-
PDF
- Split View
-
Views
-
Cite
Cite
Yue Cao, Chia-Lin Tseng, James M. Balter, Feifei Teng, Hemant A. Parmar, Arjun Sahgal, MR-guided radiation therapy: transformative technology and its role in the central nervous system, Neuro-Oncology, Volume 19, Issue suppl_2, April 2017, Pages ii16–ii29, https://doi.org/10.1093/neuonc/nox006
- Share Icon Share
Abstract
This review article describes advancement of magnetic resonance imaging technologies in radiation therapy planning, guidance, and adaptation of brain tumors. The potential for MR-guided radiation therapy to improve outcomes and the challenges in its adoption are discussed.
Computed tomography (CT) has been the standard imaging technique for radiation therapy (RT) simulation, planning, and image guidance, as well as target definition. All these processes are aimed to optimize the delivery of a radiation dose distribution to the tumor target with high precision while maximally sparing the surrounding normal tissue organs at risk (OARs). However, compared with CT, magnetic resonance imaging (MRI) can provide much better definition of both the tumor and OARs due to its superior soft tissue contrast. This is particularly relevant in the central nervous system, where CT is not reliable for either tumor or OAR delineation, and as a result this site was among the first to integrate diagnostic MRI into RT planning. In general, with greater access to diagnostic MRI scanners, the integration of MRI technologies into the RT workflow has been growing rapidly over the past decade.
However, only the latest state-of-art MRI scanners feature functionalities that are more suitable for RT workflow and integration—such as a large-bore magnet to accommodate RT immobilization devices; fast gradients and parallel imaging for high-speed image acquisition; improved signal-to-noise ratio via digitalization of radiofrequency (RF) signals near RF receivers; flat table tops; and flexible RF coil configuration. In addition, recent developments of hybrid MRI–linear accelerator (linac; MRL) systems and their initial introduction into the clinic provide the next step in the integration of MRI in RT by means of real-time MR image-guided dose delivery. Ultimately, the integration of MRI into the overall RT workflow, from simulation to real-time guidance, could potentially improve precision and clinical outcomes and introduce a new paradigm for the field of radiation oncology.
MRI has been used for RT target definition of brain tumors since its introduction in the diagnostic clinic. It has been standard practice to use post-gadolinium (Gd) T1 weighted images and T2 weighted or T2 fluid attenuation inversion recovery (FLAIR) images for brain tumor and OAR delineation, which are fused with CT for RT planning. However, the limitations of conventional MRI for brain tumor target definition are recognized, and much work is ongoing in the areas of physiological and metabolic MRI for better target definition and adaptation of treatment. Also, the role of MRI in sparing functional critical structures and neural networks during RT, to reduce brain toxicity and preserve cognitive function, has been explored and represents a major area of research and development. Furthermore, MRI-only simulation, treatment planning, and real-time guidance (taking CT out of the entire RT workflow) using MRL systems could potentially be cost- and time-saving while reducing the uncertainty associated with CT-MRI registration. Finally, radiographic response assessment of brain tumors treated with RT could benefit from physiological and metabolic MRI.
This article reviews the current state-of-the-art MRI technologies, hybrid MRL systems, and physiological and metabolic MRI, as well as their clinical applications in RT simulation and planning, brain tumor target definition, and RT adaptation based upon assessment of response in tumor and normal tissue. The opportunities to leverage these technologies to reduce health care costs, improve outcomes, and advance the treatment of brain tumors are also discussed, while recognizing the challenges of adoption in mainstream RT clinics.
MRI Alone for RT Simulation and Planning
The dominant role that MRI plays in both standard as well as emerging practices, for precision RT of intracranial targets, is a strong motivating factor for the development of “MRI-only” solutions for patient simulation. The role CT plays in the delineation of target(s) and normal anatomy in treatment planning may be replaced with MRI in most cases; however, the reliance on CT for radiation dose calculation and image-guided patient positioning requires alternate means of support. MRI-only patient modeling for intracranial treatment planning is not new. In fact, MRI has been commonly used without CT for stereotactic radiosurgery (SRS) treatment planning on Gamma Knife (Elekta AB) systems. Instead of using electron densities derived directly from MRI data, a simple geometric model of the head shape is typically derived using a series of depth measurements from a helmet placed on the patient’s head ring.1 The ring is similarly used as the primary positioning aid on such systems as well, with coordinates measured relative to images for mechanical positioning. With appropriate attention paid to distortion,2,3 MRI can play a primary role for SRS targeting.
More recently, MRI systems have evolved to better support radiation therapy treatment simulations with advances including wide bores, support of immobilization devices, and installation of in-room movable lasers.4 While surface coils for imaging have not been fully optimized for operation with typical cranial immobilization devices, MRI “simulators” optimized for dedicated or shared use by radiation oncology departments are gaining prevalence, with well over 100 systems currently in use worldwide. Many of these systems currently support scanning of patients in configurations consistent with their CT simulation and treatment, and the technology is rapidly evolving to directly support MR-based treatment planning and image guidance. MRI is often used in brachytherapy treatment planning, but the needs in external beam RT differ and are being addressed by the generation of synthetic CT models of the patient from one or more MRI volumes.
Synthetic CT Models Derived from MRI (“MRCT”)
MRCT has been a subject of active research and development over the past 6 years. Significant efforts have focused on MRCT generation in the pelvis5–7 and the head.8–10 For intracranial applications, a popular class of MRCT generation algorithms involves atlas-based methods, wherein a population of MRI-CT correlated image volumes or regions is used to optimally select local assignments of CT numbers based on matching criteria. Other methods for MRCT generation have involved direct binary or probabilistic classification of tissues, and assignment of CT numbers from standard values for such tissues. Such solutions have shown sufficient accuracy for dose calculation,11 as well as providing reference images for image-guided patient positioning.12Figure 1 shows an example comparison of MRCT and an actual CT from a solution generated at the University of Michigan.
