-
PDF
- Split View
-
Views
-
Cite
Cite
Alice Lambolez, Ayako Kawamura, Tatsuya Takahashi, Bart Rymen, Akira Iwase, David S Favero, Momoko Ikeuchi, Takamasa Suzuki, Sandra Cortijo, Katja E Jaeger, Philip A Wigge, Keiko Sugimoto, Warm Temperature Promotes Shoot Regeneration in Arabidopsis thaliana, Plant and Cell Physiology, Volume 63, Issue 5, May 2022, Pages 618–634, https://doi.org/10.1093/pcp/pcac017
- Share Icon Share
Abstract
Many plants are able to regenerate upon cutting, and this process can be enhanced in vitro by incubating explants on hormone-supplemented media. While such protocols have been used for decades, little is known about the molecular details of how incubation conditions influence their efficiency. In this study, we find that warm temperature promotes both callus formation and shoot regeneration in Arabidopsis thaliana. We show that such an increase in shoot regenerative capacity at higher temperatures correlates with the enhanced expression of several regeneration-associated genes, such as CUP-SHAPED COTYLEDON 1 (CUC1) encoding a transcription factor involved in shoot meristem formation and YUCCAs (YUCs) encoding auxin biosynthesis enzymes. ChIP-sequencing analyses further reveal that histone variant H2A.Z is enriched on these loci at 17°C, while its occupancy is reduced by an increase in ambient temperature to 27°C. Moreover, we provide genetic evidence to demonstrate that H2A.Z acts as a repressor of de novo shoot organogenesis since H2A.Z-depleted mutants display enhanced shoot regeneration. This study thus uncovers a new chromatin-based mechanism that influences hormone-induced regeneration and additionally highlights incubation temperature as a key parameter for optimizing in vitro tissue culture.
Introduction
Thanks to their extensive regenerative capacities, plants can repair tissues after an injury as well as regrow organs or even entire individuals from explants (Ikeuchi et al. 2016). These phenomena underlie many agricultural techniques that have been used for thousands of years, such as propagation by cutting (Howard 1971) and grafting (Melnyk 2016). However, not all plant species have equal regenerative capabilities, which impedes the large-scale culture and engineering of some recalcitrant crops, such as walnut (Juglans regia) or oak (Quercus spp.; Pijut et al. 2011). Barriers to regeneration can sometimes be overcome by controlling and directing the regeneration process in vitro through treatment of explants with different hormones (Skoog and Miller 1957, Che et al. 2007), a process hereinafter referred to as hormone-induced regeneration. This approach, widely used in both laboratory research and horticultural practice, has led to the establishment of new propagation techniques, such as in vitro embryo culture that has dramatically improved plantain (Musa spp.) and oil palm (Elaeis guineensis) yields (Vuylsteke and Swennen 1992, Weckx et al. 2019). Moreover, regeneration on hormone-supplemented medium is a key step in Agrobacterium-mediated transformation (Gelvin 2003), the main method used to generate transgenic plants. Thus, given its scientific and economic importance, establishing optimal conditions for hormone-induced regeneration is a crucial issue and requires a thorough understanding of the molecular mechanisms underlying this process.
Hormone-induced shoot regeneration is often performed using a two-step procedure, in which explants are first incubated on auxin-rich callus-inducing medium (CIM) and subsequently transferred to shoot-inducing medium (SIM) containing a high cytokinin/auxin ratio (Feldmann and Marks 1986). Incubation on CIM triggers the formation of calli, unorganized masses of cells that undergo cellular reprogramming—defined here as the reconfiguration of cellular identity—through which they acquire regenerative competency (Ikeuchi et al. 2019). These calli exhibit several features that are also found in lateral root meristems (Sugimoto et al. 2010), including the auxin-induced and spatially organized expression of root meristem genes that appear to be necessary for the acquisition of cellular pluripotency (Kareem et al. 2015, Kim et al. 2018, Liu et al. 2018, Ikeuchi et al. 2019, Zhai and Xu 2021).
Subsequent shoot regeneration on SIM involves the conversion of these lateral root primordium-like structures into shoot-like structures. Auxin and cytokinin present in SIM permit the activation of ENHANCER OF SHOOT REGENERATION 1 (ESR1) and its downstream paralog ESR2, which both contribute to the formation of shoot promeristems (Banno et al. 2001, Ikeda et al. 2006, Matsuo et al. 2011, Iwase et al. 2017). In particular, ESR2 directly activates CUP-SHAPED COTYLEDON 1 (CUC1; Banno et al. 2001, Ikeda et al. 2006). CUC1 and its homolog CUC2 play a key role in shoot regeneration (Aida et al. 1997, Daimon et al. 2003) and are required for both development and maintenance of the shoot apical meristem (SAM; Aida et al. 1997, Gordon et al. 2007). In parallel, cytokinin contained in SIM promotes the expression of WUSCHEL (WUS), encoding a homeobox transcription factor required to initiate the formation of shoot progenitors (Gallois 2004, Gordon et al. 2007, Meng et al. 2017, Pernisova et al. 2018). Subsequent shoot meristem development is accompanied by the emergence of auxin- and cytokinin-response domains, which occupy mutually exclusive regions within the callus (Cheng et al. 2012). On one hand, SIM induces the expression of auxin biosynthesis enzymes YUCCA 1 (YUC1) and YUC4 and YUC-mediated auxin production in turn promotes SAM formation (Cheng et al. 2012). On the other hand, the domains producing cytokinin, a hormone that is required to stabilize WUS in regular SAM development (Snipes et al. 2018), are established at least in part by cytokinin biosynthesis enzymes of the ISOPENTENYLTRANSFERASE (IPT) family (Kakimoto 2001, Takei et al. 2001, Cheng et al. 2012).
While the molecular mechanisms underlying the developmental transitions that occur during regeneration are becoming increasingly clear, how environmental conditions impact these regeneration processes has not yet been thoroughly investigated (Ikeuchi et al. 2016). One of the major parameters affecting plant growth is ambient temperature, which influences numerous developmental processes such as hypocotyl and petiole growth (Gray et al. 1998, Koini et al. 2009) and timing of both flowering and silique dehiscence (Blázquez et al. 2003, Li et al. 2018). Such temperature-influenced plant development, termed thermomorphogenesis, is in part regulated at the chromatin level (Casal and Balasubramanian 2019). In particular, histone variant H2A.Z, encoded by HISTONE H2A PROTEIN 8 (HTA8), HTA9 and HTA11 in Arabidopsis thaliana (March-Díaz and Reyes 2009), is evicted from nucleosomes when plants are exposed to warm temperature (Kumar and Wigge 2010, Cortijo et al. 2017). Accordingly, constitutively H2A.Z-depleted mutants phenocopy plants grown at higher temperatures, displaying for instance long hypocotyls and petioles (Kumar and Wigge 2010). H2A.Z-containing nucleosomes are predominantly found at transcription start sites (TSSs) and on gene bodies (Kumar and Wigge 2010, Coleman-Derr and Zilberman 2012), where they are thought to prevent transcription (Thakar et al. 2010, Dai et al. 2017). This likely maintains genes in an off state that is poised for rapid activation in response to environmental cues, including temperature and light (Kumar and Wigge 2010, Coleman-Derr and Zilberman 2012, Cortijo et al. 2017, Willige et al. 2021). How H2A.Z is removed from nucleosomes in these contexts remains unclear, but recent evidence suggests that this depends on the activity of transcription factors, such as PHYTOCHROME-INTERACTING FACTOR 7 (PIF7) in response to light (Willige et al. 2021) or heat shock factors at the HEAT SHOCK PROTEIN 70 (HSP70) locus in response to heat (Cortijo et al. 2017).
Although ambient temperature is briefly mentioned to modulate the efficiency of in vitro regeneration for several crops, such as tobacco (Nicotiana tabacum), sunflower (Helianthus annuus) or carrot (Daucus carota), in a now quite dated review (Haissig 1965), no molecular mechanisms explaining how temperature affects hormone-induced regeneration have been reported so far. In this study, we show that warm temperature enhances both callus formation and subsequent shoot regeneration in Arabidopsis thaliana, and that the transcriptional events mediating this process correlate with temperature-dependent eviction of H2A.Z. Consistently, shoot regeneration is enhanced in double mutants for HTA9 and HTA11, which resemble wild-type (WT) explants grown at a higher temperature. This study thus provides new insights into the temperature-dependent regulation of hormone-induced regeneration and thereby lays a foundation for designing molecular approaches aimed at optimizing in vitro regeneration.
