-
PDF
- Split View
-
Views
-
Cite
Cite
Katrin Schindler, Tzvetanka Bondeva, Claudia Schindler, Ralf A. Claus, Sybille Franke, Gunter Wolf, Preconditioned suppression of prolyl-hydroxylases attenuates renal injury but increases mortality in septic murine models, Nephrology Dialysis Transplantation, Volume 31, Issue 7, July 2016, Pages 1100–1113, https://doi.org/10.1093/ndt/gfv442
- Share Icon Share
Abstract
Septic conditions contribute to tissue hypoxia, potentially leading to multiple organ failure, including acute kidney injury. The regulation of cellular adaptation to low oxygen levels is regulated by hypoxia-inducible transcription factors (HIFs). While the role of HIFs in ischaemia/reperfusion is more studied, their function in sepsis-induced renal injury is not well characterized. In this study, we investigated whether pharmacological activation of HIFs by suppression of prolyl-hydroxylases (PHDs) protects against septic acute kidney injury.
Two models of sepsis—caecal ligation and punction and peritoneal contamination and infection—were induced on 12-week-old C57BL6/J mice. Pharmacological inhibition of PHDs, leading to HIF activation, was achieved by intraperitoneal application of 3,4-dihydroxybenzoate (3,4-DHB) before sepsis. A quantitative real-time reverse transcription polymerase chain reaction, immunohistology and enzyme-linked immunosorbent assays were utilized to detect gene expression, renal protein levels and renal functional parameters, respectively. Tissue morphology was analysed by periodic acid–Schiff reaction. Early kidney injury was estimated by kidney injury molecule-1 analyses. Apoptosis was detected in situ by terminal deoxynucleotidyl transferase–mediated dUTP nick end labelling stain. The systemic effect of 3,4-DHB pretreatment in sepsis was analysed by 72-h survival studies.
Pharmacological activation of HIFs before sepsis induction attenuated sepsis-related vacuolization and dilation of the proximal tubules, reduced tubular apoptosis and correlated to lower T-cell infiltration in renal tissue compared with the non-treated septic animals. PHD suppression elevated the basal renal HIF-1α expression and basal plasma concentrations of HIF targets erythropoietin and vascular endothelial growth factor. Whereas it preserved renal structure in both models, it improved renal function in a model-dependent manner. Moreover, inhibition of PHDs led to increased mortality in both models. Analysis of liver function showed increased organ destruction with massive glycogen loss and hepatocyte's apoptosis due to 3,4-DHB administration before sepsis induction.
In summary, the pharmacological activation of HIFs by 3,4-DHB administration, although it showed renoprotective effects in sepsis-related kidney injury, induced more severe problems in other organs such as the liver during sepsis, leading to increased mortality.
INTRODUCTION
Septic conditions contribute to tissue hypoxia, [1], apoptosis and multiple organ failure, thereby leading to significant problems in the care of critically ill patients in intensive care units [1–6]. Animal studies have shown that sepsis initiates renal injury [7]. Sepsis is the most common cause of acute kidney injury (AKI) [8, 9]. Patients with a chronic kidney disease are especially susceptible to high mortality when severe sepsis occurs [10, 11]. Septic inflammation is characterized by reduced levels of oxygen in tissue [12], activating transcription factors, termed hypoxia-inducible factors (HIFs), which are the main regulators of cellular accommodation to low oxygen tension [13]. The regulation of HIF-1,2α proteins in normoxia is controlled by prolyl-hydroxylases (PHDs) through an oxygen-dependent hydroxylation of Pro402 and Pro564 residues, followed by ubiquitination and subsequent peroxisomal degradation of the HIF-1,2α molecules [14]. In hypoxia, PHD activity is inhibited, leading to stabilization and accumulation of HIF-1,2α proteins and a complex formation with the HIF-1β subunit, leading to nuclear translocation and transcriptional activation of the HIF target genes [15]. Recently, it has been shown that suppression of PHDs by pharmacological inhibitors such as FG-4497 [16, 17] and 3,4-dihydroxybenzoate (3,4-DHB) [18] leads to HIF-1,2α accumulation in vitro and in vivo [16–18]. Fast up-regulation of HIF-1,2α proteins in renal tissues has been reported in AKI [11] and has also occurred in infections with different human pathogens [19]. There is a growing body of evidence that inhibition of PHDs before AKI may ameliorate renal damage [20]. In addition, the application of HIF targets such as erythropoietin (EPO) before ischaemia-reperfusion protects the kidney from ischaemic insult, as shown in several studies [16, 21, 22]. We also reported that a reduced expression of Morg1 [mitogen-activated protein kinase(MAPK) organizer protein 1], a scaffold module of the PHD3, in Morg1 heterozygous mice showed renoprotective properties in an ischaemia-reperfusion murine model due to increased renal levels of HIF-1,2α proteins [23]. However, data have emerged that the aetiology of non-septic and septic renal dysfunction has distinct mechanisms [24]. Indeed, little is known about whether the systemic activation and stabilization of HIFs is beneficial for sepsis outcome and sepsis-related AKI, and the impact of HIFs in this process has only been rarely investigated. With this study, we explored the role of pharmacological activation of HIF-1,2α in sepsis-induced renal damage by suppression of PHDs activity using 3,4-dihydroxybenzoate in murine sepsis models.
MATERIALS AND METHODS
Animals and experimental design
All animal experiments were approved by the local Animal Committee of the State of Thuringia (file numbers 02-023/10 and 02-023/11). The study was carried out on wild-type male mice (12–16 weeks old) with the C57BL/6J genetic background, purchased from Jackson Laboratory (Bar Harbor, ME, USA). Animals received a standard diet and access to tap water. To assess the role of pharmacological PHD inhibition, mice were i.p. injected with 150 mg/kg body weight ethyl 3,4-DHB (Sigma-Aldrich Chemicals, Munich, Germany), a non-specific PHD inhibitor shown to inhibit all three PHD isoforms [18], 4 h before being subjected to sepsis, or the control treatment. Two polymicrobial sepsis models were chosen: caecal ligation and puncture (CLP) [25, 26] and peritoneal contamination and infection (PCI) [25, 27]. For the CLP operation, the animals were anaesthetized and subjected to midline laparotomy. The caecum was ligated and punctured twice below the constriction with a 21-gauge needle and two small droplets were pressed out of the wound [25, 28]. The corresponding control mice underwent a sham operation that was performed similarly to the CLP except without ligation and puncture of the caecum. The PCI sepsis was initiated by i.p. injection of a human stool suspension with a known bacterial content. In general, 1.5 µL/g body weight was injected from the stool suspension, which contained 3.4 × 1010 bacteria/mL. The corresponding control mice for PCI sepsis were i.p. injected with an equal volume of 0.9% saline. Twenty-four hours after the experimental treatments, the mice were anaesthetized with diethyl ether and sacrificed. The kidneys were extracted and paraffin embedded for further studies. Blood samples were collected for blood plasma isolation. The urine samples were stored at −80°C for further analysis.
Survival analysis
The systemic effects of PHD suppression were assessed by survival rates. The mice were randomly separated into experimental groups of 10 mice each: control, sham-operated; CLP group; 3,4-DHB + CLP group; 0.9% saline-treated, control group; PCI group and 3,4-DHB + PCI group. The animals were monitored every 6 h over a 72-h period and had access to water and standard rodent food.
Analysis of renal function and structure
Plasma levels of creatinine were measured by colorimetric chip assay on a clinical chemical analyser (Fuji DRI-CHEM 3500i, Fujifilm, Düsseldorf, Germany). To calculate the urinary albumin:creatinine ratio (ACR), the creatinine and albumin concentrations in urine were detected by enzyme-linked immunosorbent assay (ELISA) from Cayman Chemical Company (Tallinn, Estland) and CellTrend GmbH (Luckenwalde, Germany), respectively. Organ damage was assessed by periodic acid–Schiff (PAS) reaction performed on 5-µm paraffin kidney sections or 10-µm frozen liver sections by the aid of a PAS staining kit (Baacklab, Schwerin, Germany). The staining was evaluated using an Axioplan microscope and AxioVision release 4.6. software (Zeiss, Jena, Germany). The tubular damage was estimated by a scoring system where 0 represented no damage and 5 corresponded to >90% damaged tubuli.
