-
PDF
- Split View
-
Views
-
Annotate
-
Cite
Cite
Lei Wen, Jing Chen, Ji Liang, Feng Li, Hui-Ming Cheng, Flexible batteries ahead, National Science Review, Volume 4, Issue 1, January 2017, Pages 20–23, https://doi.org/10.1093/nsr/nww041
- Share Icon Share
While there has been much recent research on the development of flexible or wearable electronic devices that can be integrated into clothes, glasses, watches and even skin, a bottleneck in this technology appears to be the power source. Thus, one of the most significant issues impeding the success of these newly developed portable gadgets is the development of flexible electrochemical energy-storage devices, which can convert chemical energy and electricity, reversibly. Currently, the most suitable candidates for this purpose are flexible batteries, in particular, lithium-ion batteries (LIBs). Flexible batteries are devices that can work in a range of elastic states and retain electrochemical performance during various deformation processes, such as stretching and bending [1].
Until now, the flexible batteries for which there are the most reports are bendable LIBs. Similarly to the key issues for flexible electronics, the limiting factors of bendable batteries involve electrodes, electrolyte and other components that are conventionally made from rigid materials. In order to obtain a bendable electrode, active materials for LIBs have usually been deposited on a flexible substrate (e.g. paper, cloth, plastic, carbon nanotube (CNT) and graphene films, etc.) to achieve a certain bendability.
One of the major advantages of these non-conductive (paper, cloth and plastic) substrates is their higher elastic values. However, non-conductive substrates usually show poor electrical conductivity and higher side reactions with electrolyte. Compared with non-conductive substrates, CNT- or graphene-based flexible electrodes have obvious advantages in terms of electronic and ionic conductivity, while showing lower stretchability. The electrolyte is another bottleneck for flexible LIBs. To avoid the possible leakage of liquid electrolytes, a suitable gel polymer or solid-state ceramic electrolytes with enough flexibility must be used [2]. For example, gel polymer electrolytes not only have enough mechanical flexibility, but also have excellent interfacial contact, which may make them an ideal component for flexible LIBs [3].
Another kind of flexible LIB is the stretchable one. Stretchable batteries are particularly difficult to achieve, because they have to accommodate not only bending, but also stretching. To endow electrodes with stretchability, two approaches are usually adopted. One is based on the materials themselves, such as various active polymer materials with reversible lithium storage ability and enough stretchability [4]. Unfortunately, this strategy is very challenging and difficult to achieve. The other is to use innovative structural design to make brittle inorganic materials stretchable, such as using wavy, fibrous or island-bridge structure electrodes [4]. These newly developed structures can overcome the elastic limit of brittle materials and open new roads for stretchable devices. The maximum elastic limit of these newly designed stretchable electrodes can be up to ∼1000%. Due to the excellent mechanical properties and electron conductivity of CNTs and graphene, they have great potential to be used to construct stretchable electrodes.
The main shortcoming of the present flexible batteries is their low gravimetric and volumetric energy densities. Another difficulty is the stability of the flexible electrodes, especially on repeated stretching that may eventually produce cracks and fatigue in the electrodes.
Although there are many problems need to be solved, flexible LIBs can be widely used in future electronics. Future flexible LIBs will make wearable products more comfortable and their design more feasible. Figure 1 shows the technological trends and possible applications of future flexible LIBs. Specifically, the following aspects need to be the focus of research.
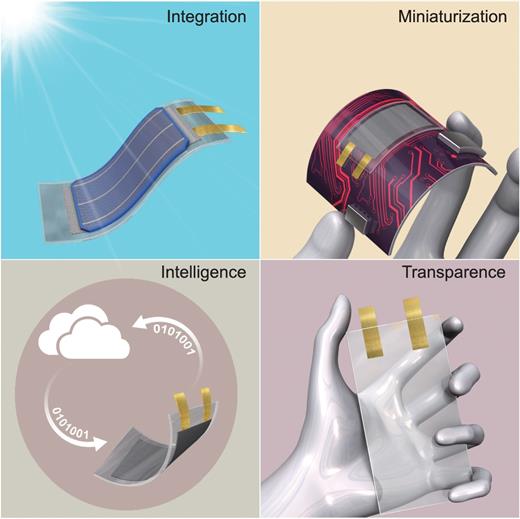
Schematic showing trends of future flexible batteries. Integration: flexible batteries combined with other flexible devices; Miniaturization: flexible batteries with minimum dimensions; Intelligence: flexible batteries with novel functions, such as self-diagnosis and interactive with other smart devices; Transparence: batteries with some visibility.