Results
Warm temperature accelerates hormone-induced callus formation and shoot regeneration
To first assess whether ambient temperature affects hormone-induced callus formation, we incubated hypocotyl explants from 7-day-old WT plants on CIM at 17°C, 22°C or 27°C, a temperature range that is considered not stressful for Arabidopsis thaliana (Kumar and Wigge 2010; Fig. 1A, B). While a minor but significant decrease in fresh weight at 22°C or 27°C is observed after 4 days, likely due to water loss, this trend reverts later on. After 15 days on CIM, calli grown at 27°C are significantly heavier (2.5 times more on average) than those grown at 22°C, which are themselves significantly heavier than those grown at 17°C (3.2 times more on average). This positive effect of temperature on callus formation is even more pronounced after 25 days, as 27°C-grown calli are 4.6 times heavier than those grown at 22°C.
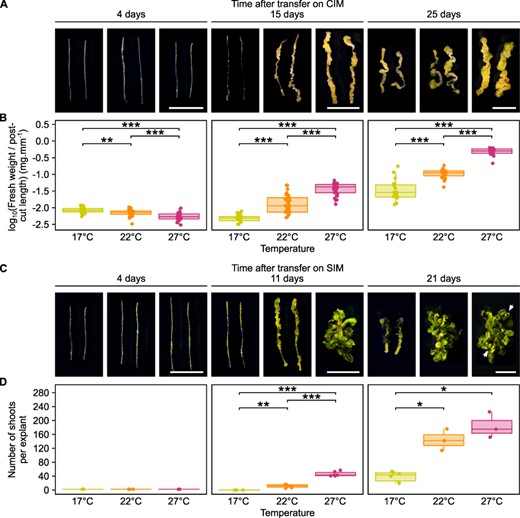
Hormone-induced regeneration efficiency increases at warmer temperatures. (A) Light microscopy images of WT hypocotyl explants incubated on CIM for 4, 15 or 25 days at 17°C, 22°C or 27°C. Scale bars = 5 mm. (B) Fresh weight of WT hypocotyl explants incubated on CIM for 4, 15 or 25 days at 17°C, 22°C or 27°C (n ≥ 17). Values were normalized to the post-cutting length of explants. *P value < 0.05. **P value < 0.01. ***P value < 0.001 (Student’s t-test). (C) Light microscopy images of WT hypocotyl explants incubated on CIM for 4 days and then on SIM for 4, 11 or 21 days at 17°C, 22°C or 27°C. White arrows indicate flower buds. Scale bars = 5 mm. (D) Number of shoots produced per WT hypocotyl explant after incubation on CIM for 4 days and then on SIM for 4, 11 or 21 days at 17°C, 22°C or 27°C (n ≥ 3). *P value < 0.05. **P value < 0.01. ***P value < 0.001 (Student’s t-test).
We next investigated how temperature affects hypocotyl explants transferred to SIM following a 4-day incubation on CIM, a stage at which calli grown at 17°C, 22°C or 27°C are morphologically indistinguishable from each other (Fig. 1). A significant increase in shoots at warmer ambient temperature was observed from 11 days after transfer to SIM, when explants cultured at 27°C display 4.1 times more shoots than explants cultured at 22°C, while none can be observed at 17°C (Fig. 1C, D). Importantly, shoots are visible on explants cultured at 17°C 21 days after transfer on SIM, revealing that low temperature does not prevent shoot regeneration per se, but rather slows it down. In particular, at the point when leaves are just starting to appear at 17°C, 27°C-grown explants already present true leaves and start producing flower buds, indicating that the shoots regenerated at 27°C have already reached more mature developmental stages (Fig. 1C). These results show that an increase in ambient temperature drastically accelerates hormone-induced regeneration in hypocotyl.
To check whether this behavior can be reproduced with other organs, we incubated root explants from 7-day-old WT plants for 21 days on CIM at either 17°C or 27°C (Supplementary Fig. S1A, B). Similarly to what we had obtained with hypocotyls (Fig. 1A, B), we observed an 18.1-fold increase in weight for explants grown at 27°C compared to those grown at 17°C (Supplementary Fig. S1A, B). Likewise, root explants incubated for 4 days on CIM then 16 days on SIM at 27°C yield on average 37.1 shoots per explant whereas root explants incubated at 17°C do not show any shoots at this time point (Supplementary Fig. S1C, D). These results show that the temperature-mediated acceleration of hormone-induced regeneration is not a hypocotyl-specific phenomenon.
As the positive influence of increased temperature on shoot regeneration could be due to its promotive effect on callus formation during CIM incubation, we tested whether ambient temperature affects shoot regeneration on SIM alone. We therefore incubated hypocotyl explants for 4 days on CIM at either 17°C or 27°C and then for 11 days on SIM at 27°C or 17°C, respectively (Supplementary Fig. S1E, F). We found that, at this time point, no shoot regeneration is observed on explants grown at 17°C on SIM, irrespective of the incubation temperature on CIM (Supplementary Fig. S1). We also observed that explants incubated continuously at 27°C display 1.3 times more shoots on average than explants grown on CIM at 17°C and then on SIM at 27°C (Supplementary Fig. S1E, F). These results reveal that, while the temperature at which the incubation on CIM is performed does have an effect on explant growth, the temperature at which the incubation on SIM is performed appears to be the major factor contributing to the increase in shoot regeneration capacity, although this could also be due to the fact that the SIM step lasted longer than the CIM step in our experimental set-up.
Considering that wounding is essential for successful shoot regeneration upon external hormone application (Iwase et al. 2015), we tested if the positive effect of temperature seen during CIM and SIM incubation is a consequence of an enhanced wound response. We thus wounded 7-day-old WT hypocotyls and allowed them to regenerate without any hormone addition at 17°C, 22°C or 27°C (Supplementary Fig. S2). We observed callus formation after wounding at all temperatures and noticed a significant increase in the number of explants forming callus at warmer temperatures 6 days after cutting (1.4-fold increase at 22°C versus 17°C and 1.6-fold increase at 27°C versus 22°C), although these differences become non-significant at 21 days (Supplementary Fig. S2A, B). Furthermore, we could not observe any clear correlation between ambient temperature and callus growth (Supplementary Fig. S2A, C), suggesting that temperature may accelerate callus formation upon wounding, but this effect is likely too mild to explain the dramatic enhancement seen during hormone-induced regeneration.
Temperature affects the expression of a great number of regeneration and hormone-associated genes
To determine which genes are responsible for the increase in callus formation and shoot regeneration efficiency at higher temperatures, we performed RNA extraction followed by high-throughput sequencing (RNA-seq) on WT hypocotyl explants cultured at either 17°C or 27°C. Samples were harvested immediately after cut, right before transfer on SIM after 4 days on CIM and after 4 days on SIM, i.e. before the emergence of the first shoots at 27°C (Fig. 2, Supplementary Fig. S3, Supplementary Table S1). By comparison with explants harvested immediately after cutting, we first found that 76.7% of genes up-regulated and 81.1% of genes down-regulated on CIM at 27°C show the same differential expression at 17°C (Supplementary Fig. S3A). Likewise, 75.3% of genes up-regulated and 80.5% of genes down-regulated on SIM at 27°C show the same differential expression at 17°C. Moreover, we noticed that a significant number of genes up-regulated (47.3%) or down-regulated (50.9%) at 27°C compared to 17°C during CIM incubation are also up-regulated or down-regulated, respectively, at 27°C during SIM incubation (Supplementary Fig. S3B). This suggests that non-stressful changes in ambient temperature tend to affect the amplitude of expression rather than the set of expressed genes, many of which are similarly regulated by exposure to CIM and SIM. In line with this hypothesis, genes up-regulated by increased temperature are significantly enriched (48.7%) with CIM- and SIM-induced genes (Fig. 2A, Supplementary Table S1), whereas genes down-regulated by warm temperature are significantly enriched (45.7%) with CIM- and SIM-repressed genes (Supplementary Fig. S3C, Supplementary Table S1). These data imply that combining an increase in temperature with the CIM/SIM protocol generates an additive effect that leads to enhanced regeneration.

