-
PDF
- Split View
-
Views
-
Cite
Cite
Pranita D Tamma, Sima L Sharara, Zoi D Pana, Joe Amoah, Stephanie L Fisher, Tsigereda Tekle, Yohei Doi, Patricia J Simner, Molecular Epidemiology of Ceftriaxone-Nonsusceptible Enterobacterales Isolates in an Academic Medical Center in the United States, Open Forum Infectious Diseases, Volume 6, Issue 8, August 2019, ofz353, https://doi.org/10.1093/ofid/ofz353
- Share Icon Share
Abstract
Knowledge of whether Enterobacterales are not susceptible to ceftriaxone without understanding the underlying resistance mechanisms may not be sufficient to direct appropriate treatment decisions. As an example, extended-spectrum β-lactamase (ESBL)–producing organisms almost uniformly display nonsusceptibility to ceftriaxone. Regardless of susceptibility to piperacillin-tazobactam or cefepime, carbapenem antibiotics are the treatment of choice for invasive infections. No such guidance exists for ceftriaxone-nonsusceptible organisms with mechanisms other than ESBL production. We sought to investigate the molecular epidemiology of ceftriaxone-nonsusceptible Enterobacterales.
All consecutive Escherichia coli, Klebsiella pneumoniae, Klebsiella oxytoca, or Proteus mirabilis clinical isolates with ceftriaxone minimum inhibitory concentrations (MICs) of ≥2 mcg/mL from unique patients at a United States hospital over an 8-month period were evaluated for β-lactamase genes using a DNA microarray–based assay.
Of 1929 isolates, 482 (25%) had ceftriaxone MICs of ≥2 mcg/mL and were not resistant to any carbapenem antibiotics. Of the 482 isolates, ESBL (blaCTX-M, blaSHV, blaTEM) and/or plasmid-mediated ampC (p-ampC) genes were identified in 376 (78%). ESBL genes were identified in 310 (82.4%), p-ampC genes in 2 (0.5%), and both ESBL and p-ampC genes in 64 (17.0%) of the 376 organisms. There were 211 (56%), 120 (32%), 41 (11%), and 4 (1%) isolates with 1, 2, 3, or ≥4 ESBL or p-ampC genes. The most common ESBL genes were of the blaCTX-M-1 group (includes blaCTX-M-15), and the most common p-ampC gene was blaCMY-2.
There is considerable diversity in the molecular epidemiology of ceftriaxone-nonsusceptible Enterobacterales. An understanding of this diversity can improve antibiotic decision-making.
Ceftriaxone is one of the most commonly prescribed antibiotics to treat infections caused by the Enterobacterales (formerly the Enterobacteriaceae), both as empiric and culture-directed antibiotic therapy [1]. Unfortunately, rising rates of resistance to ceftriaxone and other expanded-spectrum cephalosporins among the Enterobacterales have led to the need to resort to increasingly broad-spectrum β-lactam agents like carbapenems on a more frequent basis [1]. Escherichia coli, Klebsiella species, and Proteus mirabilis contribute to large portions of gram-negative infections. These organisms have achieved global prominence for their production of extended-spectrum β-lactamase (ESBL) enzymes—particularly CTX-M-15 [2, 3]. Reasons for the international success of blaCTX-M genes are multifold and include horizontal gene transfer of mobile genetic elements (eg, ISEcp1, F plasmids); successful E. coli clones (eg, sequence type 131); presence of ESBL genes in animal products, possibly as a consequence of antibiotic overuse and overcrowding (including close proximity of animals and humans); environmental contamination from human and animal waste due to poor sanitation; and human travel and migration patterns [3] (eg, 22% of travelers to South Asia become colonized with ESBL-producing bacteria) [4].