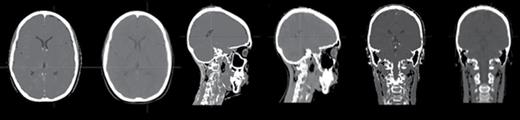
MRCT (left) and actual CT (right) axial, sagittal, and coronal planes of a subject scanned at the University of Michigan.
Intracranial MRI-only simulation for external beam RT does face logistical challenges, which include the availability of MRCT solutions as an integrated part of the simulation RT workflow. Ideally, MRCT representations of the patient should be created at the time of scanning and made available (integrated with the same reference coordinates as the other MRI scans) immediately for direct simulation (eg, placement of fields at the simulator) or treatment planning. At the time of this publication, 2 commercial solutions have been introduced for prostate treatment planning, and at least one solution for intracranial MRCT generation is under development for commercial release.
Management of Geometric Integrity of MRI and Quality Assurance
The geometric accuracy of MRI for brain application in RT needs to be considered, particularly when using MRI alone for simulation and treatment planning. There are several physics effects contributing to geometric distortion in MR images, including both system-level and subject-level sources.13–15 It is important to have adequate management and quality assurance (QA) of these factors during brain scanning to minimize geometric distortion.15–18
Major system-level factors affecting geometric accuracy of MR images include homogeneity of the B0 magnetic field, linearity of gradients, k-space sampling of pulse sequences, and shimming control. The B0 magnetic field in the scanner bore is not perfectly uniform even after passive and active shimming provided by vendors. The uniformity of the B0 magnetic field decreases with the distance from the isocenter. However, the effect of the B0-field inhomogeneity on image geometry decreases with decreasing field strength, as well as with increasing readout bandwidth. Magnetic gradients are not built linearly, which causes miscoding of spatial information and thereby image distortion. The gradient nonlinearity effect increases with the distance from the isocenter. In general, vendors provide software tools to perform gradient nonlinearity correction either in 3D or 2D fashion to improve image geometric integrity. For scanning a head positioned in the isocenter, geometric distortion in MR images due to system-level factors is most likely less than 1 mm18 if the magnet is well shimmed, a conventional k-space sampling (non-echo planar) pulse sequence is used, and gradient nonlinearity correction is applied. However, due to the flexibility of MRI scanners and especially their uses in radiation planning, a quality control program needs to be in place to ensure that these conditions are met.
There are several patient-level factors influencing geometric accuracy of MR images. One is the patient-induced susceptibility effect. Putting a human subject in a magnet can disrupt uniformity of the field due to this effect. This disrupted field is particularly observed in the inferior frontal and anterior temporal lobes near the skull base due to magnetic susceptibility changes at the boundary of soft tissue/bone and air.17 This effect is greater at higher magnetic field strengths (eg, 3 Tesla). The distortion caused by this effect is not much greater than 2–3 mm if using a conventional k-space sampling pulse sequence with a readout bandwidth of 200 Hz or greater at 3 Tesla (T).17 However, this distortion, while starting at the boundary of tissue/bone and air, persists into the tissue in a slow decay fashion.17 This distortion can be mitigated using a low magnetic field scanner, high readout bandwidth, or parallel imaging, with tradeoffs on image contrast and signal-to-noise ratio. It is important to note that this patient-induced susceptibility effect cannot be calibrated using a water phantom. Another patient-related effect is the chemical shift effect of fat, which can cause fat signals to be physically displaced from water signals. Again, this effect can be mitigated by increasing readout bandwidth or voxel size to keep the chemical shift within a single voxel. Also, various techniques can be applied to suppress undesirable fat signals (eg, fat saturation, water and fat separation using Dixon methods, water signal only excitation).
Finally, different k-space sampling pulse sequences can mitigate or amplify the B0-field inhomogeneity effect (including both patient-induced and system-level) on geometric accuracy of MR images. For example, with the same level of B0 inhomogeneity, echo planar imaging (EPI), which is commonly used for diffusion weighted images, causes much more geometric distortion and signal loss than conventional k-space (line sampling) pulse sequences.19 Use of a segmented multi-shot EPI and/or a high parallel imaging factor can reduce this geometric distortion.20 High-order individual patient shimming, if available, can help reduce the patient-caused B0 inhomogeneity. However, it is important to be aware that changing a shimming setting from one image series to the next can cause uncertainty in image geometry. Locking the shimming setting during an imaging session could reduce this uncertainty.
Methods for correcting the geometric distortion in MR images caused by the patient-induced susceptibility effect have been proposed.13–17 These methods include corrections applied in k-space and in image domains. Robustness of the correction methods needs to be determined. Individual patient B0-field mapping should be considered as a QA tool to estimate whether the B0-field inhomogeneity causes any distortion greater than a tolerance. This B0-field acquisition adds only a minute or less to the total scan time. The B0 inhomogeneity map computation can be robust and easy when phase wrapping is avoided by extending the B0 dynamic range using optimal echo spacing.
Although geometric distortion in MR images is of potential concern, it can be managed. QA of geometric accuracy of MR images should be implemented as an automated procedure and integrated into RT workflow.
Other Considerations for MRI-Only Radiotherapy Simulation
Beyond the issues listed above, the nascent state of MRI-only simulation in the head can be further advanced by the provision of more convenient coil systems for scanning immobilized patients. The evolution of RT-specific QA guidelines and processes for MR simulation will also help spread the adoption of MR simulators, as well as recommendations for scanning sequences optimized specifically for radiation oncology applications.21 Proper education and training of staff in MR safety and scanning, as well as RT simulation, is required. In addition, some access to MR-trained physicists is important to maintain at least some elements of specification, commissioning, and routine scanner QA. Many of these issues are currently being addressed in a task group (TG284) of the American Association of Physicists in Medicine.