Numerous regeneration genes are transcriptionally affected by warm temperature during hormone-induced regeneration. (A) Venn diagram representation of the overlap between CIM-induced genes, SIM-induced genes and thermo-induced genes. CIM-induced genes include those induced after CIM incubation at either 17°C or 27°C compared to right after cutting. SIM-induced genes include those induced after CIM + SIM incubation at either 17°C or 27°C compared to right after cutting. Thermo-induced genes include those up-regulated at 27°C compared to 17°C either after CIM or after CIM + SIM incubation. The enrichment (representation factor, hypergeometric test) of genes up-regulated at 27°C among all genes up-regulated by CIM or CIM + SIM incubation is indicated. ***P value < 0.001. (B) Top 10 GO terms enriched among genes induced by CIM and/or SIM and up-regulated at 27°C. (C) Line graphs showing the expression of IPT1, LOG3, CUC1, CUC3, ESR2, WUS, YUC1, YUC4 and SAUR15. Explants were incubated on CIM for 4 days and then on SIM for 4 days at 17°C or 27°C. HSP70 is shown as a reference temperature-induced gene (Kumar and Wigge 2010) and PROTEIN PHOSPHATASE 2A SUBUNIT A3 (PP2AA3) as a reference non-induced gene (Czechowski et al. 2005). Error bars show the standard deviation between three sequencing replicates. *P value < 0.05. **P value < 0.01. ***P value < 0.001 (Student’s t-test, n = 3).
To further investigate the genes that may play a role in enhancing regeneration at higher temperatures, we performed a Gene Ontology (GO) analysis on genes that are up-regulated at 27°C on CIM or SIM and also induced by exposure to the same or both type of media (Fig. 2B). As expected, we found a high enrichment for genes annotated with terms related to heat responses, such as ‘heat acclimation’ (Fig. 2B). More interestingly, we also found a high enrichment for genes annotated with terms related to shoot development, such as ‘secondary shoot formation’, and this GO category was represented even among genes induced by warm temperature only during CIM incubation (Fig. 2B). When performing a GO analysis on genes down-regulated by warm temperature and by CIM and/or SIM incubation (Supplementary Fig. S3D), we found a high enrichment of stress-response genes, annotated with terms such as ‘response to water deprivation’, ‘response to wounding’, ‘response to salt stress’ and ‘response to oomycetes’. This suggests that an increase in temperature may tilt the transcriptional balance between stress responses and growth pathways toward the latter.
We then investigated whether the expression of genes encoding transcription factors that are known to play a role in regeneration as well as histone modifiers and hormone-related factors that may participate in this process (Ikeuchi et al. 2017, 2019) is impacted by temperature (Supplementary Fig. S3E, F). During CIM incubation, we found that an increase in temperature promotes the expression of genes implicated in cytokinin synthesis, such as IPT1 and LONELY GUY 3 (LOG3; Fig. 2C, Supplementary Fig. S3E). On SIM, warm temperature mostly induces genes coding for transcription factors involved in shoot development, such as CUC1, CUC3, ESR2 and WUS, as well as genes involved in auxin synthesis, such as YUC1 and YUC4, and auxin response, such as SMALL AUXIN UPREGULATED 15 (SAUR15; Fig. 2C, Supplementary Fig. S3F). Notably, YUC4 is also up-regulated by warm temperature during the CIM incubation step (Fig. 2C). Altogether, these observations suggest that warm temperature affects regeneration in part by modifying the expression of transcription factor-coding genes as well as auxin- and cytokinin-related genes.
Warm temperature reduces H2A.Z occupancy on thermo-induced regeneration genes
Previous studies have shown that the alterations induced by temperature on plant development are linked with eviction of histone variant H2A.Z (Kumar and Wigge 2010, Cortijo et al. 2017). It is thus possible that a similar mechanism improves hormone-induced regeneration efficiency at warmer temperatures. To test this hypothesis, we re-analyzed a chromatin immunoprecipitation followed by high-throughput sequencing (ChIP-seq) dataset from Cortijo et al. (2017), in which the genome-wide occupancy of HTA11 was assessed in whole WT seedlings grown at 17°C and then either shifted to 27°C or maintained at 17°C for 240 min (Fig. 3, Supplementary Fig. S4, Supplementary Table S2). We found that 6,693 genes show reduced HTA11 enrichment at 27°C compared to 17°C, while 9,541 genes gain HTA11 at 27°C (Fig. 3A, Supplementary Fig. S4A, B, Supplementary Table S2). This is in accordance with the results reported in Cortijo et al. (2017) and confirms that ambient temperature affects H2A.Z occupancy on many loci.
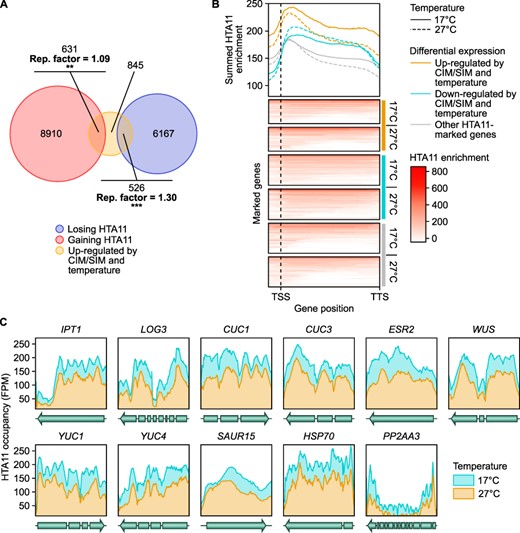
Differential regulation of gene expression at 27°C correlates with changes in H2A.Z enrichment. (A) Venn diagram representation of the overlap between thermo- and CIM/SIM-induced genes and genes with temperature-dependent HTA11 enrichment. Thermo- and CIM/SIM-induced genes include CIM- and/or SIM-induced genes that are up-regulated at 27°C compared to 17°C on either medium. Genes with temperature-dependent HTA11 enrichment include those that lose or gain HTA11 within the chromatin spanning their coding region plus 2 upstream nucleosomes (294 bp) in plants transferred to 27°C compared to plants maintained at 17°C. The enrichment (representation factor, hypergeometric test) of thermo- and CIM/SIM-induced genes among genes with temperature-dependent HTA11 enrichment is indicated. **P value < 0.01. ***P value < 0.001. (B) Heat map representation of the normalized ChIP-seq read density distribution for HTA11 within the chromatin spanning the coding region plus 2 upstream nucleosomes (294 bp) of all HTA11-marked genes in plants transferred to 27°C or plants maintained at 17°C. The position of the transcription start site (TSS) and transcription termination site (TTS) is marked. Line graphs show the summed HTA11 enrichment for HTA11-marked genes that are thermo-induced and up-regulated on CIM/SIM, HTA11-marked genes that are thermo-repressed and down-regulated on CIM/SIM and all other HTA11-marked genes. For the sake of clarity, only a subset of randomly chosen other HTA11-marked genes is shown in the heat maps, but the whole group is taken into account in the line graphs. (C) Line plots showing the HTA11 occupancy across the coding region of IPT1, LOG3, CUC1, CUC3, ESR2, WUS, YUC1, YUC4 and SAUR15. Data are shown for 240 min at 17°C or after transfer to 27°C. HSP70 is shown as a reference temperature-induced gene (Kumar and Wigge 2010) and PP2AA3 as a reference non-induced gene (Czechowski et al. 2005).
To assess whether regeneration genes are impacted by such changes in H2A.Z occupancy, we first compared the CIM- and/or SIM-induced genes up-regulated by temperature (Fig. 2A) to genes showing modified HTA11 enrichment with an increase in temperature. We found that a significant proportion (26.3%) of these up-regulated genes lose HTA11 at 27°C, while another significant proportion (31.5%) gains HTA11 (Fig. 3A). Surprisingly, performing the same comparison with CIM- and/or SIM-repressed genes down-regulated by temperature (Supplementary Fig. S3C) returned similar results: 22.8% of down-regulated genes lose HTA11 after transfer to 27°C, while 40.9% gain HTA11 (Supplementary Fig. S4B). As these results solely reflect the presence or absence of differential HTA11 enrichment at any location on the gene body, we next investigated the correlation between differential expression and HTA11 dynamics in a more quantitative and position-specific manner. To this end, we looked at the summed HTA11 enrichment on CIM/SIM-induced genes up-regulated at 27°C, CIM/SIM-repressed genes down-regulated at 27°C, as well as all other HTA11-marked genes (Fig. 3B). We found that up-regulated and down-regulated genes display an overall higher enrichment of HTA11 compared to other HTA11-marked genes, which likely makes them more transcriptionally responsive to changes in H2A.Z occupancy (Fig. 3B). Moreover, genes down-regulated at 27°C exhibit an overall higher HTA11 occupancy at 27°C than at 17°C, whereas HTA11 enrichment levels are lower at 27°C compared to 17°C on the gene body of up-regulated genes (Fig. 3B). In particular, all nine genes associated with regeneration that are strongly up-regulated at 27°C on CIM and/or SIM (Fig. 2C) exhibit decreased HTA11 levels at this temperature, similar to HSP70 which is induced both by temperature and loss of H2A.Z (Kumar and Wigge 2010; Fig. 3C). This correlation between thermosensitive changes in HTA11 enrichment and differential gene expression suggests that loss of H2A.Z caused by an increase in ambient temperature could contribute to the induction of key genes during in vitro regeneration. Therefore, H2A.Z appears to mainly contribute to the regulation of hormone-induced regeneration by repressing gene expression, in accordance with previous results obtained in other developmental contexts (Thakar et al. 2010, Cortijo et al. 2017, Dai et al. 2017).
