluorescence protein-coding sequences. To construct the promoter-swapped genes PIP5K3p-PIP5K1-YFP and PIP5K1p-PIP5K3-YFP, upstream intergenic regions (promoter regions) were exchanged between PIP5K1p-PIP5K1-YFP and PIP5K3p-PIP5K3-YFP. The constructed transgenes in binary vectors were introduced into Arabidopsis plants using the Agrobacterium tumefaciens-mediated vacuum infiltration method (Bechtold and Pelletier 1998). Plural homozygous transgenic lines at the T3 generation were established for each construct and examined in subsequent experiments. Supplementary Table S1 lists the sequences of the PCR primers used, and Supplementary Table S2 lists the junction sequences in the transgene constructs.
GUS histochemical analysis
GUS histochemical analysis was performed using a previously described basic procedure (Taniguchi et al. 2010). Arabidopsis seedlings were submerged in 90% acetone for 30 min on ice. After several washes with 100 mm sodium phosphate buffer (pH 7.0), samples were incubated for 12 h at 37°C in GUS staining solution [2 mm 5-bromo-4-chloro-3-indolyl-β-D-glucuronide, 0.5 mm potassium ferricyanide, 0.5 mm potassium ferrocyanide, 0.1% (w/v) Triton X-100, 10 mm EDTA-Na, 20% (v/v) methanol and 100 mm sodium phosphate buffer (pH 7.0)]. The reaction was stopped by several washes with 100 mm sodium phosphate buffer (pH 7.0). The samples were then depigmentated and fixed with FAA (50% ethanol, 10% formalin, 5% acetic acid).
Genotyping and RT-PCR
To determine the genotypes of plants bearing PIP5K mutations, genomic PCR was performed with primer sets for the detection of wild-type genes and genome-T-DNA junctions using a SimpliAmp Thermal Cycler (Thermo Fisher Scientific, Waltham, MA, USA). For RT-PCR, total RNA was first isolated with ISOGEN (Nippon Gene CO., Ltd., Tokyo, Japan) according to the manufacturer’s protocol. The total RNA was then reverse-transcribed to first-strand cDNA using a SuperScriptTM III first-Strand System for RT-PCR (Life Technologies Co., Carlsbad, CA, USA) with random hexamers as primers. Quantitative RT-PCR was performed with the cDNA and gene-specific primer sets using Lumino Ct CYBR Green qPCR ReadyMix (Sigma-Aldrich Co., St. Luis, MI, USA) and an Eco™ Real-Time PCR System (Illumina Inc., San Diego, CA, USA). RT-PCR analyses were run in triplicate for each sample to obtain the threshold cycle value. Relative transcript levels were determined using the 2−ΔΔCt method with the UBIQUITIN 4 transcript level as the internal control. Supplementary Table S1 lists the primer sets used for genomic PCR and RT-PCR.
Observation of seedlings and measurement of root hair lengths
Images of primary roots and cotyledons on agar medium were captured with a digital stereomicroscope S9i (Leica, Wetzlar, Germany). Root hairs that grew parallel to the agar surface at a distance of 4–10 mm from the root tip were measured in images of primary roots using ImageJ software (https://imagej.nih.gov/ij/). The data presented are representative of consistent results from experiments repeated at least three times.
Confocal imaging and image processing
Fluorescence images were captured using a microscope (Axiovert 200 M; Carl Zeiss, Oberkochen, Germany) equipped with a confocal laser-scanning unit (CSU-X1; Yokogawa, Tokyo, Japan). To visualize root apical meristem structures, roots were stained with 20 μg/mL propidium iodide (PI). Excitation beams were set at 488 nm for YFP and 561 nm for mCherry and PI, and detection bands were set at 505–550 nm for YFP and 580–630 nm for mCherry and PI.
Phylogenetic analysis of angiosperm PIP5Ks
Amino-acid sequences of angiosperm type B PIP5Ks were retrieved from the Arabidopsis Information Resource (https://www.arabidopsis.org/) for A. thaliana, Rice Genome Annotation Project (http://rice.plantbiology.msu.edu/) for Oryza sativa, INRA Sunflower Bioinformatics Resources (https://www.heliagene.org/) for Helianthus annuus, International Tomato Genome Sequencing Project (https://solgenomics.net/organism/Solanum_lycopersicum/genome) for Solanum lycopersicum, National Center for Biotechnology Information (https://www.ncbi.nlm.nih.gov) for Amborella trichopoda, Beta vulgaris, Citrus clementina, Gossypium raimondii, Nelumbo nucifera, and Pavaver somniferum and Phytozome (https://phytozome-next.jgi.doe.gov/) for Aquilegia coerulea, Cleome violacea, Cucumis sativus, Glycine max, Populus trichocarpa and Spirodela polyrhiza. Those for Mamiellophyceae species, Bathycoccus prasinos, Ostreococcus tauri and Chlamydomonas reinhardtii were retrieved using BLASTP search on GenomeNet (https://www.genome.jp/) and used as the outgroup. Sequences were aligned using MAFFT L-INS-i (Katoh and Standley 2013). For the construction of a phylogenic tree containing all examined angiosperm type B PIP5Ks, the alignment was automatically trimmed using trimAl with the options—gt 0.1 and—st 0.0001 (Capella-Gutiérrez et al. 2009). Trees were reconstructed using IQ-TREE multicore version 1.6.12 (Minh et al. 2020) with the mixture model LG + PMSF + G (Wang et al. 2018) and optimization of weights of the mixture model (-mwopt option). Branch support values were computed out of 1,000 bootstrap replicates in a SH-like approximate likelihood ratio test (SH-aLRT) (-alrt option) (Guindon et al. 2010) and in an ultrafast bootstrap (UFBoot) procedure (-bb option) (Minh et al. 2013, Hoang et al. 2018).
Supplementary Data
Supplementary data are available at PCP online.
Data Availability
The data that support the findings of this study are available from the corresponding author upon reasonable request.
Funding
This work was supported by Grants-in-Aid for Scientific Research (KAKENHI) from the Japanese Society for the Promotion of Science (JSPS); 21K05392 to M.K., and 21H02505 to T.A., and from the Kyoto University Foundation and ISHIZUE 2020 of Kyoto University Research Development Program to M.K.
Acknowledgements
We are grateful to Ms. Keiko Yasuda (Institute for Chemical Research, Kyoto University) for technical assistances. This work was supported by Grants-in-Aid for Scientific Research (KAKENHI) from the Japanese Society for the Promotion of Science (JSPS); 21K05392 to M.K., and 21H02505 to T.A., and from the Kyoto University Foundation and ISHIZUE 2020 of Kyoto University Research Development Program to M.K.
Author Contributions
M.W. conceived the study, designed the experiments, performed the research, analyzed the data and prepared the manuscript. M.K. and T.T. contributed to experimental design and analyzed the data. R.B.-M. and H.O. contributed to phylogenetic analysis. T.A. contributed to experimental design, data analysis and manuscript preparation and submitted the manuscript.
Disclosures
The authors have no conflicts of interest to declare.