Unlike blaCTX-M genes, the contribution of blaTEM and blaSHV ESBL genes has been understudied—possibly because US Food and Drug Administration–cleared platforms for β-lactamase gene detection (eg, Verigene Gram-Negative Blood Culture Test, GenMark ePlex Blood Culture Identification Gram-negative Panel, etc.) limit ESBL detection to blaCTX-M genes. All CTX-M enzymes are ESBLs, whereas not all TEM and SHV enzymes are ESBLs; TEM and SHV ESBLs (eg, blaTEM-12, blaSHV-12) arise from amino acid substitutions in non-ESBL TEM or SHV β-lactamases—most notably, Gly238 or Arg164 [5].
The impact of plasmid-associated AmpC (p-ampC) genes, a cause of constitutive and often high-level resistance to expanded-spectrum cephalosporins, has been largely ignored by clinicians. Initial recognition of p-ampCs occurred in 1989 with identification of blaCMY-1 [6]. Common p-ampC genes that have since been recognized include blaDHA, blaFOX, and blaACT. Unlike ESBL genes, p-ampC genes have had wide inconsistencies in their nomenclature criteria, including being named by the place of discovery, by hydrolyzed substrates, or even by patients’ names, challenging their name recognition [7]. Additionally, their exclusion from Food and Drug Administration–cleared multiplex platforms and the lack of a standardized approach for AmpC β-lactamase detection has led to an underappreciation of this resistance mechanism.
Currently, there is no Clinical and Laboratory Standards Institute–endorsed phenotypic method for confirming either ESBL or AmpC production, resulting in a minority of clinical microbiology laboratories performing confirmatory ESBL testing and virtually no clinical laboratories performing AmpC confirmatory testing [8]. Knowledge of whether E. coli, Klebsiella spp., and P. mirabilis are not susceptible to ceftriaxone without an understanding of the underlying resistance mechanisms may not be sufficient to direct appropriate treatment decisions. As an example, ESBL-producing organisms almost uniformly display nonsusceptibility to ceftriaxone (ie, ceftriaxone minimum inhibitory concentrations [MICs] ≥2 mcg/mL) [9], and even if these organisms are susceptible to piperacillin-tazobactam or cefepime, carbapenem antibiotics are the treatment of choice for invasive ESBL infections [10]. On the other hand, no such guidance exists for ceftriaxone-nonsusceptible organisms with mechanisms other than ESBL production. Our objective was to describe the molecular epidemiology of ceftriaxone-nonsusceptible clinical isolates to inform antibiotic decision-making practices.
METHODS
From December 2014 to July 2015, all consecutive ceftriaxone-nonsusceptible E. coli, K. pneumoniae, K. oxytoca, or P. mirabilis clinical isolates from unique patients who received medical care at The Johns Hopkins Hospital in Baltimore, Maryland, were collected and stored at –80°C in glycerol until further testing was performed. No surveillance isolates were included. Clinical samples were processed at The Johns Hopkins Hospital Medical Microbiology Laboratory according to standard operating procedures. Antimicrobial susceptibility testing was previously performed using the BD Phoenix Automated System (BD Diagnostics, Sparks, MD).
Clinical isolates with ceftriaxone MICs of ≥2 mcg/mL were further evaluated for the presence of β-lactamase genes. Isolates not susceptible to any carbapenem antibiotic (ertapenem MIC ≥1 mcg/mL, meropenem or imipenem MIC ≥2) were excluded from further analysis. Genomic DNA was extracted from isolates using the DNeasy Blood & Tissue Kit (QIAGEN, Inc., Valencia, CA). Identification of β-lactamase-encoding genes was assessed utilizing a DNA microarray–based assay, the Check-MDR CT101 kit (Check-Points, Wageningen, the Netherlands), according to the manufacturer’s protocol. The Check-Points assay detects important blaCTX-M ESBL and p-ampC genes and single nucleotide polymorphisms that correspond to relevant amino acid changes that result in clinically significant TEM and SHV-type ESBLs. More specifically, it detects the following: (1) blaCTX-M ESBLs: blaCTX-M-1 group, blaCTX-M-2 group, blaCTX-M-8 & -25 group, blaCTX-M-9 group; (2) blaTEM ESBLs: TEM E104K, TEM R164S, TEM R164H, TEM G238S; (3) blaSHV ESBLs: SHV G238S, SHV G238A, and SHV E240K; and (4) p-ampCs: blaCMY-1/MOX, blaCMY-2, blaACC, blaDHA, blaACT/MIR, and blaFOX. Data analysis was largely descriptive in nature. The study was approved by The Johns Hopkins University School of Medicine Institutional Review Board. A waiver of informed consent was granted.