Loss of H2A.Z is sufficient to enhance hormone-induced regeneration
To further investigate this hypothesis, we assessed whether loss of H2A.Z causes enhanced hormone-induced callus formation and shoot regeneration, similar to incubation at warm temperature. We thus performed in vitro regeneration procedures using explants from WT and two double mutants for the H2A.Z proteins, namely, hta9-1;hta11-1 and hta9-1;hta11-2. We did not use hta8;hta9;hta11 triple mutants, which completely lack H2A.Z, as they show very strong developmental defects (Coleman-Derr and Zilberman 2012).
We first investigated callus formation in these double mutants by incubating hypocotyl explants for 17 days at 22°C on CIM (Supplementary Fig. S5A, B) and observed no significant difference with WT explants. Therefore, we cannot conclude from this experiment that H2A.Z eviction contributes to the enhancement of callus formation by warm temperature.
In contrast, results were more consistent with our expectations when looking at shoot regeneration in these mutant explants after 4 days on CIM and then 11, 16 or 29 days on SIM at either 17°C, 22°C or 27°C (Fig. 4). We first noticed that only explants incubated at 27°C present shoots 11 days after transfer on SIM, when explants incubated at lower temperatures are still devoid of them (Fig. 4A, B). Similarly, 16 days after transfer on SIM, shoots are visible on explants incubated at 22°C or 27°C but remain absent from explants incubated at 17°C (Fig. 4C, D). As this applies to both WT and hta9;hta11 explants, this suggests that loss of H2A.Z at warmer temperatures poorly explains the dramatic acceleration of shoot regeneration observed in these conditions. We then examined the enhancement of shoot regeneration in hta9;hta11 explants compared to WT explants in function of the incubation temperature, using time points at which WT explants showed a similar regenerative progress for each condition (> 0 and <50 shoots per explant, i.e. 11 days after transfer on SIM at 27°C, 16 days after transfer on SIM at 22°C and 29 days after transfer on SIM at 17°C; Fig. 4B, D, F). At 17°C, hta9-1;hta11-1 explants produce 2.5 times and hta9-1;hta11-2 explants 2.1 times more shoots on average than WT explants (Fig. 4F), whereas at 22°C, hta9-1;hta11-1 explants produce 1.9 times and hta9-1;hta11-2 explants 2.1 times more shoots on average (Fig. 4D). Following the same trend, at 27°C, hta9-1;hta11-1 explants produce 1.6 times more shoots on average than WT explants, while no significant difference is detected between WT and hta9-1;hta11-2 explants (Fig. 4B). The positive effect of the hta9;hta11 double mutation on de novo shoot organogenesis thus appears to be reduced at 27°C compared to 17°C, indicating that loss of H2A.Z is sufficient to enhance shoot regeneration on SIM and that this effect largely contributes to the increased shoot production observed at warmer temperatures (Fig. 1C, D).
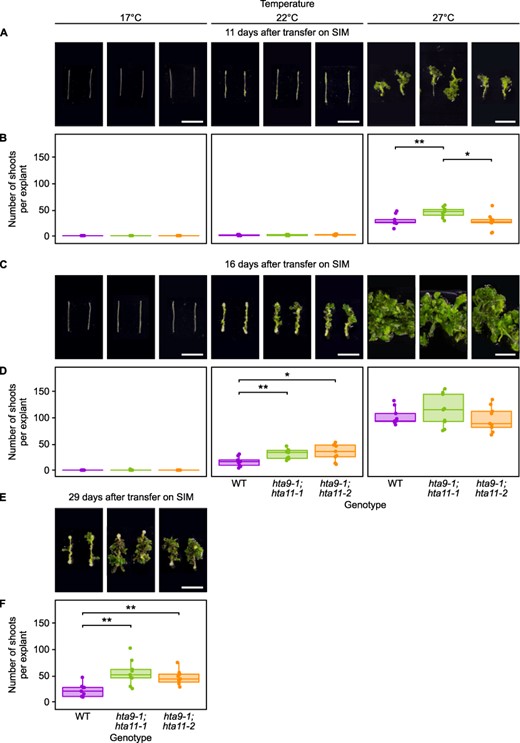
The enhancement of shoot regeneration in h2a.z mutants is stronger at cooler temperatures than at warmer temperatures. (A) Light microscopy images of WT, hta9-1;hta11-1 and hta9-1;hta11-2 hypocotyl explants incubated on CIM for 4 days and then on SIM for 11 days at 17°C, 22°C or 27°C. Scale bars = 10 mm. (B) Number of shoots produced per WT, hta9-1;hta11-1 and hta9-1;hta11-2 hypocotyl explant after incubation on CIM for 4 days and then on SIM for 11 days at 17°C, 22°C or 27°C (n ≥ 8). *P value < 0.05. **P value < 0.01 (Student’s t-test). (C) Light microscopy images of WT, hta9-1;hta11-1 and hta9-1;hta11-2 hypocotyl explants incubated on CIM for 4 days and then on SIM for 16 days at 17°C, 22°C or 27°C. Scale bars = 10 mm. (D) Number of shoots produced per WT, hta9-1;hta11-1 and hta9-1;hta11-2 hypocotyl explant after incubation on CIM for 4 days and then on SIM for 16 days at 17°C, 22°C or 27°C (n = 9). *P value < 0.05. **P value < 0.01 (Student’s t-test). (E) Light microscopy images of WT, hta9-1;hta11-1 and hta9-1;hta11-2 hypocotyl explants incubated on CIM for 4 days and then on SIM for 29 days at 17°C. Scale bars = 10 mm. (F) Number of shoots produced per WT, hta9-1;hta11-1 and hta9-1;hta11-2 hypocotyl explant after incubation on CIM for 4 days and then on SIM for 29 days at 17°C (n = 9). **P value < 0.01 (Student’s t-test).
Increased CUC1 expression contributes to enhanced shoot regeneration at warmer temperatures
We ultimately sought to check whether up-regulation of the regeneration genes putatively affected by H2A.Z eviction (Fig. 2C) is indeed responsible for the enhancement of callus formation and shoot regeneration at warm temperature. In particular, among genes for which up-regulation at 27°C correlates with H2A.Z loss (Figs. 2C, 3C), we focused our attention on CUC1 since its overexpression is known to enhance shoot regeneration at 22°C (Daimon et al. 2003). To test if a lack of H2A.Z is sufficient to induce CUC1 expression, we performed reverse transcription followed by quantitative polymerase chain reaction (RT-qPCR) on total RNA extracted from WT and hta9-1;hta11-1 hypocotyl explants which were incubated on CIM for 4 days then on SIM for 4 days at either 17°C or 27°C (Fig. 5A). In accordance with our hypothesis, we observed increased CUC1 expression in hta9-1;hta11-1 at all assessed time points when explants were incubated at 17°C (Fig. 5A). While this is also true at 27°C for explants incubated on CIM, the difference in the amount of CUC1 transcripts between hta9-1;hta11-1 and WT becomes non-significant when these explants are transferred to SIM (Fig. 5A). These results suggest that, in terms of CUC1 expression, hta9-1;hta11-1 is less sensitive than WT to an increase in temperature during hormone-induced shoot regeneration. Moreover, using expression array data published in Takeda et al. (2011), where the authors assayed the transcriptional landscape of 5-day-old cotyledons from a 35S::CUC1 overexpressor, we found a significant overlap between genes up-regulated in 35S::CUC1 and those up-regulated at 27°C compared to 17°C (Fig. 5B), implying that the CUC1-mediated transcriptional pathway is enhanced at 27°C.