Physiological and Metabolic Imaging for Glioblastoma Target Definition and Adaptation
Conventional MRI, post-Gd T1 weighted, and/or T2 FLAIR are standard images used in brain tumor delineation, including high- and low-grade gliomas and brain metastases, for RT planning. However, in recent years, limitations of conventional MRI for target definition of glioblastoma (GBM) have been recognized, and the roles of metabolic and physiological imaging (eg, proton MR spectroscopic imaging [1H-MRSI], perfusion, water diffusion imaging) for target definition have been investigated. To use these advanced imaging techniques to define the GBM target, several scientific questions need to be addressed, such as whether these metabolic/physiological imaging modalities detect abnormalities in GBM beyond those detected by post-Gd T1 and T2 FLAIR images, whether the detected abnormalities represent true tumor, and whether the detected abnormalities carry any prognostic significance. Finally, are these technologies ready to be integrated into the RT clinical workflow?
Proton Spectroscopic Imaging
Proton MRSI is a technique that can detect chemical compounds and metabolites in brain tissue, including choline-containing compounds, creatine, lactate, lipid, and N-acetylaspartate (NAA). Evidence to support that GBM has abnormally increased choline and lactate but decreased NAA and creatine compared with normal tissue has been accumulated over decades.22,23 The increased choline in brain tumor is thought to be associated with cell membrane phospholipid turnover resulting from tumor cell proliferation and cell density.24–27 A ratio of choline to NAA (Cho/NAA) in tumor, normalized to the contralateral normal tissue, is often used as a semi-quantitative metric to define the metabolic abnormality in GBM.22,24,28 However, there is no consensus on the cutoff threshold. Although elevated lactate is suggested to be a unique marker for hypoxia and tumor radiation resistance,29,30 it is less frequently used in the studies. Nevertheless, the metabolic abnormality volume of Cho/NAA in GBM is often observed beyond the enhanced lesion and sometimes even beyond the FLAIR abnormality.28,31,32 Also, there are many studies reporting that the non-enhanced Cho/NAA abnormal areas prior to treatment are coincident with newly enhanced lesions after chemoradiation therapy.33 Overall, the pretherapy metabolic abnormality volume defined by Cho/NAA predicts overall survival (OS) and progression-free survival (PFS) in GBM.22,28,30,34 An increase in Cho/NAA during the mid-course of RT is associated with a greater chance for early progression.28 However, few prospective studies investigate whether intensified radiation treatment of the Cho/NAA abnormal volume leads to better outcomes. Early retrospective studies show that missing the majority of the abnormal Cho/NAA volume in the recurrent GBM (eg, >50%) by high dose radiation tends to result in worse survival.35,36
With all the promises discussed, why is this technique still not deployed in clinical trials and translated into clinical practice after decades of investigations? The challenges of this technique include both spectral acquisition and quantification. Although long acquisition and low spatial resolution of 1H-MRSI have been pointed out as major obstacles, for which recent improvement has been reported, robustness of spectral acquisition, the ability to consistently obtain high-quality spectral images, could be the first major challenge in using this technique in a clinical trial. The second major challenge is spectrum quantification. Automated software tools that are provided by almost all scanner vendors cannot produce consistent high-quality spectrum quantification. Finally, creating spectral images in DICOM format and importing them in a treatment planning system are necessary steps in the RT workflow.
Perfusion and Cerebral Blood Volume MRI
Dynamic susceptibility contrast (DSC) and dynamic contrast enhanced (DCE) MRI are techniques for quantitative estimations of cerebral blood volume (CBV), cerebral blood flow (CBF), vascular leakage, and mean transit time.37,38 GBM exhibits neovascularization, an aspect of tumor growth, resulting in increased CBV, CBF, and vascular leakage.39–43 Studies show that elevated CBV and CBF in malignant gliomas are associated with worse OS and PFS,40,41,43–45 suggesting that CBV and CBF are prognostic biomarkers. In a retrospective study with 189 patients with gliomas, elevated mean relative (r)CBV values (>1.75) in tumors are significantly associated with shorter time to progression for both low- and high-grade gliomas.40 Similarly, high vascular leakage, quantified by transfer constant of Gd-DTPA (diethylenetriamine pentaacetic acid) (Ktrans) to the tissue is a significant predictor of OS.46 Most interestingly, when testing prediction of OS in GBM using the Verhaak molecular classification, adding the maximum tumor CBV in the model can improve the prediction power.47 Also, a study shows that a threshold metric of rCBV correlates with histological tumor fraction and OS in recurrent GBM.48 It is known that GBM is a highly heterogeneous tumor, therefore there are some limitations in using mean or median values of CBV and CBF in the whole tumor volume, which potentially translates to reduced sensitivity of the metrics for response assessment. Several approaches have been proposed to address this problem—for instance, classifying the whole tumor volume into a few distinct subvolumes based upon different vascular leakage or CBV values,41,43 or a voxelwise approach to create a parametric response map by comparing CBV prior to and during RT.49 All of these non‒mean value‒based approaches seem to lead to better prediction for outcome.41,43,49,50 The subvolume-based approach has the potential to identify the boosting target if the subvolume represents an aggressive or nonresponsive component of the tumor.