RESULTS
During the 8-month study period, there were 1929 E. coli (n = 1041), K. pneumoniae (n = 540), K. oxytoca (n = 115), and P. mirabilis (n = 233) isolates from unique patients. Of these, 482 (25%) had ceftriaxone MICs of ≥2 mcg/mL and were not resistant to any carbapenem antibiotics. These 482 isolates underwent further molecular testing to evaluate for the presence of ESBL and/or p-ampC genes. Sources of the ceftriaxone-nonsusceptible isolates included urine (352; 73%), blood (43; 9%), respiratory fluid (42; 9%), intra-abdominal fluid (40; 8%), and wound tissue (5; 1%).
Out of the 482 isolates, ESBL and/or p-ampC genes were identified in 376 (78%). Figure 1 indicates the ESBL or p-ampC genes identified in these 376 isolates, separated by species. More specifically, ESBL genes were identified in 310 (82.4%), p-ampC genes in 2 (0.5%), and both ESBL and p-ampC genes in 64 (17.0%) of the 376 organisms. Table 1 displays the β-lactamase genes identified by organism.
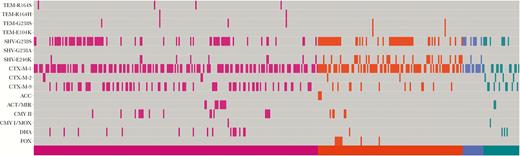
Extended-spectrum β-lactamase and plasmid-mediated ampC genes identified using the Check-MDR CT101 kit (Check-Points, Wageningen, the Netherlands). X-axis shows individual patient isolates sorted by species with Escherichia coli (pink), Klebsiella pneumoniae (orange), Klebsiella oxytoca (blue), and Proteus mirabilis (green). Y-axis shows β-lactamases genes identified.
ESBL and p-ampC Genes Identified in 376 Ceftriaxone-Nonsusceptible Organisms With at Least 1 β-Lactamase Gene Identified; a Large Number of Organisms Had >1 Gene Identified
Genes Identified . | Escherichia coli 220 (58.5%),a No. (%)b . | Klebsiella pneumoniae 112 (29.8%), No. (%)b . | Klebsiella oxytoca 16 (4.3%), No. (%)b . | Proteus mirabilis 28 (7.4%), No. (%)b . |
---|---|---|---|---|
blaCTX-M-typeESBL genes (n = 326c) | 204 | 86 | 12 | 24 |
blaCTX-M-1 group (n = 236) | 138 (62.7) | 74 (66.1) | 12 (75.0) | 12 (42.9) |
blaCTX-M-2 group (n = 10) | 4 (1.8) | 2 (1.8) | — | 4 (14.3) |
blaCTX-M-8/25 group | — | — | — | — |
blaCTX-M-9 group (n = 90) | 70 (31.8) | 12 (10.7) | — | 8 (28.6) |
blaTEMESBL genes (n = 10) | 8 (3.6) | 2 (1.8) | — | — |
blaSHVESBL genes (n = 146) | 70 (31.8) | 60 (53.6) | 8 (50.0) | 8 (28.6) |
Plasmid-mediated ampC genes (n = 66) | 43 | 17 | 0 | 6 |
blaACC (n = 3) | — | 3 (2.7) | — | — |
blaACT/MIR (n = 12) | 10 (4.5) | — | — | 2 (7.1) |
blaCMY-1/MOX (n = 2) | 1 (0.5) | — | — | 1 (3.6) |
blaCMY-2 (n = 21) | 16 (7.3) | 5 (4.5) | — | — |
blaDHA (n = 20) | 16 (7.3) | 1 (0.9) | — | 3 (10.7) |
blaFOX (n = 8) | — | 8 (7.1) | — | — |
Genes Identified . | Escherichia coli 220 (58.5%),a No. (%)b . | Klebsiella pneumoniae 112 (29.8%), No. (%)b . | Klebsiella oxytoca 16 (4.3%), No. (%)b . | Proteus mirabilis 28 (7.4%), No. (%)b . |
---|---|---|---|---|