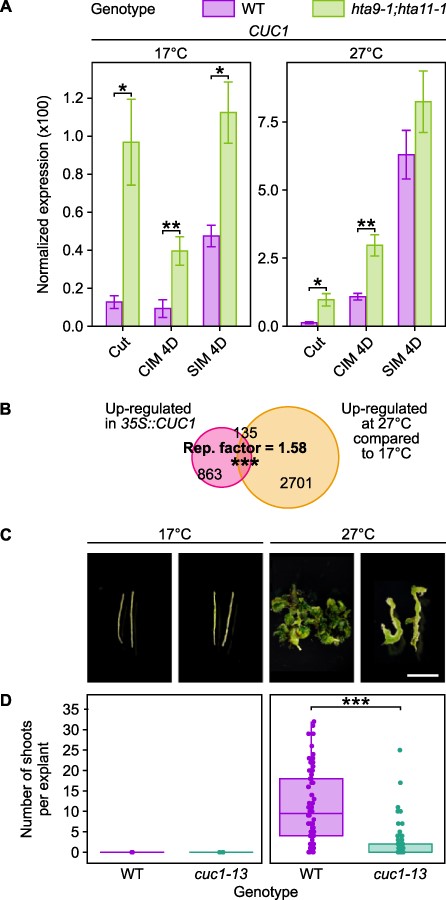
A mutation in CUC1 impairs the enhancement of regeneration by warm temperature. (A) RT-qPCR analysis showing the expression of CUC1 in WT and hta9-1;hta11-1 hypocotyl explants incubated on CIM for 4 days then on SIM for 4 days at 17°C or 27°C. Gene expression levels were normalized to PP2AA3 expression. Error bars show the standard deviation between three biological replicates. *P value < 0.05. **P value < 0.01 (Student’s t-test, n = 3). (B) Venn diagram representation of the overlaps between thermo-induced genes and CUC1-induced genes. Thermo-induced genes include those up-regulated at 27°C compared to 17°C on either CIM or SIM. CUC1-induced genes include those up-regulated in 5-day-old cotyledons from 35S::CUC1 overexpressors compared to the WT (Landsberg erecta; data from Takeda et al. (2011)). The enrichment (representation factor, hypergeometric test) between thermo-induced genes and CUC1-induced genes is indicated. ***P value < 0.001. (C) Light microscopy images of WT and cuc1-13 hypocotyl explants incubated on CIM for 4 days and then on SIM for 11 days, at either 17°C or 27°C. Scale bars = 5 mm. (D) Number of shoots produced per WT and cuc1-13 hypocotyl explant after incubation on CIM for 4 days and then on SIM for 11 days at 17°C or 27°C (n ≥ 15). ***P value < 0.001 (Student’s t-test).
We therefore investigated the callus formation and shoot regeneration abilities of cuc1-13 hypocotyls at 17°C and 27°C (Supplementary Fig. S5C, D, Fig. 5C, D). We found that cuc1-13 calli grown on CIM for 15 days weigh more than WT calli at both 17°C and 27°C (Supplementary Fig. S5C, D). However, different results were observed in explants incubated on CIM for 4 days and then on SIM for 11 days (Fig. 5C, D). At 17°C, where CUC1 is only slightly induced on SIM (Fig. 2C), no shoots were observed in WT nor cuc1-13 explants. At 27°C, where CUC1 is dramatically induced on SIM (Fig. 2C), explants from both genotypes exhibit shoot formation, but cuc1-13 explants produce substantially fewer shoots (6.1 times less; Fig. 5C, D). Altogether, these observations strongly suggest that up-regulation of CUC1 at 27°C contributes to the thermosensitive enhancement of shoot regeneration.
YUC activity is required for the enhancement of shoot regeneration at warmer temperatures
Our selection of warmth-induced regeneration genes that concomitantly lose H2A.Z (Figs. 2C, 3C) additionally features an auxin-responsive factor, SAUR15, as well as two genes coding for auxin biosynthesis enzymes, YUC1 and YUC4. Members of the YUC family play a role in thermomorphogenesis (Sun et al. 2012, Gyula et al. 2018), and higher auxin concentrations are known to enhance shoot regeneration to a certain extent (Skoog and Miller 1957, Ikeuchi et al. 2019). Moreover, YUC1 and YUC4 have previously been shown to be induced on SIM and double mutants for these genes exhibit drastically reduced shoot regeneration frequency (Cheng et al. 2012). Using the same reasoning and the same RT-qPCR assay described previously for CUC1 (Fig. 5A), we evaluated the expression of YUC1 and YUC4 in hta9-1;hta11-1 during shoot regeneration at 17°C and 27°C (Fig. 6A). On one hand, we found that the hta9-1;hta11-1 mutation does not affect YUC1 expression on CIM or SIM at 17°C and leads to diverging results at 27°C, with a 75.8% increase in YUC1 transcripts on CIM and a 37.8% decrease on SIM (Fig. 6A). It thus appears that loss of H2A.Z is not a promoting event for YUC1 expression. On the other hand, we observed that YUC4 expression is increased in hta9-1;hta11-1 compared to WT after 4 days on SIM at 17°C (21.4% more), but not at 27°C (Fig. 6A). Consequently, like for CUC1 (Fig. 5A), H2A.Z depletion at 27°C seems to promote YUC4 transcription in these conditions. Furthermore, we found a very strong overlap when comparing genes up-regulated at 27°C with genes up-regulated by a 3-day application of 4.5 µM exogenous auxin (NAA) in 15-day-old rosette leaves (Fig. 6B), which were obtained by re-analyzing a RNA-seq dataset published by Zia et al. (2019). Increased auxin biosynthesis due to the up-regulation of YUC1 and/or YUC4 may therefore also explain the enhancement of callus formation and shoot regeneration at 27°C and in h2a.z mutants.
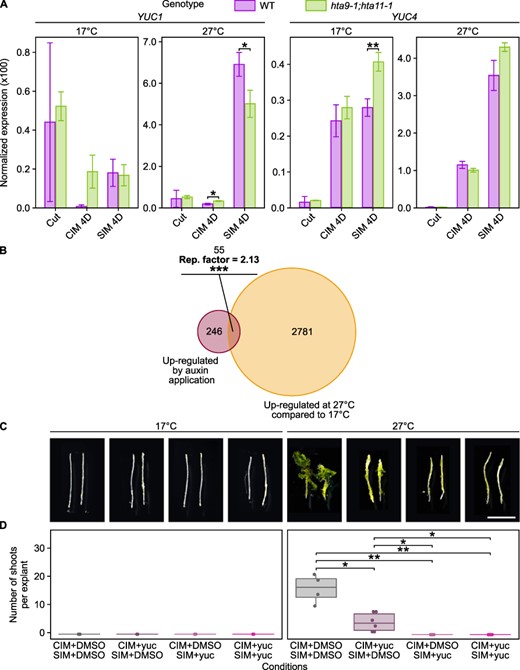
Increased YUC4 expression is partially responsible for enhanced hormone-induced regeneration at warmer temperatures. (A) RT-qPCR analysis showing the expression of YUC1 and YUC4 in WT and hta9-1;hta11-1 hypocotyl explants incubated on CIM for 4 days then on SIM for 4 days at 17°C or 27°C. Gene expression levels were normalized to PP2AA3 expression. Error bars show the standard deviation between three biological replicates. *P value < 0.05. **P value < 0.01 (Student’s t-test, n = 3). (B) Venn diagram representation of the overlaps between thermo-induced genes and auxin-induced genes. Thermo-induced genes include those up-regulated at 27°C compared to 17°C on either CIM or SIM. Auxin-induced genes include those up-regulated in 15-day-old rosettes from seedlings incubated for 3 days on regular medium supplemented with 4.5 µM auxin (NAA; data from Zia et al. (2019)). The enrichment (representation factor, hypergeometric test) between thermo-induced genes and auxin-induced genes is indicated. ***P value < 0.001. (C) Light microscopy images of WT hypocotyl explants incubated at 17°C or 27°C for 4 days on CIM and then for 11 days on SIM. CIM and SIM were supplemented with DMSO or 10 µM yucasin (yuc). Scale bars = 5 mm. (D) Number of shoots produced per WT hypocotyl explant after incubation at either 17°C or 27°C for 4 days on CIM and then for 11 days on SIM. CIM and SIM were supplemented with DMSO or 10 µM yucasin (yuc; n ≥ 6). *P value < 0.05. **P value < 0.01 (Student’s t-test).