Before using elevated CBV to define the boost target in GBM, to what extent elevated CBV in GBM represents tumor needs to be addressed. A preliminary study suggests that tumor can be beyond the elevated CBV region in GBM.51
As discussed, both DSC and DCE MRI can be used to estimate CBV. However, DSC MRI acquired by EPI is subject to severe susceptibility-induced geometric distortion and signal loss. As an alternative, CBV can be derived from T1-weighted DCE MRI.38 Again, determining a reliable arterial input function is still a key issue. However, using a large sagittal field of view could allow determination of an arterial input function in a carotid artery (a large artery) and thereby increase reliability. To integrate the CBV computation in the RT workflow, an automated software tool with sufficient validation is highly desirable.
Diffusion MRI
Diffusion MR imaging is a technique to measure water molecule mobility in the tissue microscopic environment, which is sensitive to cell density and size, cell membrane permeability, extracellular space tortuosity, and water content. Preclinical studies show an inverse correlation between apparent diffusion coefficient (ADC) and cellularity in the brain tumor.52 Restricted diffusion of water has been explored as an indicator for solid tumor in malignant gliomas. However, the mixture of high cellular tumor, normal tissue, edema, and micronecrosis in GBM results in unpredictable and frequently elevated ADC values compared with normal tissue when quantified from 2-point diffusion weighted MRI with b-values of 0 and 800–1000 s/mm2. As a consequence, investigators have sought to use the minimum ADC value in malignant gliomas and find its prognostic value for OS.53–55 However, the regions with minimum ADC within GBM can severely underestimate the extent of solid tumor, underscoring the critical question of whether it can be used as a boosting target. A variation of this approach is to analyze the low ADC segment of the bi-distribution histogram of the whole tumor,56 which is more rigorous than looking for an arbitrary “minimum” ADC. Another alternative is to use high b-value diffusion weighted images (eg, 3000–4000 s/mm2) to attenuate signals from edema.57–60 A recent study shows that a hypercellular subvolume (HCV) of GBM identified by high b-value (3000 s/mm2) diffusion weighted imaging (DWI) predicts PFS, and approximately 40% of the HCV are non-enhanced, which may fall outside of the typical high dose volume,60 suggesting a potential boost target.
A limited number of studies investigated the early response of GBM during RT using diffusion weighted images,61,62 which could provide information for individual patient adaptation of RT. In the studies, the patients with a large volume of voxelwise ADC changes during week 3 of RT compared with pre-RT are associated with a better response to RT.61,62 The challenge in this approach is the consistent production of high quality registration of paired images acquired prior to and during RT when there is tumor growth or shrinkage.63
There are some technical challenges to using diffusion images (including ADC maps) to define a boost or adaptive target. First, most diffusion images are acquired by a single-shot EPI pulse sequence, which is sensitive to susceptibility-induced geometric distortion. Several strategies can be used to reduce EPI-related geometric distortion, such as using multi-shot EPI, readout segmented multi-shot EPI, and a high parallel imaging factor. Ultimately, it is important to pathologically validate high b-value DWI and parametric response mapping for target definition in GBM.
Given that GBM is a heterogeneous tumor, multiparametric imaging modalities or contrasts are necessary to better characterize the tumor and assess therapy response. However, further investigations are required to establish the definitive roles of multiparametric imaging modalities.
Assessment of Treatment Failure After Concurrent Chemoradiotherapy in GBM
In the era of concurrent RT with temozolomide (TMZ) followed by adjuvant TMZ for GBM treatment, pseudoprogression, which has been defined as new areas of enhancement that arise from therapy-related inflammation and increased vessel permeability but not tumor progression, occurs in 20%–30% of patients.64 It is hard to distinguish pseudoprogression from true progression by enhancement alone or even using Response Assessment in Neuro-Oncology criteria. Misdiagnosis could lead to premature termination of an effective therapy and unnecessary surgery or additional chemotherapies. In case of irradiation of recurrent GBM, target definition based upon enhancement is also problematic.
CBV, DWI, and 1H-MRSI have been investigated in both prospective and retrospective studies for aiding in values for identifying pseudoprogression from real progression in GBM post-RT. Overall, rCBV in new or enlarged enhanced regions has a higher value for true progression compared with pseudoprogression.7,65,66 A wide range of cutoff threshold values of normalized rCBV, 1.47–3.19, have been reported which are able to achieve 80%–87% sensitivity and 77%–90% specificity.7,65,66 Another approach is to predict progression within the most likely period of pseudoprogression, 1–3 months post-RT, using parametric response map analysis of rCBV acquired pre-RT and in week 3 during RT.67 However, a consensus cutoff threshold value of rCBV needs to be established and tested using multicenter data for differentiating true progression from pseudoprogression.