blaCTX-M-typeESBL genes (n = 326c) | 204 | 86 | 12 | 24 |
blaCTX-M-1 group (n = 236) | 138 (62.7) | 74 (66.1) | 12 (75.0) | 12 (42.9) |
blaCTX-M-2 group (n = 10) | 4 (1.8) | 2 (1.8) | — | 4 (14.3) |
blaCTX-M-8/25 group | — | — | — | — |
blaCTX-M-9 group (n = 90) | 70 (31.8) | 12 (10.7) | — | 8 (28.6) |
blaTEMESBL genes (n = 10) | 8 (3.6) | 2 (1.8) | — | — |
blaSHVESBL genes (n = 146) | 70 (31.8) | 60 (53.6) | 8 (50.0) | 8 (28.6) |
Plasmid-mediated ampC genes (n = 66) | 43 | 17 | 0 | 6 |
blaACC (n = 3) | — | 3 (2.7) | — | — |
blaACT/MIR (n = 12) | 10 (4.5) | — | — | 2 (7.1) |
blaCMY-1/MOX (n = 2) | 1 (0.5) | — | — | 1 (3.6) |
blaCMY-2 (n = 21) | 16 (7.3) | 5 (4.5) | — | — |
blaDHA (n = 20) | 16 (7.3) | 1 (0.9) | — | 3 (10.7) |
blaFOX (n = 8) | — | 8 (7.1) | — | — |
Abbreviations: ESBL, extended-spectrum β-lactamase; p-ampC, plasmid-mediated ampC.
aA large number of organisms have >1 gene identified.
bPercentages in the table use the denominator of the total number of organisms in that column.
cRepresents the number of each specific organism with at least 1 blaCTX-M gene.
ESBL and p-ampC Genes Identified in 376 Ceftriaxone-Nonsusceptible Organisms With at Least 1 β-Lactamase Gene Identified; a Large Number of Organisms Had >1 Gene Identified
Genes Identified . | Escherichia coli 220 (58.5%),a No. (%)b . | Klebsiella pneumoniae 112 (29.8%), No. (%)b . | Klebsiella oxytoca 16 (4.3%), No. (%)b . | Proteus mirabilis 28 (7.4%), No. (%)b . |
---|---|---|---|---|
blaCTX-M-typeESBL genes (n = 326c) | 204 | 86 | 12 | 24 |
blaCTX-M-1 group (n = 236) | 138 (62.7) | 74 (66.1) | 12 (75.0) | 12 (42.9) |
blaCTX-M-2 group (n = 10) | 4 (1.8) | 2 (1.8) | — | 4 (14.3) |
blaCTX-M-8/25 group | — | — | — | — |
blaCTX-M-9 group (n = 90) | 70 (31.8) | 12 (10.7) | — | 8 (28.6) |
blaTEMESBL genes (n = 10) | 8 (3.6) | 2 (1.8) | — | — |
blaSHVESBL genes (n = 146) | 70 (31.8) | 60 (53.6) | 8 (50.0) | 8 (28.6) |
Plasmid-mediated ampC genes (n = 66) | 43 | 17 | 0 | 6 |
blaACC (n = 3) | — | 3 (2.7) | — | — |
blaACT/MIR (n = 12) | 10 (4.5) | — | — | 2 (7.1) |
blaCMY-1/MOX (n = 2) | 1 (0.5) | — | — | 1 (3.6) |
blaCMY-2 (n = 21) | 16 (7.3) | 5 (4.5) | — | — |
blaDHA (n = 20) | 16 (7.3) | 1 (0.9) | — | 3 (10.7) |
blaFOX (n = 8) | — | 8 (7.1) | — | — |
Genes Identified . | Escherichia coli 220 (58.5%),a No. (%)b . | Klebsiella pneumoniae 112 (29.8%), No. (%)b . | Klebsiella oxytoca 16 (4.3%), No. (%)b . | Proteus mirabilis 28 (7.4%), No. (%)b . |
---|---|---|---|---|
blaCTX-M-typeESBL genes (n = 326c) | 204 | 86 | 12 | 24 |
blaCTX-M-1 group (n = 236) | 138 (62.7) | 74 (66.1) | 12 (75.0) | 12 (42.9) |
blaCTX-M-2 group (n = 10) | 4 (1.8) | 2 (1.8) | — | 4 (14.3) |
blaCTX-M-8/25 group | — | — | — | — |
blaCTX-M-9 group (n = 90) | 70 (31.8) | 12 (10.7) | — | 8 (28.6) |
blaTEMESBL genes (n = 10) | 8 (3.6) | 2 (1.8) | — | — |