To test this hypothesis, we first incubated WT hypocotyl explants on CIM supplemented with or without 10 µM 5-(4-chlorophenyl)-4 H-1,2,4-triazole-3-thiol (yucasin), a YUC inhibitor (Nishimura et al. 2014), for 17 days at 17°C or at 27°C (Supplementary Fig. S5E, F). At 17°C, explants show mildly increased callus formation upon exposure to yucasin, although the significance of this increase weakens at 27°C, strongly suggesting that elevated YUC activity does not contribute to increased callus formation on CIM at higher temperatures. We next incubated WT explants for 4 days on CIM supplemented with or without 10 µM yucasin and then for 13 days on SIM supplemented with or without 10 µM yucasin, at either 17°C or 27°C (Fig. 6C, D). As expected at 17°C, where YUC4 is only mildly induced on CIM and SIM (Fig. 2C), we observed no difference between explants submitted to different treatments (Fig. 6C, D). At 27°C, where YUC4 is highly induced on CIM/SIM (Fig. 2C), the number of shoots produced per explants decreases as the time of exposure to yucasin increases; in particular, explants grown at 27°C and exposed to yucasin during SIM incubation largely resemble those grown at 17°C (Fig. 6C, D). These observations strongly suggest that increased YUC activity is partially responsible for enhancing shoot regeneration at 27°C. Interestingly, while YUC activity during CIM incubation does not seem necessary for callus formation per se, it does appear to be required on CIM for proper shoot regeneration later on, as inhibition of auxin biosynthesis on this medium alone leads to significantly reduced shoot regeneration in explants incubated at 27°C (3.7 times less; Fig. 6D). However, we cannot exclude that this effect results from yucasin being carried over in the explants during the transfer from CIM to SIM. Nevertheless, these results together support the idea that temperature enhances shoot regeneration via the up-regulation of genes coding for transcription factors such as CUC1 as well as auxin biosynthesis enzymes such as YUC4.
Discussion
Warm ambient temperature promotes hormone-induced callus formation and shoot regeneration
In this study, we uncovered a new parameter that influences the efficiency of hormone-induced regeneration in Arabidopsis thaliana. Our CIM/SIM assays demonstrate that an increase in ambient temperature improves both callus formation on CIM and shoot regeneration on SIM (Fig. 1). Likely because the temperature range used in this study is not very stressful for Arabidopsis thaliana (Kumar and Wigge 2010), warm temperature appears to primarily amplify changes in the transcription of genes that are already affected by the hormones present in CIM and SIM (Supplementary Fig. S3A).
Our observations suggested that warm temperature enhances shoot regeneration by affecting multiple pathways ranging from transcriptional regulation of shoot meristem formation to auxin biosynthesis (Figs. 5, 6). These results could reflect the global enhancement of plant growth at warm temperatures. In particular, we predict that the transcription factor CUC1 plays an important role in the response to temperature since its expression is strongly enhanced at 27°C (Figs. 2C, 5A) and a previous study showed that CUC1 overexpression in 35S::CUC1 explants promotes shoot regeneration at 22°C (Daimon et al. 2003). While no clear connections have previously been made between CUC1 and thermosensitive development, its homologs CUC2 and CUC3, which are also up-regulated at 27°C on SIM (Supplementary Fig. S3F), are known to define leaf morphogenesis (Nikovics et al. 2006, Hasson et al. 2011), a process which is affected by ambient temperature in various species (Royer 2012, Nakayama et al. 2014, McKee et al. 2019). It is thus possible that members of the CUC family act as downstream effectors of thermo-sensory responses in contexts other than regeneration.
Our data further showed that YUC-mediated auxin biosynthesis is a key downstream pathway important for the temperature response. We found that two members of the YUC family, YUC1 and YUC4, are up-regulated during CIM and/or SIM incubation at 27°C (Fig. 2C) and that application of a YUC inhibitor decreases the enhancement of shoot regeneration by higher temperatures (Fig. 6C, D). Previous studies have shown that warm temperatures increase auxin levels by stimulating the expression of YUC4 and YUC8 (Gray et al. 1998, Sun et al. 2012). Moreover, mutants deficient in auxin synthesis display defective hypocotyl elongation and leaf hyponasty in response to warm temperature (Gray et al. 1998, Sun et al. 2012). These observations, together with our results, strongly suggest that responses to warm temperature heavily rely on elevated auxin levels promoted by an increase in its biosynthesis during regular development and de novo shoot organogenesis. As demonstrated for CUC1 (Daimon et al. 2003), it will be interesting to test whether YUC overexpression alone is sufficient to mimic increased shoot regeneration at warm temperatures. Intriguingly, increased YUC expression does not appear to account for improved callus formation on CIM, as this process is not affected by chemical inhibition of YUCs (Supplementary Fig. S5E, F). Given that none of the pathways investigated in this study explain how an increase in ambient temperature enhances hormone-induced callus formation, further research is needed to understand the molecular basis of this phenomenon.
It should also be noted that multiple cellular pathways that underlie hormone-induced regeneration, such as cell proliferation and fate change, appear to be enhanced at 27°C compared to lower temperatures (Fig. 1). This leads us to predict that the developmental effects of warm temperature described in this study may be restricted to or strongly exaggerated in the context of in vitro culture on regenerative media. In line with this idea, we additionally found that warm temperature poorly influences wound-induced callus formation at the cut site in hypocotyls (Supplementary Fig. S2). Accordingly, our GO analysis revealed that many wound response genes are down-regulated by warm temperature (Supplementary Fig. S3D). However, this result could simply be due to the timepoints at which our RNA-seq samples were harvested (4 days and 8 days after cutting) as wound responses are activated quite rapidly (within 24 h after cutting; Ikeuchi et al. 2017, Rymen et al. 2019). Despite this caveat, these observations bring additional evidence that the processes of hormone- and wound-induced callus formation are regulated differently. This is consistent with previous results showing divergent roles for epigenetic modifiers, such as HISTONE ACETYLTRANSFERASE OF THE GNAT FAMILY 1 (HAG1), in the regulation of CIM-induced callus formation versus wound-induced callus formation (Kim et al. 2018, Rymen et al. 2019).
An increase in temperature relieves the H2A.Z-mediated repression of shoot regeneration
Our study brings new evidence suggesting that the influence of warm temperature on de novo shoot organogenesis is regulated by H2A.Z. Kumar and Wigge (2010) first enunciated the model in which an increase in temperature induces H2A.Z eviction and thereby modifies the expression of numerous thermosensitive genes. Our results expand this model to the context of hormone-induced regeneration, and confirm that H2A.Z is a dynamic mark conferring transcriptional sensitivity to the environment in plants, as proposed by Coleman-Derr and Zilberman (2012). It should be noted that some studies have proposed a dual regulatory role for H2A.Z when located close to the TSS, where it is suggested to act both as transcriptional activator and a transcriptional repressor depending on the context (Zilberman et al. 2008, Coleman-Derr and Zilberman 2012, Sura et al. 2017, Gómez-Zambrano et al. 2019). However, in our study, elevated H2A.Z occupancy close to the TSS was mainly associated with repressive effects (Fig. 3). Importantly, by choosing to re-analyze a previously published ChIP-seq dataset that examines early responses to warm temperatures (Cortijo et al. 2017), we did not assess H2A.Z occupancy at the same time points at which we evaluated gene expression or regeneration phenotypes. Further work is therefore needed to determine whether the H2A.Z landscape remain unchanged throughout CIM/SIM incubation at warm or cool temperature. Nevertheless, our RT-qPCR assays (Figs. 5A, 6A) clearly demonstrate that, like in an hta9;hta11 mutant where H2A.Z levels are constitutively reduced, exposure to 27°C promotes CUC1 or YUC4 transcription in WT explants after 4 days on SIM, suggesting that H2A.Z occupancy remains low during prolonged incubation at warm temperatures. In addition, as we detected the strongest differential expression at this time point, i.e. when CUC1 or YUC4 are the most transcribed (Fig. 2C), we hypothesize that a reduction in H2A.Z levels alone may not be sufficient to induce the expression of target regeneration genes, but rather creates a chromatin environment that facilitates their up-regulation by hormone-responsive factors upon exposure to SIM. Moreover, our phenotyping results using yucasin (Fig. 6C, D) are consistent with those obtained by Lee and Seo (2017), who showed that application of this inhibitor to mutants for ARP6, encoding a subunit of the SWR1 complex that catalyzes the deposition of H2A.Z (March-Díaz and Reyes 2009), rescues their elongated hypocotyl phenotype caused by YUC9 overexpression.