Immunohistochemistry on paraffin kidney sections
The kidneys were fixed in 10% neutral-buffered formalin and paraffin embedded. The deparaffinized sections were exposed to heat-mediated antigen retrieval in citrate buffer (pH 6.0). After blocking with 5% BSA for 1 h at room temperature, sections were incubated with the primary antibodies. Immunohistochemical detection of the protein expression was performed on 5-µm paraffin kidney sections as already described [29, 30]. The following primary antibodies were used for analysis: goat polyclonal antibody anti-HIF-1α (1:200 dilution) (R&D Systems, Wiesbaden, Germany), goat polyclonal anti-tumour necrosis factor α (TNF-α) (1:200 dilution) (Santa Cruz Biotechnology, Heidelberg, Germany) and anti-CD3 antibody (1 : 100 dilution) (Dianova, Hamburg, Germany). The corresponding secondary antibodies, HRP conjugated (1:500 dilution), were purchased from KPL (Gaithersburg, MD, USA). For HIF-1α and TNF-α, detection was performed using the Vectastain ABC anti-goat IgG detection kit (Vector Laboratories, Burlingame, CA, USA). Peroxidase substrates 3-amino-9-ethylcarbazole (AEC) or diaminobenzidine (DAB) were used as appropriate (Vector Laboratories). When appropriate, the nuclei were haematoxylin counterstained as described elsewhere. The stained sections were analysed using an Axioplan microscope and AxioVision 4.6. software (Zeiss). A minimum of six to eight animals per experimental group were investigated. The staining was analysed by a person unaware of the experimental protocol using the scoring system as previously described [29, 30].
Apoptosis analysis
The apoptosis was investigated by terminal deoxynucleotidyl transferase–mediated dUTP nick end labelling (TUNEL) assay on 4-µm paraffin kidney sections or on 10-µm frozen liver sections by the aid of a commercially available in situ cell death detection kit (Roche Diagnostics, Penzberg, Germany) according to manufacturer's instructions. Sections from at least three animals per treatment were subjected to apoptosis analysis by TUNEL assay. In parallel, positive (DNAse-treated kidney or liver sections) and negative controls were performed (not shown). DAB was used as a peroxidase substrate (Vector Laboratories). The sections were analysed using an Axioplan microscope and AxioVision 4.6 software (Zeiss). To assess the number of positive nuclei, kidney and liver sections were examined under and ×400 magnification and the positive-labelled nuclei were counted and graphically presented as an average of the nuclei detected per field.
Estimation of the tubular damage by detection of the kidney injury molecule-1 (KIM-1)
Tubular damage was analysed on 2-µm paraffin kidney sections via immunodetection of KIM-1 using a rabbit polyclonal antibody to KIM-1 (1:50 dilution) (Uscn Life Sciences, Houston, TX, USA). The immunohistochemistry was performed as described earlier. As peroxidase substrate, we used DAB, and nuclei were counterstained with haematoxylin. Stainings were analysed using an Axioplan microscope and AxioVision 4.6 software (Zeiss). The images were taken with ×200 magnification and were subjected to an evaluation of the tubular damage by measurement of the KIM-1-positive tubular area using ImageJ software. At least 12 images were analysed per treatment. The data are graphically presented as an average KIM-1-positive area per field. Representative images are shown from all animal groups.