blaSHVESBL genes (n = 146) | 70 (31.8) | 60 (53.6) | 8 (50.0) | 8 (28.6) |
Plasmid-mediated ampC genes (n = 66) | 43 | 17 | 0 | 6 |
blaACC (n = 3) | — | 3 (2.7) | — | — |
blaACT/MIR (n = 12) | 10 (4.5) | — | — | 2 (7.1) |
blaCMY-1/MOX (n = 2) | 1 (0.5) | — | — | 1 (3.6) |
blaCMY-2 (n = 21) | 16 (7.3) | 5 (4.5) | — | — |
blaDHA (n = 20) | 16 (7.3) | 1 (0.9) | — | 3 (10.7) |
blaFOX (n = 8) | — | 8 (7.1) | — | — |
Abbreviations: ESBL, extended-spectrum β-lactamase; p-ampC, plasmid-mediated ampC.
aA large number of organisms have >1 gene identified.
bPercentages in the table use the denominator of the total number of organisms in that column.
cRepresents the number of each specific organism with at least 1 blaCTX-M gene.
There were 211 (56%), 120 (32%), 41 (11%), and 4 (1%) isolates with 1, 2, 3, or ≥4 ESBL or p-ampC genes identified, with multiple resistance genes found in notable percentages across all 4 species evaluated (Figure 1). Not surprisingly, the majority of β-lactamase genes identified were blaCTX-M genes. Of all blaCTX-M genes, the blaCTX-M-1 group, blaCTX-M-9 group, and blaCTX-M-2 group accounted for 72%, 28%, and 3%, respectively, with some isolates containing >1 blaCTX-M gene. There was notable diversity in the p-ampC genes identified (Table 1), with the most commonly identified p-ampC genes being blaCMY-2 (32%), blaDHA (30%), blaACT/MIR (18%), and blaFOX (12%). Variability in p-ampC genes was observed across organisms, with blaCMY-2 being the most common in E. coli, blaFOX the most common in K. pneumoniae, and blaDHA the most common in P. mirabilis (Table 1). No p-ampC genes were detected in K. oxytoca isolates, although only 16 K. oxytoca isolates were represented in the cohort.
DISCUSSION
Our findings suggest that the underlying mechanisms of resistance contributing to ceftriaxone nonsusceptibility among E. coli, Klebsiella spp., and P. mirabilis are diverse. Of 482 ceftriaxone-nonsusceptible isolates, 376 (78%) had ESBL or p-ampC genes identified, with ESBL genes identified in 82.5%, p-ampC genes identified in 0.5%, and both ESBL and p-ampC genes identified in 17% of isolates. There is a common presumption that most ceftriaxone-nonsusceptible Enterobacterales are proxies for ESBL production and that the ESBLs involved are almost always CTX-M-15 enzymes. Although the blaCTX-M-1 group—which includes blaCTX-M-15—was identified in 63% of isolates for which at least 1 β-lactamase gene was identified in our cohort (based on available targets in the Check-MDR CT101 panel), 24% of isolates contained blaCTX-M-9 group genes—which includes blaCTX-M-14 and blaCTX-M-27 (a single nucleotide variant of blaCTX-M-14). Furthermore, there was considerable diversity with regards to the blaSHV and blaTEM ESBLs identified.