Considering that GBM progression can be non-enhancing, and anti-angiogenesis drugs can alter tumor vasculature dramatically,68 nonvascular-based imaging such as diffusion and proton spectroscopy could be useful in differentiating true progression from pseudoprogression. Although they can be altered by edema effects due to vascular leakage caused by either treatment effect or tumor recurrence, ADC values (either mean, minimum, or maximum) are generally lower in recurrent gliomas.69,70 To overcome the edema effect on ADC, high b-value (>1000 s/mm2) DWI has advantages. In a retrospective study that compares the 5th percentiles of ADC maps calculated from b-values of 0 to 1000 and 0 to 3000 s/mm2 (ADC1000 and ADC3000) for distinguishing progression from pseudoprogression in GBM, ADC3000 compared with ADC1000 increased diagnostic accuracy of true progression to 88.9% from 66.7%.59Figure 2 shows consistent results from high b-value DWI and proton spectroscopy imaging for identifying tumor recurrence from newly enhanced lesions, in which the first technique is much easier to translate into clinic than the latter one. There is a caveat to identifying recurrent GBM after treatment with bevacizumab using minimum ADC,71 as both coagulative necrosis and recurrent tumor show restricted diffusion (low ADC). But the two can be differentiated by perfusion, since coagulative necrosis has very low CBV.72 Also, abnormal metabolism in recurrent GBM measured by proton spectroscopy, commonly expressed as ratios of Cho/NAA, NAA/creatine, and Cho/normal creatine, have been investigated for differentiating treatment effects from recurrence.23,73–78 Significant differences in the metabolite ratios are found between recurrent tumor and tissue with therapy effect with various cutoff thresholds. Due to many discussed challenges, MRS is not yet for routine clinical use. As expected, multiparametric imaging shows improved power in distinguishing pseudoprogression from true progression in GBM—for instance, combining rCBV and conventional ADC.7,70
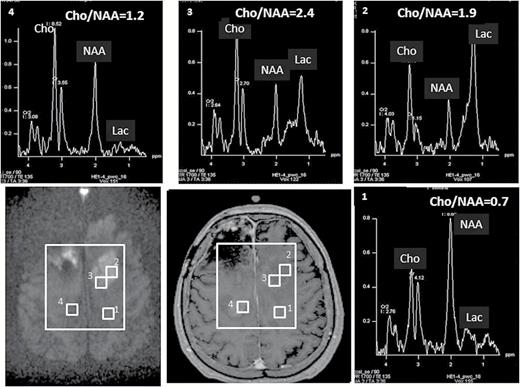
Two new enhanced lesions were observed in a GBM 19 months post-RT (middle panel on the second row). High b-value diffusion imaging (b = 3000 s/mm2, left panel on the second row) indicated that the lesion in the left frontal lobe had hypercellularity suggesting tumor recurrence, while the lesion in the right frontal lobe had cellularity similar to normal tissue suggesting treatment effect. Results from high b-value DWI are similar to proton spectroscopic imaging, as elevated Cho/NAA ratios (2.4-1.9) and increased lactate (2 right panels on the first row) were observed in the left lesion but close to normal Cho/NAA ratio (1.2) and minimum lactate in the right lesion (left panel on the first row). Note that high b-value diffusion imaging only takes approximately one minute to acquire.
MRI in Management of Brain Metastasis for RT
Currently, radiation options for brain metastatic tumors include whole brain radiotherapy (WBRT) and SRS, and the optimal approach mainly depends on the number of metastases and prognosis of primary cancers.79 Accurate diagnosis of brain metastases, particularly for small lesions on across-sectional post-Gd T1 weighted MRI, could be a challenging task for radiologists. Misdiagnosis can affect the decision on selecting the appropriate modality of RT. Computer-aided detection could help reduce misdetection of small metastatic lesions.80 Evidence shows that response of metastatic lesions to WBRT or SRS is highly heterogeneous between patients and even between lesions within the same patient.81 Identifying a nonresponsive lesion to RT earlier could provide an opportunity to locally boost the lesion. Also, establishing imaging biomarkers in brain metastases in response to WBRT or SRS could aid in testing combining RT with new chemo- or immunotherapy drugs in the future. Currently, there are limited studies reporting the use of physiological and metabolic imaging to assess the early response of metastasis to WBRT or SRS. Diffusion imaging has the same issues in brain metastasis as in GBM, such as alteration of ADC by edema. High b-value DWI (b-value up to 4000 s/mm2) combined with a bi-exponential model have shown some success for predicting therapy response.58 Also, at conventional b-values such as 1000 s/mm2, a diffusion abnormality index, accounting for both edema and tumor cell responses to radiation, has been shown to be a significantly better predictor for treatment response than changes in mean ADC in metastatic lesions at the end of RT.82 CBV and Ktrans derived from DCE MRI provide tumor vascular response to radiotherapy. Studies show that early decreases in CBV and Ktrans after WBRT and SRS indicate tumor response to therapy.81,83,84 In a study of 25 patients with brain metastases treated with SRS, chemical exchange saturation transfer (CEST), an MRI method sensitive to the presence of proton pools exchanging with the bulk water protons, has demonstrated the ability to predict volumetric changes in the treated lesions as early as 1 month post-SRS.85 Future extension of this work may permit the use of CEST metrics as biomarkers of tumor response for targeted radiotherapy.
Assessment of Blood‒Tumor Barrier Permeability After RT
The effectiveness of systemic therapies in brain tumors is affected by whether drugs can cross the blood‒tumor barrier (BTB) and reach the tumor cells. It has been shown that radiation can open the BTB.42,86 Monitoring BTB opening in the clinical setting would aid in selecting the optimal time to deliver chemotherapy, thereby potentially improving the treatment of brain tumors. The permeability of the BTB can be evaluated quantitatively by the amount of contrast agents accumulated in the extracellular extravascular space through the BTB by Ktrans derived from DCE MRI. Permeability of brain tumor vasculature exhibits great heterogeneity prior to RT, as well as responding to radiation heterogeneously. In a study of permeability changes in GBM and normal brain vasculature by contrast enhanced MRI,42 it was found that Gd-DTPA uptake in a pre-RT non-enhanced tumor region is increased but not in normal brain after irradiation, suggesting a radiation-induced BTB opening. This BTB opening has a time window between 1 week after the start of RT and 1 month post-RT, during which chemo agents have maximum access to the tumor. Radiation effects on the BTB in brain metastases seem to be more complex, as Ktrans has either a mild decrease or an increase depending upon initial vascular conditions.87 DCE MRI is a valuable tool for assessing the time window for BTB opening in response to RT in brain metastases. However, due to the small molecule size of Gd-DTPA, BTB permeability for Gd-DTPA does not automatically imply permeability for drugs with large molecular sizes. Currently, there is no FDA-approved large molecule contrast agent for clinical use.