Analyses of KIM-1 concentrations in urine with an ELISA
Evaluation of sepsis-related early renal damage was performed as well by detection of the KIM-1 concentrations in urine samples from all animal groups using a KIM-1 ELISA (Uscn Life Sciences). After the corresponding treatments, the animals were housed in metabolism cages and urine was collected for 24 h and used for the detection of ACR and KIM-1 concentrations. The collected urine was stored at −80°C until analysed. The samples were diluted 50 times with sterile PBS and 100 µL was subjected to analyses according to the manufacturer's instructions. Finally, the assays were developed by addition of a 90 µL substrate TMB solution and the absorbance was measured on an Infinate 200PRO microplate reader (Tecan Austria, Groedig, Austria) at 450 nm. The KIM-1 urinary concentrations were calculated and presented in absolute terms (ng/mL). The KIM-1 plasma levels 24 h after experimental treatments from all animal groups were also analysed by KIM-1 ELISA. The KIM-1 plasma concentrations were expressed in absolute terms (ng/mL).
RNA isolation, reverse transcription and real-time PCR
Renal tissue was homogenized using a SpeedMill P12 homogenizer (Analytik Jena Bio Solutions, Jena, Germany) and total RNA was isolated using the RNeasy kit (Qiagen, Hilden, Germany) according to the manufacturer's instructions. The synthesis of cDNA was performed from 1 µg of total RNA using the M-MLV reverse transcription system (Invitrogen Life Technologies, Darmstadt, Germany). The gene-specific primers for the real-time RT–PCR are as follows: tnf-α—forward: 5′ GGCAGGTCTACTTTGGAGTCATTGC 3′, reverse: 5′ ACATTCGAGGCTCCAGTGAATTCGG 3′; gapdh—forward: 5′ TGTCAGCAATGCATCCTGCA 3′, reverse: 5′ GATGTCATCATACTTGGCAGGTT 3′; gusb—forward: 5′ CCGATTATCCAGAGCGAGTATG 3′, reverse: 5′ CTCAGCGGTGACTGGTTCG 3′; hsp90ab1—forward: 5′ AGATTCCACTAACCGACGCC 3′, reverse: 5′ CCGCACTCGCTCCACAAA 3′; tbp—forward: 5′ CAAACCCAGAATTGTTCTCCTT 3′, reverse: 5′ ATGTGGTCTTCCTGAATCCCT 3′; hprt—forward: 5′ ATCAGTCAACGGGGGACATA 3′, reverse: 5′ AGAGGTCCTTTTCACCAGCA 3′. The tnf-α, gapdh and hprt (hypoxanthine phosphoribosyltransferase) primers were purchased from Invitrogen (Darmstadt, Germany), while the gusb (β-glucuronidase), hsp90ab1 [heat shock protein 90-kDa α (cytosolic), class B member 1] and tbp (TATA box binding protein) primers were from TIB MolBiol (Berlin, Germany). The relative expression of the gene of interest was quantified by RT–PCR using a Realplex Mastercycler (Eppendorf, Hamburg, Germany). The quantitative real-time PCR was done as previously described [30]. Analyses were performed on an Eppendorf thermocycler (Eppendorf Instruments, Hamburg, Germany). Expression of the gene of interest was normalized to the expression of five housekeeping genes (gapdh, tbp, hsp90ab1, gusb and hprt) by geometric averaging [31]. The relative expression ratio was quantified by the ΔΔCT method, where R = 2−ΔΔCT [32]. The gene expression of the sham-operated mice with CLP sepsis or the saline-treated animals with PCI sepsis was set as one.
Analysis of liver function
Plasma levels of the liver enzymes alanine aminotransferase (ALT) and aspartate aminotransferase (AST) as markers for liver injury were assessed 24 h after sepsis or endotoxemia induction and were measured by a colorimetric chip assay on a clinical chemical analyser (Fuji DRI-CHEM 3500i, Fujifilm, Düsseldorf, Germany).
Measurements of EPO and vascular endothelial growth factor (VEGF) levels in blood plasma
The plasma levels of EPO and VEGF were analysed by ELISAs from R&D Systems (Minneapolis, MN, USA) and Signosis (Santa Clara, CA, USA), respectively, according to the manufacturer's instructions. The VEGF measurement in our assay did not discriminate between the different VEGF isoforms in blood plasma and in fact represents the total plasma VEGF levels.