Purified Arabidopsis H2A.Z-containing nucleosomes show no direct reduction of DNA-histone octamer association upon changes in ambient temperature in vitro (Cortijo et al. 2017), revealing the existence of a sensory mechanism responsible for detecting temperature fluctuations and translating it to H2A.Z occupancy in vivo. Recent studies have unveiled the role of the INO80 chromatin remodeling complex (Zander et al. 2019, Willige et al. 2021, Xue et al. 2021) and/or of specific transcription factors for H2A.Z eviction upon environmental cues, such as PIF7 in the response to changes in light quality (Willige et al. 2021), PIF4 during thermomorphogenesis (Xue et al. 2021) or heat shock factors for HSP70 induction at warm temperature (Cortijo et al. 2017). Whether the temperature-mediated relief of H2A.Z repression on shoot regeneration genes depends on the function of such factors should therefore be addressed in future studies.
Although we observed significantly enhanced shoot regeneration in mutants for the HTA genes at 22°C, these mutants do not completely phenocopy explants cultured at 27°C (Figs. 1, 4). This could be due to none of the tested mutants being null for H2A.Z, but may also suggest that our H2A.Z-based model is not the only mechanism by which temperature affects hormone-induced regeneration. H2A.Z eviction may mainly contribute to the temperature response by increasing chromatin accessibility, but likely does not explain all other effects induced by warmth, such as the recruitment of thermosensitive transcription factors to their target promoters. In addition, other epigenetic marks may also contribute to the enhancement of regeneration by temperature. For instance, repressive histone H3 lysine 27 trimethylation (H3K27me3) has been associated with the regulation of thermosensitive processes such as flowering (Noh et al. 2004, Zheng et al. 2019), and seedlings unable to catalyze H3K27me3 spontaneously form callus structures (Bouyer et al. 2011). Moreover, H3K27me3 co-localizes with H2A.Z (Carter et al. 2018), for example on the YUC4 loci (Lu et al. 2019), and is hypothesized to regulate common transcriptional networks (Carter et al. 2018). Another histone modification mark, histone H3 lysine 9 acetylation (H3K9ac), has been more clearly linked to the regulation of thermomorphogenesis. Specifically, warm temperature promotes POWERDRESS (PWR) to recruit HISTONE DEACETYLASE 9 (HDA9) to the YUC8 locus and thereby maintains low levels of H3K9ac at its +1 nucleosome (Tasset et al. 2018). This was reported to be critical for proper H2A.Z eviction by warm temperature (van der Woude et al. 2019) and thus makes it a candidate mechanism worth investigating.
Given that our results were all obtained using Arabidopsis thaliana ecotype Columbia, whether exposure to warm temperature and/or loss of H2A.Z similarly enhances hormone-induced regeneration in other ecotypes or plant species, notably those that are more recalcitrant to regeneration (Benson 2000, Motte et al. 2014, Lardon et al. 2020), would be an interesting question to address in future research. Previous studies showed that various Arabidopsis ecotypes display diverse degrees of regeneration efficiency (Motte et al. 2014, Lardon et al. 2020), which leads us to predict that some respond to temperature cues in a similar manner. Moreover, as temperature impacts the development and yield of numerous crop species such as tomato (Lycopersicon esculentum; Adams et al. 2001), wheat (Triticum spp.; Wang et al. 2017), corn (Zea mays), soybean (Glycine max) and cotton (Gossypium spp.; Schlenker and Roberts 2009), warm temperatures may likewise promote shoot regeneration in these species. In particular, H2A.Z is one of the most conserved histone variants among eukaryotes (Thatcher and Gorovsky 1994) and also regulates responses to temperature in Brachypodium distachyon (Boden et al. 2013). H2A.Z may thus have an analogous repressor role in the regeneration of other plants and this possibility as well as its potential downstream applications should be thoroughly investigated.
Materials and Methods
Plant materials and growth conditions
All Arabidopsis thaliana plants used in this study are ecotype Columbia (Col-0), from the following accessions: SALK_054814 crossed with SALK_017235 (hta9-1;hta11-1), SALK_054814 crossed with SALK_031471 (hta9-1;hta11-2) and SALK_006496 (cuc1-13). These alleles were previously described in March-Díaz et al. (2007; hta9-1;hta11-1), Xu et al. (2017; hta9-1;hta11-2) and Hibara et al. (2006; cuc1-13). Plants were grown on nylon mesh placed on Murashige–Skoog (MS) medium containing 1% sucrose and 0.6% (w/v) gelzan. Plates were placed vertically at 22°C in SANYO MLR-352 (Panasonic®) growth chambers with an unmonitored humidity varying from 24% to 57%, and kept in the dark for 7 days to induce hypocotyl elongation.
Callus formation and shoot regeneration
For hormone-induced callus formation, 7-day-old etiolated seedlings were dissected with a razor blade to generate hypocotyl explants, which were taken from the region spanning ∼3 mm above the root/hypocotyl junction and ∼5 mm below the hypocotyl/shoot junction, or root explants, which were taken from the region spanning ∼4 to ∼14 mm below the root/hypocotyl junction. Explants were incubated in the growth chambers described above under constant fluorescent white light (∼50 µmol.m−2.s−1) at the specified temperatures and for the indicated number of days on CIM, i.e. MS medium supplemented with Gamborg’s B5 vitamin, 20 g.L−1 glucose, 0.5 g.L−1 2-(N-morpholino)ethane-sulfonic acid (MES), 7.5 g.L−1 Gellan gum, 0.1 mg.L−1 kinetin and 0.5 mg.L−1 2,4-dichlorophenoxyacetic acid (2,4 D; Valvekens et al. 1988). To induce shoot regeneration, explants incubated for 4 days on CIM were transferred to SIM, i.e. MS medium supplemented with Gamborg’s B5 vitamin, 20 g.L−1 glucose, 0.5 g.L−1 MES, 2.5 g.L−1 Gellan gum, 0.5 mg.L−1 N6-(2-isopentenyl)adenine (2-iP) and 0.15 mg.L−1 indole-3-acetic acid (IAA; Valvekens et al. 1988). The explants were then incubated in the same conditions as for CIM, at the specified temperatures and for the indicated number of days. To assess the importance of auxin biosynthesis in the context of hormone-induced callus formation and shoot regeneration, CIM and/or SIM were supplemented with 10 µM yucasin (CAS No. 26028-65-9, Wako®, FUJIFILM®; Nishimura et al. 2014), and dimethyl sulfoxide (DMSO) was used as a control. To measure fresh weight, explants were dabbed on a Kimwipe® paper tissue to remove media and weighed five at a time using an XS104 balance (Mettler Toledo®). Weight values were then normalized based on the summed initial lengths of the corresponding explants. Shoot regeneration efficiency was quantified as the number of shoots displayed by each explant.
For wound-induced callus formation, 7-day-old seedlings were cut with a razor blade ∼7 mm above the root/hypocotyl junction. For each seedling, the apical part was removed and the remaining portion was left on the same plate of MS medium and incubated vertically, in the dark and at the specified temperature for the indicated number of days. Callus formation was quantified by counting, per plate, the number of explants showing at least two newly formed cells at the wound-site when observed using a dissecting microscope. To calculate callus area projections, calli were photographed using DIC microscopy with an Olympus® BX51 microscope and their edges were manually traced on the resulting pictures using a graphic tablet (XP-PEN® Artist 22E Pro). The filling areas were measured with ImageJ (version 1.52p).
All assays were replicated on at least three different plates per genotype and/or condition.
RNA extraction, sequencing and data analysis
Total RNA was isolated from hypocotyl explants incubated in the specified conditions in triplicates. For each replicate, ∼100 hypocotyls were harvested and frozen with liquid nitrogen. The samples were then ground using zirconia ceramic beads in a TissueLyserTM (QIAGEN®) mixer mill. RNA was extracted using the RNeasy® Plant Mini kit (QIAGEN®) following the manufacturer’s instructions. Libraries for sequencing were prepared using the KAPA Stranded mRNA-seq KitTM (Kapa Biosystems®, Roche®) with a mix of FastGeneTM (Nippon Genetics®) and NEBNext® (New England Biolabs®) adapters, and sequencing was performed on an Illumina® NextSeqTM 500 platform in single-end.