Assessment of Radiation-Induced Cognitive Dysfunction
Cognitive dysfunction has been reported after brain RT,88,89 which affects quality of life of long-term survivors.90 Interestingly, there is no apparent lesion in the brain associated with the majority of cognitive functional decline. The mechanisms remain unclear.91 Therefore, as a surrogate, measuring subtle physiological and metabolic changes in brain tissue as well as dose response after brain irradiation could provide guidance for RT delivery and preserve brain function. 1H-MRSI has been shown to be able to detect metabolic changes in normal brain after RT, showing decreased NAA and increased Cho,92–95 including in the hippocampus.96 Also, an early vascular response to radiation in the hippocampus using DCE MRI demonstrates an association with delayed cognitive functional decline.97 Diffusion tensor MRI (DTI), the best technique to assess white matter integrity, has shown associations of DTI index changes in white matter with radiation-induced cognitive decline.98–100 Interestingly, white matter fiber bundles have different responses to the same radiation doses, indicating regional variation in radiation sensitivity.101,102 Moreover, elongated white matter fiber bundles seem to be sensitive to the maximum dose exposed.103 Sex and age could also be factors for different sensitivities of brain tissue to radiation doses.97 Drugs, such as TMZ, could also affect brain function. In summary, DCE and DTI acquisitions can be integrated into a scan for both target definition and assessment of normal tissue response to RT. Additional studies are needed to further understand brain tissue changes after irradiation and their associations with cognitive deterioration.
MR Image Guidance Integrated in Modern External Beam Radiotherapy
Kilovoltage cone-beam computer tomography (CBCT) has been the dominant online image-guided radiotherapy (IGRT) tool for nearly a decade to ensure high precision in patient setup prior to radiation delivery104; however, it is limited in that it cannot perform beam-on intrafractional tumor-specific positional verification. Moreover, the lack of soft tissue contrast has resulted in pre-treatment position verification and error correction primarily based on registration to the bony anatomy and/or fiducial markers. Although delivery devices such as the Cyberknife (Accuray) provide near real-time imaging with a feedback mechanism to the robotic arm during irradiation, its biplanar radiography must again rely on bony structures or internally placed fiducial markers as opposed to the true soft tissue 3D volume.
Online MR IGRT provides superior soft tissue contrast allowing for visualization of the 3D tumor volume during delivery and the added benefit of not exposing the patient to additional radiation associated with CBCT imaging. Improved certainty in anatomical changes facilitates accurate dose reconstruction and accumulation throughout the treatment course, which is the path to routine adaptive RT practice. Furthermore, the introduction of beam-on MRI has the potential to revolutionize radiation delivery, as direct monitoring of anatomical changes allows for real-time adaptive RT based on tumor response, deformation, organ filling, and movement associated with physiologic motion. Therefore, the next paradigm of individualized treatment margins and reduction in dose to normal tissue can be realized. Finally, implementation of online functional MRI sequences could enable identification of potential image-based biomarkers of tumor response, and consequent biologically based treatment adaptation.
Hybrid MR-Radiotherapy Systems in Clinical Use or in Preclinical Development
There are currently 3 integrated systems that have incorporated an MRI with a radiation delivery system. A single integrated “hybrid” system achieves the goal of beam-on imaging to allow tumor tracking and potential radiation adaptation in real time. Table 1 summarizes the key technical features of current preclinical or commercial hybrid MR IGRT systems. In brief, the collaboration between Elekta AB, Philips, and the University Medical Center Utrecht yielded a hybrid MRL consisting of an integrated 70-cm-bore 1.5 T Philips MRI and a 7-megavoltage (MV) linac mounted on a ring-shaped gantry in the mid-transversal plane of the MRI.105–108 This design is unique in that it incorporates modern linac technology with a clinical high field strength MRI (1.5 T), which results in improved signal-to-noise ratio. This clinical-grade high field strength MRI will permit MR spectroscopy and other functional imaging sequences at the level of diagnostic imaging standards. Installation of the first-generation system in Utrecht began in 2014, and at the time of this report, each of the 7 members of the international Elekta MRL consortium have begun installation.109
Key technical features of current preclinical or commercial hybrid MR-guided radiotherapy systems
Hybrid MR–RT system . | MRI . | Treatment unit . | Magnetic field orientation relative to incident radiation . | Development status . |
---|---|---|---|---|
Viewray MRIdian System109,110 | 70 cm bore, 0.35 T split superconducting magnet | Robotic 3-headed 60Co system each equipped with 30 leaf pairs of doubly focused multileaf collimators providing a combined dose rate of 550 cGy/ min at isocenter | Perpendicular | Commercial; linac-based MR-radiotherapy system European regulatory approved, pending US approval |
Elekta MR-linac104–107 | 70 cm bore 1.5 T Philips MRI with active magnetic shielding | 7-megavolt linac (intensity-modulated RT) | Perpendicular | Preclinical; installation in progress within Atlantic Consortium108 |
Rotating biplanar linac–MRI system115,116 | 60 cm open bore 0.6 T superconducting whole-body magnet | 6-megavolt linac | Parallel | Research; commercialization in progress (MagnetTx) |
Hybrid MR–RT system . | MRI . | Treatment unit . | Magnetic field orientation relative to incident radiation . | Development status . |
---|---|---|---|---|
Viewray MRIdian System109,110 | 70 cm bore, 0.35 T split superconducting magnet | Robotic 3-headed 60Co system each equipped with 30 leaf pairs of doubly focused multileaf collimators providing a combined dose rate of 550 cGy/ min at isocenter | Perpendicular | Commercial; linac-based MR-radiotherapy system European regulatory approved, pending US approval |
Elekta MR-linac104–107 | 70 cm bore 1.5 T Philips MRI with active magnetic shielding | 7-megavolt linac (intensity-modulated RT) | Perpendicular | Preclinical; installation in progress within Atlantic Consortium108 |
Rotating biplanar linac–MRI system115,116 | 60 cm open bore 0.6 T superconducting whole-body magnet | 6-megavolt linac | Parallel | Research; commercialization in progress (MagnetTx) |