Statistical analysis
All values are presented as mean ± SEM. Statistical analyses were performed with SigmaPlot12 software (SYSTAT Software, San Jose, CA USA). Results were evaluated by the Kruskal–Wallis test followed by the Mann–Whitney U-test if applicable. Survival was analysed by the Kaplan–Meier test with log-rank statistic. Differences were considered significant when P < 0.05.
RESULTS
Suppression of PHDs before induction of sepsis ameliorates sepsis-related tubular damage
To evaluate the role of pre-activated HIFs in kidney damage during sepsis, we inhibited the prolyl 4-hydroxylase activity by i.p. application of ethyl 3,4-DHB, a non-specific PHD blocker. The renal injury was assessed by PAS on kidney sections. Our studies revealed that 24 h after the onset of sepsis by CLP or PCI the kidneys showed overt morphological sepsis-induced renal damage, demonstrated by dilatation and vacuolization of the proximal tubule in both sepsis models (Figure 1A, arrows show tubular damage). However, we observed significantly less injury of the proximal tubular cells in mice injected with 3,4-DHB before sepsis induction (Figure 1B). Application of the PHD inhibitor alone in sham-operated or saline-injected controls did not cause tubular damage (Figure 1A, 3,4-DHB panels). Next, we analysed the renal apoptosis in situ by TUNEL stain. The apoptotic cells are represented with TUNEL positively stained nuclei [33]. We found that septic conditions (Figure 2) increased mostly tubular cell apoptosis, whereas glomerular cells were less affected. However, animals that underwent pharmacological HIF activation before sepsis had a decreased overall renal apoptosis, depicted by lower TUNEL-positive staining (Figure 2). Interestingly, 3,4-DHB reagent more effectively suppressed PCI-related tubular apoptosis (Figure 2B) than CLP-dependent apoptosis.
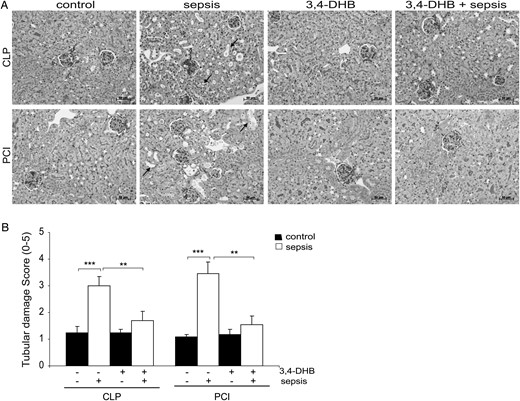
Effect of PHD suppression on renal injury in septic mice assessed by PAS. Inhibition of the PHDs was achieved by i.p. administration of 3,4-DHB 4 h prior to sepsis induction by CLP or PCI. Sepsis-related kidney damage was analysed on 5-µm paraffin kidney sections. (A) Effect of septic conditions and 3,4-DHB application on renal damage. The arrows show the tubular damage presented as vacuolization and dilation of the tubular regions in CLP and PCI septic mice. Less damage is detectable when the PHD activity was suppressed prior to sepsis. Representative images are shown. Scale bars for all images correspond to 50 µm. 3,4-DHB, ethyl-3,4-dihydroxybenzoate; CLP sepsis, caecal ligation and punction-induced sepsis; PCI sepsis, PCI-induced sepsis. (B) Estimation of the tubular damage by scoring system. 0, no damage; 5, extreme vacuolization and dilation of the tubuli. **P < 0.01, ***P < 0.001.