p-ampC resistance remains an underappreciated mechanism of ceftriaxone nonsusceptibility—in part due to the lack of standardized approaches for detection. Based on available investigations, there appears to be a steady rise in the prevalence of p-ampC genes in the United States. Evaluating 1286 isolates from a Veterans Affairs hospital in Virginia from 1995 to 1997, 1%–2% of E. coli, K. pneumoniae, and P. mirabilis species produced p-AmpC enzymes [11]. A more nationally representative US cohort also from the late 1990s identified p-AmpC enzymes in 4%–9% of E. coli and Klebsiella spp. [12]. Data from slightly more than a decade later (2008 to 2010) collected from 3 medical centers in Pennsylvania, Michigan, and Texas found that 17% of ceftriaxone-nonsusceptible E. coli isolates contained the p-ampC gene blaCMY-2 [13]. Organisms infecting children appear to harbor similar proportions of p-ampC genes as those from adult populations; p-ampC genes were identified in 14% of expanded-spectrum cephalosporin isolates from children in Chicago (2011–2015) [14]. Investigators at a children’s hospital in Seattle found a 9-fold increase in Enterobacterales with p-ampC genes from 1999 to 2007 [15].
blaCMY genes are the most common p-ampC identified both in the United States and globally—and most notably in E. coli [13, 14, 16]. In a multicenter cohort of cephalosporin-resistant E. coli isolates from Australia, New Zealand, and Singapore collected as part of the MERINO Trial, 17% of isolates produced p-AmpCs, and >90% of p-ampCs were blaCMY-2 [17]. Data suggest that the proportion of p-ampC genes due to blaCMY might also be increasing. In a cohort of 120 E. coli and K. pneumoniae isolates from Australia that were nonsusceptible to cefoxitin, which is hydrolyzed by p-AmpCs but not ESBLs, 57% of isolates from 2005–2009 contained blaCMY-2 genes vs 85% of isolates from 2013–2014 [18].
Understanding the relative contributions of both ESBLs and p-AmpCs is important as they can directly impact treatment decisions for a number of reasons. First, in the absence of guidance advocating for routine ESBL testing [9], many health care facilities use ceftriaxone nonsusceptibility as a proxy for ESBL production. If Enterobacterales ceftriaxone MICs are ≥2 mcg/mL, patients are often relegated to receiving carbapenem therapy based on clinical trial data indicating that carbapenems are the preferred treatment for ESBL-producing infections [10]. However, not all E. coli, Klebsiella spp., and P. mirabilis with ceftriaxone MICs of ≥2 mcg/mL are ESBL producers. In our cohort of 482 ceftriaxone-nonsusceptible isolates that remained susceptible to carbapenems, 22% had no identifiable ESBL genes, suggesting that escalation to carbapenem therapy may be avoidable for about 20% of patients. This 22% includes a small percentage (<1%) of patients with p-ampC genes (and no ESBL genes identified). Although infectious diseases clinicians tend to favor carbapenem therapy for presumed or proven AmpC-producing infections [19], AmpC-producing organisms are generally more susceptible to cefepime than ESBL-producing ones [20], and the bulk of available clinical studies—although all observational—do not suggest that patients with AmpC-producing Enterobacterales infections have inferior outcomes when prescribed cefepime or piperacillin-tazobactam compared with carbapenem therapy [21–23].
Second, the accepted guidance at many institutions that E. coli, Klebsiella spp., and P. mirabilis nonsusceptible to ceftriaxone are likely to be ESBL producers can be misleading, as it suggests that vigilance for ESBL production is not necessary for other Enterobacterales, when in fact all Enterobacterales have the potential for ESBL production. As an example, estimates of ESBL production among expanded-spectrum cephalosporin-resistant Enterobacter spp., Citrobacter spp., and Serratia spp. from Israel in the early 2000s approached 42%, 24%, and 14%, respectively [24]. Similarly, although p-ampC genes have mostly been recognized in E. coli, Klebsiella spp., and P. mirabilis, there are other Enterobacterales that have acquired plasmids with these genes [13].