Key technical features of current preclinical or commercial hybrid MR-guided radiotherapy systems
Hybrid MR–RT system . | MRI . | Treatment unit . | Magnetic field orientation relative to incident radiation . | Development status . |
---|---|---|---|---|
Viewray MRIdian System109,110 | 70 cm bore, 0.35 T split superconducting magnet | Robotic 3-headed 60Co system each equipped with 30 leaf pairs of doubly focused multileaf collimators providing a combined dose rate of 550 cGy/ min at isocenter | Perpendicular | Commercial; linac-based MR-radiotherapy system European regulatory approved, pending US approval |
Elekta MR-linac104–107 | 70 cm bore 1.5 T Philips MRI with active magnetic shielding | 7-megavolt linac (intensity-modulated RT) | Perpendicular | Preclinical; installation in progress within Atlantic Consortium108 |
Rotating biplanar linac–MRI system115,116 | 60 cm open bore 0.6 T superconducting whole-body magnet | 6-megavolt linac | Parallel | Research; commercialization in progress (MagnetTx) |
Hybrid MR–RT system . | MRI . | Treatment unit . | Magnetic field orientation relative to incident radiation . | Development status . |
---|---|---|---|---|
Viewray MRIdian System109,110 | 70 cm bore, 0.35 T split superconducting magnet | Robotic 3-headed 60Co system each equipped with 30 leaf pairs of doubly focused multileaf collimators providing a combined dose rate of 550 cGy/ min at isocenter | Perpendicular | Commercial; linac-based MR-radiotherapy system European regulatory approved, pending US approval |
Elekta MR-linac104–107 | 70 cm bore 1.5 T Philips MRI with active magnetic shielding | 7-megavolt linac (intensity-modulated RT) | Perpendicular | Preclinical; installation in progress within Atlantic Consortium108 |
Rotating biplanar linac–MRI system115,116 | 60 cm open bore 0.6 T superconducting whole-body magnet | 6-megavolt linac | Parallel | Research; commercialization in progress (MagnetTx) |
The MRIdian system by Viewray is another integrated MR IGRT system, which combines a split-bore double-donut 0.35 T MRI and a robotic 3-headed cobalt-60 (60Co) system, each equipped with 30 leaf pairs of doubly focused multileaf collimators.110,111 A pilot study has demonstrated the feasibility of performing diffusion MRI on the MRIdian system to assess tumor response.112 The performance of the system has been reported, and shown to generate clinically acceptable plans with a minor increase in planning target volume heterogeneity, and OAR mean doses, concomitant with an increase in low dose volumes compared with linac plans.113,114 It received regulatory approval in 2012 and is currently the only hybrid MR–RT clinical system in operation. The company has recently furthered its efforts and developed a 6-MV linac-based MRI-guided radiation system which has received regulatory approval in Europe and is awaiting approval in the United States.115
The group at the Cross Cancer Institute, Alberta, Canada, demonstrated a different hybrid design using a biplanar 0.2 T MRI system integrated with a 6-MV accelerator.116 A recent update to this design has interfaced the accelerator with a superconducting whole-body (0.6 T) open-bore magnet, and MagnetTx Oncology Solutions has secured rights to commercialize this technology.117 The design is based on fixed geometry of the accelerator and the MRI, allowing irradiation either between the magnet planes or through the central opening of one of the planes. Consequently, in this framework, the accelerator must rotate together with the MRI around the target when radiation is delivered from multiple beam angles.
Technical Considerations in Hybrid MR-Radiotherapy Systems
The primary challenge in the design of a linac-based hybrid MRL system is the minimization of the magnetic and RF interferences between the MRI and the accelerator. This decoupling of the MRI from the accelerator is achieved in the Elekta MRL using active magnetic shielding to create a low-field toroid in the transversal mid-plane and thereby generate a zero magnetic field at the gun and minimal field at the accelerator tube. Decoupling effectively allows continued operation of the accelerator in the presence of the MRI and minimizes the accelerator-induced geometric distortions in the MRI. A redesigned Faraday cage positions the accelerator outside of the cage to manage RF interferences between the accelerator and the MRI. The gradient coil has been split and superconducting coils set aside to allow the beam to transverse through a homogeneous section of the cryostat in the mid-plane circumferentially. The use of radioactive sources such as that in the Viewray system minimizes RF interference on the operation of the MRI unit, and conversely the performance of the radioactive sources are not affected by the stray magnetic field of the MRI. However, 60Co gamma rays relative to MV linac beams produce lower output, larger penumbra, less penetration, and higher surface doses.118 Moreover, compromise is made with a relatively low field MRI which is known to provide images with a lower signal-to-noise ratio and shorter relaxation times but with the benefit of diminished magnetic susceptibility artifacts and therefore potentially improved spatial accuracy. The updated design of the system, which incorporates a 6-MV linac, achieves the decoupling of the MRI from the accelerator as well as RF minimization via compact passive shielding. The Cross Cancer Institute MRL employs simple active shielding combined with finite element method–based passive shielding to magnetically decouple the linac.119 The system uses an RF cage that houses both the MRI and the linac and has been reported to effectively eliminate undesired RF noise from the linac to permit full recovery of imaging signal-to-noise ratio in the MRI.120
Dose Delivery within a Magnetic Field