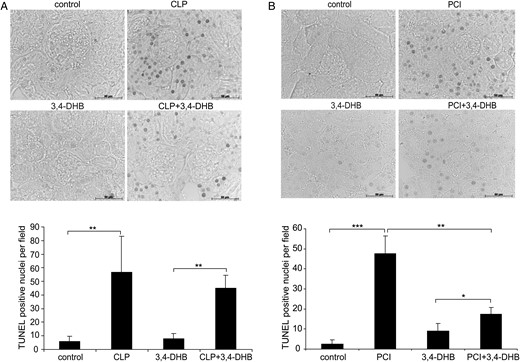
Effect of PHD inhibition on sepsis and endotoxemia-induced renal apoptosis. Apoptotic events were analysed on paraffin kidney sections by TUNEL assay. The staining was analysed using an Axioplan microscope and Axiovision 4.6 software (Zeiss). Few apoptotic nuclei were detected at baseline. Mostly tubular cells underwent apoptosis under our experimental conditions. Images were taken under ×400 magnification. The number of TUNEL-positive nuclei was counted and is graphically presented for each treatment. (A) CLP induced apoptosis in kidney tissue. Septic conditions significantly increased the number of apoptotic nuclei (**P < 0.01 versus sham-operated mice), which was lower in mice that received 3,4-DHB before CLP induction. Representative images are shown. Scale bars correspond to 50 µm. n = 3 per treatment. (B) PCI-dependent apoptosis in renal tissues. Inhibition of PHDs prior to PCI sepsis significantly attenuated renal apoptosis compared with PCI treatment alone (**P < 0.01 versus PCI) (***P < 0.001, **P < 0.01, *P < 0.05). Representative images are shown. Scale bars correspond to 50 µm. n = 3 per treatment.
Inhibition of PHDs prior to sepsis attenuates microalbuminuria development in PCI-treated mice
To better understand the effects of pharmacological PHD blockers in sepsis on renal function under our experimental settings, we measured two functional parameters: urinary ACR [34] and plasma creatinine [35]. We found that 24 h after the onset of sepsis the mice developed moderate albuminuria, defined as ACR ≥ 30 mg/g [34] in both models (Figure 3A). This was not observed in the saline-treated controls or sham-operated animals. The line on the graph (Figure 3A) corresponds to ACR + 30 mg/g; higher values are an indication for the presence of microalbuminuria. However, while HIF preactivation was ineffective in preventing CLP-related microalbuminuria, most of the animals injected with 3,4-DHB did not develop albuminuria, as shown by ACR < 30 mg/g (Figure 3A). Furthermore, the plasma creatinine concentrations were significantly raised 24 h after CLP surgery compared with the sham-operated mice, and the pharmacological HIF activation before CLP sepsis did not reduce plasma creatinine concentrations (Figure 3B). Interestingly, PCI treatment did not affect the plasma creatinine level during the first 24-h period of sepsis initiation (Figure 3B).

Evaluation of renal damage by analyses of functional parameters. The plasma creatinine concentrations and the urinary ACR were analysed. (A) Determination of ACR. The urinary albumin and creatinine concentrations were measured in 24-h urines. The dotted line corresponds to ACR + 30 mg/g; higher values are known as microalbuminuria. Septic conditions clearly lead microalbuminuria (ACR ≥ 30 mg/g). 3,4-DHB treatment reduced microalbuminuria in PCI, but not in the CLP model. n = 9–12 per treatment. (B) Plasma creatinine (CRE) levels. Plasma concentrations of creatinine significantly increased only in the CLP model (**P < 0.01 versus sham-operated controls). 3,4-DHB treatment failed to normalize the increased plasma creatinine (*P < 0.05 versus saline control). n = 9–12 per treatment.