Third, for facilities that advocate basing antibiotic treatment decisions solely on susceptibility testing results without inferring potential resistance mechanisms (such as ESBLs), there are also concerns. There will be a portion of Enterobacterales with ceftriaxone MICs ≥2 mcg/mL that are ESBL-producing but retain susceptibility to cefepime and piperacillin-tazobactam [25, 26]. Clinicians may unknowingly prescribe these suboptimal agents for patients with serious ESBL-producing infections, in lieu of carbapenem therapy. All of these issues exemplify why molecular testing for β-lactamase genes can be beneficial in guiding treatment decisions.
There are several limitations to this work. First, we only evaluated isolates in a specific region of the United States, and our results may not be representative of the molecular epidemiology of other regions. Ideally, this work will be repeated using a large, contemporary, and regionally diverse cohort of isolates with representation across the Enterobacterales. Additionally, the Check-Points assay only looks for the most common β-lactamase genes. Although it has been associated with high sensitivity and specificity [27], as with all multiplex assays, it has an inability to detect ESBL and p-ampC genes not included in the platform, including emerging resistance genes. Third, our evaluation was limited to E. coli, K. pneumoniae, K. oxytoca, and P. mirabilis isolates, as these are the organisms for which the prevalence of ESBL genes has been best defined—likely because of previously established methods for phenotypic ESBL detection specific to these Enterobacterales [28]—when in reality all Enterobacterales are at risk of carrying ESBL genes. Finally, we did not identify plasmids circulating among organisms included in the cohort. Plasmid analysis would have been helpful in identifying whether certain ESBL and p-ampC genes identified were frequently co-harbored on the same plasmids, as well as whether additional resistance determinants were also commonly found on the same plasmids (eg, aminoglycoside-modifying enzyme genes, qnr genes, sul genes, etc.).
Overall, our findings suggest that there is value in understanding the molecular mechanisms of resistance that lead to ceftriaxone nonsusceptibility. Although much of the attention has shifted to carbapenemase genes, ESBL and p-ampC genes remain a greater menace—both in health care and community settings. As commercially available diagnostic platforms become increasingly accurate and more cost-efficient and continue to reduce turnaround times, the ability to identify a broad range of resistance genes may have the potential to improve antibiotic decision-making, while simultaneously limiting carbapenem therapy to when it is likely to optimize outcomes.
Acknowledgments
Financial support. This work was supported by funding from the National Institutes of Health K23-AI127935 awarded to P.D.T.; R01-AI104895, R21-AI123747, R21-AI135522 to Y.D.; and an R21-AI130608 awarded to P.J.S.
Potential conflicts of interest. Y.D. has received grants from Accelerate Diagnostics and The Medicines Company and personal fees from Meiji, Achaogen, Allergan, Curetis, The Medicines Company, Roche, Gilead, Pfizer, Tetraphase, Merck, Astellas, and Recida. P.J.S. has received grants and personal fees from Accelerate Diagnostics, grants from BD Diagnostics, Inc., grants from bioMerieux, Inc., grants from Check-Points Diagnostics, grants from BV, grants from Hardy Diagnostics, personal fees from Roche Diagnostics, and personal fees from Opgen Inc. outside the submitted work. All other authors declare no competing interests. All authors have submitted the ICMJE Form for Disclosure of Potential Conflicts of Interest. Conflicts that the editors consider relevant to the content of the manuscript have been disclosed.
References
- antibiotics
- ceftriaxone
- plasmids
- carbapenem
- cefepime
- academic medical centers
- decision making
- enterobacteriaceae
- epidemiology, molecular
- genes
- klebsiella pneumoniae
- proteus mirabilis
- infections
- piperacillin/tazobactam
- dna microarrays
- escherichia coli
- minimum inhibitory concentration measurement
- extended-spectrum beta lactamases
- klebsiella oxytoca
- malnutrition-inflammation-cachexia syndrome
Comments