Raw sequencing files were processed on a virtual machine running on Ubuntu (version 18.04.1). Sequencing quality was first checked using FastQC (version 0.11.7; Andrews 2010). Reads were then mapped to the TAIR10 Arabidopsis thaliana genome using Bowtie2 (version 2.3.4.3; Langmead and Salzberg 2012) with the ‘--no-1mm-upfront’ option and further processed using the samtools (version 1.9; Li et al. 2009) functions view, sort, rmdup and index. The read mapping rate was > 55% for all RNA-seq samples. Read counts per gene were determined using the multicov function from bedtools (version 2.26.0; Quinlan and Hall 2010). Differential expression analyses were performed on R (version 3.4.4; R Core Team 2021) with the ‘edgeR’ package (version 3.22.5; Robinson et al. 2009, McCarthy et al. 2012). Genes with an absolute fold change > 1.5 and a false discovery rate (edgeR) < 0.001 were considered differentially expressed. Venn diagrams were drawn using the R package ‘Vennerable’ (version 3.0; Swinton 2013) and line plots and heatmaps were plotted using the R packages ‘gplots’ (version 3.0.1; Warnes et al. 2020) and ‘ggplot2’ (version 3.1.0; Wickham 2016). Figures were assembled in R with packages ‘grid’ (version 3.5.1; R Core Team 2021), ‘gridExtra’ (version 2.3; Auguie 2017), ‘cowplot’ (version 0.9.3; Wilke 2020) and ‘ggplotify’ (version 0.0.3; Yu 2020) and further refined in Inkscape (version 0.92.3).
The same in silico analyses were performed on the raw files from the RNA-seq dataset in 15-day-old rosettes exposed to an external application of auxin, which was previously published in Zia et al. (2019).
Gene Ontology (GO) enrichment analysis
GO analyses were performed on R (version 3.4.4; R Core Team 2021) using the ‘biomaRt’ (version 2.38.0; Durinck et al. 2005, 2009), ‘GO.db’ (version 3.7.0; Carlson 2020) and ‘topGO’ (version 2.34.0; Alexa and Rahnenfuhrer 2020) packages. For each gene category, the 10 most enriched terms were selected (Fisher’s exact test). Figures were drawn using the R packages ‘gplots’ (version 3.0.1; Warnes et al. 2020) and ‘ggplot2’ (version 3.1.0; Wickham 2016).
ChIP-seq data analysis
The dataset from the HTA11 ChIP-seq performed at 17°C and 27°C in WT whole seedlings was previously published in Cortijo et al. (2017). Raw sequencing files were processed on a virtual machine running on Ubuntu (version 18.04.1). Sequencing quality was first checked using FastQC (version 0.11.7; Andrews 2010), then reads were mapped to the TAIR10 Arabidopsis thaliana genome using Bowtie2 (version 2.3.4.3; Langmead and Salzberg 2012) with the ‘--no-1mm-upfront’ option, and further processed using the samtools (version 1.9; Li et al. 2009) functions view, sort, rmdup and index. The read mapping rate was > 62% for all samples. Peak-calling was performed using SICER2 (version 1.1; Xu et al. 2014) without a control and with the following parameters: window size 200 bp; fragment size 150 bp; effective genome fraction 0.74; FDR cutoff 0.01; gap size minimum 600 bp; e-value 1,000. Peaks obtained from at least 2 replicates were extracted and merged using the bedtools (version 2.26.0; Quinlan and Hall 2010) functions intersect and merge. These peaks were then mapped to TAIR10 genes using bedtools intersect. In total, 13,628 peaks intersecting with 21,378 genes were detected at 17°C and 15,542 peaks intersecting with 22,756 genes were detected at 27°C. The HTA11 enrichment of each gene was calculated as the mean coverage of all its intersecting peaks. Differentially marked genes were defined as genes with a ratio ‘enrichment at 27°C / enrichment at 17°C’ > 1.2 or < 0.8. Coverage BigWig files were produced using the deepTools (version 3.2.1; Ramírez et al. 2014) function bamCoverage with option ‘-bs 2’ from .bam files previously merged with samtools merge. Venn diagrams were drawn on R (version 3.4.4; R Core Team 2021) with the package ‘Vennerable’ (version 3.0; Swinton 2013); line plots and heatmaps were plotted using the R packages ‘gplots’ (version 3.0.1; Warnes et al. 2020), ‘ggplot2’ (version 3.1.0; Wickham 2016), ‘EnrichedHeatmap’ (version 1.12.0; Gu et al. 2018) and ‘wiggleplotr’ (version 1.6.1; Alasoo 2020); figures were assembled and further refined in Inkscape (version 0.92.3).
Expression array data analysis
The expression array dataset assessing gene expression in cotyledons isolated from 5-day-old WT Landsberg erecta and 35S::CUC1 overexpressing plants re-analyzed in this study was previously published in Takeda et al. (2011). Genes expressed at a low intensity (sum of means of raw intensity < 1) were removed and genes with an absolute fold change > 1.5 in the transformant compared to the control and a q value < 0.05 (Benjamini and Hochberg False Discovery Rate) were considered as differentially expressed. Venn diagrams were drawn on R with the package ‘Vennerable’ (version 3.0; Swinton 2013).
RT-qPCR assay
Total RNA was isolated from hypocotyl explants incubated in the specified conditions in triplicates. For each replicate, ∼100 hypocotyls were harvested and frozen with liquid nitrogen. The samples were then ground using zirconia ceramic beads in a TissueLyserTM (QIAGEN®) mixer mill. RNA was extracted using the RNeasy® Plant Mini kit (QIAGEN®) following the manufacturer’s instructions. The resulting RNA concentrations were determined with a NanoDropTM 2000 spectrophotometer (Thermo Fisher Scientific®) and normalized between samples. Genomic DNA was removed by incubating the samples for 2 min at 42°C in the presence of the gDNA Eraser enzyme (TaKaRa®). Reverse-transcription was then performed using the PrimeScriptTM RT reagent Kit (TaKaRa®) following the manufacturer’s instructions. Transcript levels were determined with a Mx300P Real-Time PCR System (Agilent®) using the THUNDERBIRDTM SYBR® qPCR Mix (Toyobo®) and the following primers:
CUC1: 5ʹ-TGCATGAGTATCGCCTTGAC-3ʹ and 5ʹ-AACGCCACGCCATCACCGAC-3ʹ (previously published in Hasson et al. 2011),
YUC1: 5ʹ-TTCCTAAGGGCTGGAGAGGA-3ʹ and 5ʹ-TATTCCTGGTGGACCCCTTC-3ʹ,
YUC4: 5ʹ-TAACGAGGAACGGGGCAAAG-3ʹ and 5ʹ-GGCGTTTTTGGCATTCCTTCT-3ʹ,
PP2AA3: 5ʹ-TAACGTGGCCAAAATG-3ʹ and 5ʹ-GTTCTCCACAACCGCTTGGT-3ʹ.
Expression values were retrieved from Ct values with an adjustment to each primer pair efficiency, which were determined by dilution series made simultaneously with control WT cDNA. CUC1, YUC1 and YUC4 expression values were then normalized to PP2AA3 expression within each sample.
Supplementary Data
Supplementary Data are available at PCP online.
Data Availability
New RNA-seq data generated in this study have been deposited to the Gene Expression Omnibus (GEO) data repository with accession number GSE176161. Raw files from previously published assays which were re-analyzed in this study can be obtained from GEO with accession numbers GSE79355 (for Cortijo et al. (2017)’s dataset), GSE27482 (for Takeda et al. (2011)’s dataset), and GSE134079 (for Zia et al. (2019)’s dataset). Sequence data for genes investigated in this study can be found in the Arabidopsis Genome Initiative or GenBank/EMBL-EBI databases under the following accession numbers: AT3G15170 (CUC1), AT1G76420 (CUC3), AT1G24590 (ESR2), AT3G12580 (HSP70), AT2G38810 (HTA8), AT1G52740 (HTA9), AT3G54560 (HTA11), AT1G68460 (IPT1), AT2G37210 (LOG3), AT1G13320 (PP2AA3), AT4G38850 (SAUR15), AT2G17950 (WUS), AT4G32540 (YUC1) and AT5G11320 (YUC4).
Funding
This work was supported by the Special Scholarship for International Students from the University of Tokyo to A.L. and KAKENHI Grants-in-Aid from the Japanese Ministry of Education, Culture, Sports, Science and Technology (MEXT) [19F19781 to D.S.F. and 17H03704, 20H05911 and 20H05905 to K.S.].
Acknowledgements
The authors are grateful to the members of Cell Function Research Team for discussion and comments on the manuscript.
Author Contributions
A.L., T.T. and K.S. designed research with inputs from B.R., A.I., M.I., S.C., K.E.J. and P.A.W.; A.L., A.K. and T.T. performed regeneration phenotyping assays; A.K. performed RNA-seq experiments, T.S. sequenced RNA-seq libraries and A.L. and T.T. analyzed the data, with inputs from A.I. and M.I.; A.L., D.S.F. and K.S. wrote the manuscript with inputs from all authors.
Disclosures
No conflicts of interest declared.