In the presence of a high field strength magnetic field oriented perpendicular to the incident photon beam, the dose distribution may be affected by the Lorentz force due to deflection of secondary electrons, resulting in a curved trajectory of the electrons. The consequence is a shallower buildup, and a shifted asymmetrical penumbra.121 Furthermore, the electron return effect (ERE) is observed at interfaces between 2 materials with large density differences, such as between air and tissue, where the mean free path of electrons is longer in the less dense material (ie, air), thus allowing electrons leaving from tissue to air to traverse back into skin in a helical fashion.122,123 The repercussion of this phenomenon is increased exit skin dose, which is also dependent on the orientation of the exit surface.124 Raaijmakers et al have shown that a multibeam intensity-modulated RT approach may be utilized to reduce the unintended dose deposition around air cavities, and the dose distribution differences for targets adjacent to air cavities in the absence and presence of a 1.5 T magnetic field is minimal.125 In a brain metastasis planning study of clinical patients, Tseng et al demonstrated that the dosimetric impact of ERE at tissue/air boundaries is minor and does not negatively impact target conformity or dose gradient.126 A recent paper by Chen et al quantified the ERE effects at tissue/air and tissue/lung boundaries using the Monaco treatment planning system. The authors found that the optimization algorithm in combination with a multibeam arrangement could substantially mitigate the ERE.127 The largest effects were observed for whole breast irradiation and a small number of beam angles.127–129 The effects of the Lorentz force reduces at lower magnetic field strengths, and only minor perturbations of the dose distributions and surface doses are seen in the Viewray system.129,130 An inline orientation of the magnetic field parallel to the direction of the accelerator beam, such as that seen in the Cross Cancer Institute MRL design, mitigates the ERE but at the expense of potential significant increases in entrance skin dose as electron contamination from the accelerator is encouraged to travel toward the patient surface.130–132 Backscatter dose effects have been quantified for higher atomic number materials (such as bone, dental fillings, stainless steel, and titanium) in the presence of a transverse magnetic field, where a reduction in dose at the interface was observed in contrast to the expected increase in the absence of a magnetic field.133 In all cases, the dose calculation engine must be able to accurately take into consideration the presence of a permanent magnet field and the interface effects, and to this end, a fast graphics processing unit–based Monte Carlo dose calculation platform has been developed and validated in the presence of a magnetic field.122,134,135
Application of MRL to Central Nervous System Indication and the Challenges Ahead
For the treatment of brain tumors, MR IGRT represents a natural evolution, as MRI fused to the planning CT is already routinely used in the planning process today. Within the current CT-based treatment delivery workflow, normal tissue and tumor-related changes cannot be visualized and, therefore, adaptation is not possible. Changes in the gross tumor volume have been reported when patients are re-simulated during treatment in the setting of glioblastoma.136 The effects of radiation itself have also been shown to induce white matter signal changes even after low doses.137 Therefore, a hybrid MRL will bring the ability to develop personalized adaptive radiation plans throughout the course of therapy for brain tumors, which may translate to improved tumor coverage and sparing of OARs.
Functional imaging sequences such as diffusion, CEST, and dynamic perfusion have the promise to allow for adaptive radiotherapy based on biologic MR-based markers85—for example, dose escalating areas of radioresistance within the tumor volume as these areas become apparent during a course of radiation and those tracts critical to tumor spread, or de-escalation to tracts within the brain showing early demyelination or necrosis. Furthermore, if these markers can determine which tumors will change in volume or biology as opposed to remaining physically and genomically static, this will facilitate patient selection for an MR IGRT system versus a conventional RT device. A hybrid MRL facilitates consistent timing in functional imaging acquisition immediately prior to, during, and after irradiation, which allows for meaningful interpretation and standardization of the sequences and treatment protocol. Optimizing these sequences to allow fast beam-on acquisitions is a challenge and an area of rapid development. Another challenge applicable to the CNS lies in the development of non–contrast enhanced MRI sequences that can visualize the gross tumor volume with the same level of detail as a contrast enhanced image, given that daily contrast is a potential hazard to a patient’s renal function and accumulation in the brain. Furthermore, adaptive RT and online treatment replanning necessitates the development of novel dose calculation algorithms, QA protocols, dosimetry standards in a magnetic field, and treatment planning workflow strategies. Timely generation of new contours for adaptation also requires new automatic tissue segmentation and deformable image registration tools. The theoretical framework has been developed to permit online adaptation on the Elekta MRL,138–140 and the clinical feasibility of online adaptation on the Viewray system has been reported.141
Conclusions
In summary, advancements in MRI and their integration into all phases of RT, from treatment planning to online image guidance to treatment response assessment during and after therapy, are expected to revolutionize CNS radiation oncology. An MRI-only workflow could potentially be both cost- and time-saving while reducing uncertainty associated with CT-MRI registration. Improved identification of critical structures and neural networks via functional imaging will reduce toxicity and preserve neurocognition. The integration of online real-time MR IGRT represents a paradigm shift to current image-guidance solutions for the CNS. Not only does superior soft tissue contrast enable identification of targets and critical structures of interest, but motion management for select tumor sites and functional information via advanced imaging techniques will extend the ability to develop individualized, real-time adaptation strategies.
Funding
Yue Cao’s research is in part supported by NIH grants of RO1 EB016079, 1U01CA183848, P01 CA059827, RO1CA184153; a Siemens Research grant; and an Epicentrix, Inc research grant. James Balter’s research is supported in part by RO1 EB016079 and a Siemens Research grant.
Conflict of interest statement. Dr Arjun Sahgal has received honoraria for past educational seminars from Medtronic, Elekta AB, Accuray Inc, and Varian Medical Systems and research grants from Elekta AB. James Balter and Yue Cao have received research grants from Siemens Healthineers. Yue Cao has received research grants from Siemens Healthineers and Epicentrix, Inc. Yue Cao has received honoraria for educational seminars from Siemens Healthineers.
References
Author notes
Corresponding Author: Yue Cao, PhD, Department of Radiation Oncology, 519 W William Street, Argus Building 1, University of Michigan, Ann Arbor, MI ([email protected]).