INTEGRATION
In addition to being directly used as the power supply for flexible devices, future flexible LIBs need to be combined with other flexible devices, such as solar cells, nanogenerators and various sensors to develop integrated equipment. If the electronics and a rechargeable battery can be integrated, the final product could be made more rapidly and seamlessly. For example, Guo et al. [5] combined dye-sensitized solar cells with LIB in an integrated device, which can achieve energy harvest and storage simultaneously. These specific applications require flexible LIBs to have high stretchability, a patterned shape and minimal thickness. Thus, the use of flexible LIBs strongly relies on not only their unique flexibility, but also their multi-functionality. From this point of view, integration or incorporation of multiple functions into one device that can adapt itself to a changing environment is essential in the evolution of flexible LIBs.
MINIATURIZATION
The design of miniaturized electronic equipment, such as micro-robots and implantable medical devices, requires the development of flexible LIBs with minimum sizes. The key feature of miniaturized flexible LIBs is to make them small in both thickness and area on flexible substrates. Current research attempts on ultrathin planar batteries have produced great progress in the fabrication of patterned thin-film electrodes by magnetron sputtering, spinning and layer-by-layer assembly. The thickness of thin-film electrodes is usually less than a few micrometers [6]. For example, Wu et al. [7] developed an in-plane interdigital graphene micro-supercapacitor on flexible substrate through micropatterning of graphene films. Another miniaturized LIB configuration is a 3D micro-battery (typical area of a few mm2), which is especially suitable for on-chip integration and miniaturized electronic devices [8]. To make the 3D micro-battery, Sun et al. [9] precisely interlaced stacks of tiny battery electrodes using 3D printing technology. These micro-batteries are as tiny as one millimeter square but perform as well as commercial batteries.
INTELLIGENCE
Mark Weiser [10] proposed the concept of smart devices, which can function interactively and autonomously to some extent. Future flexible batteries should have the characteristic of intelligence, which is the fundamental and essential concept of the Smart Planet and Internet of Things. Smart and flexible batteries can provide us with more information about their operating states and possess more novel functions, such as self-diagnosis, self-healing, self-control and interactive connection with other smart devices, which can be driven by software. For example, Shan et al. [11] have developed a smart lithium-ion capacitor (LIC) with an additional lithium electrode as a voltage modulator. This smart LIC has the functions of safety monitoring and self-regeneration. Wang et al. [12] also reported a self-heating lithium-ion battery by using an internal Ni foil with one end attached to the negative terminal and the other extending outside the cell to create a third terminal. Once the battery is at low temperature, a temperature sensor attached to a switch causes electrons to flow through the nickel foil. This rapidly warms the inside of the battery.
These new features, endowing electrochemical energy-storage devices with smart functions, can improve user experience. Based on an analysis of a large set of data, their performance indicates they can be very promising candidates for an intelligent power supply for smart, unmanned and on-demand electronics, ranging from nano-grids in devices, micro-grids for households to industrial macro-grids [13].
TRANSPARENCY
Advances in next-generation transparent electronics, such as roll-up touch-screen displays, require the development of transparent flexible LIBs. Unfortunately, conventional LIB components, such as electrode materials, current collectors, separators and packaging materials, are non-transparent. Until now, there have been two strategies for constructing transparent flexible LIBs [14]. For active materials, one common method of making them transparent is to reduce their thickness until it is smaller than their optical absorption length, as demonstrated by depositing a LiMn2O4 or Li4Ti5O12 thin-film on transparent conducting oxides or polymer substrates [15]. However, since the light transmission of materials is attenuated exponentially as the thickness increases, the film of active material has to be extremely thin, which results in the extremely low energy storage of transparent thin-film LIBs. Recently, an innovative design has been reported to use patterned grid-like battery electrodes on clear substrates, which cover only a small part of the whole electrode [14]. This grid-like electrode shows some transparency without severely sacrificing energy-storage capacity.
In order to achieve the above features, it is necessary to precisely design the structure of the electrodes and batteries. Thus, diversified fabrication methods need to be developed, such as spinning, lithography, magnetron sputtering and printing technologies [16]. In the future, the performance of flexible batteries will completely exceed that produced by the conventional slurry-coating fabrication process, and will be increasingly dependent on nano-technology. Ultimately, fully developed flexible batteries could usher in a complete range of portable gadgets, from smart wrist watches to integrated sensors and self-powered micro-robots. We may even expect the next-generation mobile phones to use a flexible transparent body that allows daylight to illuminate the screen and charge the battery.
FUNDING
This work is supported by Ministry of Science and Technology of China (2014CB932402), National Science Foundation of China (51521091, 51525206, 51372253 and 51172242) and Research Supported by the CAS/SAFEA International Partnership Program for Creative Research Teams.