Effect of PHD inhibition on the levels of the early renal injury marker KIM-1
We evaluated whether pre-septic suppression of PHDs affects the renal levels of KIM-1, a new marker for early detection of acute renal damage in animal models [36] and in humans [37]. Immunohistology of renal sections showed that KIM-1 was almost undetectable in the sham-operated as well as in saline-treated mice, and exposure to 3,4-DHB prior to sepsis did not have a significant impact on its levels in renal tissue (Figure 4A). On the other hand, 24 h after CLP and PCI sepsis, its tubular levels were greatly increased (Figure 4A and B) and 3,4-DHB treatment slightly but not significantly reduced its expression in renal tissue compared with sepsis alone. Interestingly, KIM-1 protein was elevated not only in the damaged tubuli, but also the apical membranes of the epithelial cells in completely intact tubules were KIM-1 positive (arrows show KIM-1 expression on the surface of the cells, Figure 4A). Under ischaemic conditions, the ectodomain of KIM-1 is shed into the urine; thus, we also investigated whether PHD inhibition affects the urinary levels of KIM-1. Although in healthy animals its amounts are normally lower than 1 ng/mL [36], we found accumulated KIM-1 basal levels in 24-h-collected urine samples in sham-operated and saline-injected controls, probably due to the collection time when the animal remained in the metabolic cages (Figure 4C). PHD blockade to some extend further elevated basal urinary KIM-1 concentrations. CLP and PCI septic mice showed significantly augmented KIM-1 urinary concentrations compared with the sham-operated and saline-exposed mice. Furthermore, pretreatment with 3,4-DHB followed by sepsis induction did not significantly impact KIM-1 urinary levels relative to 3,4-DHB-treated controls, presumably due to the elevated basal levels of KIM-1 protein in urine probes from PHD inhibitor–treated controls (Figure 4C). We assayed as well the KIM-1 plasma concentrations in all animal groups. Although CLP septic mice revealed significantly higher KIM-1 plasma levels relative to sham-operated mice, the pharmacological HIF preactivation followed by CLP did not significantly increase KIM-1 concentrations above those of the corresponding 3,4-DHB-injected sham-operated mice (Figure 4D). On the other hand, PCI treatment was not characterized by elevated KIM-1 protein amounts in blood plasma, neither at the absence nor in the presence of PHD inhibitors (Figure 4D).
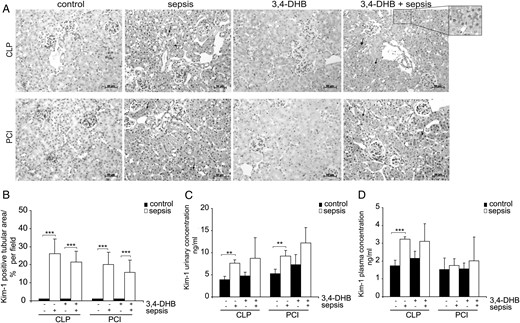
Detection of KIM-1 expression in renal tissue, urine and blood plasma. (A) Evaluation of tubular injury by immunohistological analyses of KIM-1 in kidney paraffin sections. The nuclei were counterstained with haematoxylin. The images were taken with ×200 magnification. Sham-operated or saline-treated animals showed almost undetectable KIM-1 tubular staining. PHD suppression did not influence significantly the basal or sepsis-dependent KIM-1 expression in renal tubuli. The arrows show the expression of KIM-1 in the surface of tubular epithelial cells. An enlargement of the KIM-1 tubular stain is shown in box. The nuclear KIM-1-positive stain in some of the images should be considered as non-specific staining. Representative images are shown. Scale bars correspond to 50 µm. n = 3 kidney sections per treatment. (B) Evaluation of the KIM-1-positive tubular area per field. The KIM-1-positive tubular area was analysed by ImageJ software and presented as the averaged per cent per field. At least 12 images per treatment were evaluated. ***P < 0.001. (C) Measurement of KIM-1 protein concentrations in 24-h collected urines by ELISA. The concentrations are calculated and expressed in absolute terms (ng/mL). Septic conditions increased the urinary KIM-1 levels and HIF presepsis activation slightly elevated basal as well as sepsis-dependent KIM-1 urinary concentrations. **P < 0.01, n = 4 per experimental settings. (D) Detection of KIM-1 plasma concentrations by ELISA. CLP sepsis induced KIM-1 plasma expression, but PHD inhibition did not impact KIM-1 plasma levels. PCI sepsis did not affect KIM-1 plasma levels compared with the corresponding sham-operated or saline-treated mice. ***P < 0.001, n = 4 per treatment.
3,4-DHB effects on renal expression of TNF-α after induction of sepsis
To investigate the development of inflammation in renal tissue by sepsis and the influence of preconditional HIF activation, we analysed expression of the pro-inflammatory cytokine TNF-α on protein and mRNA levels 24 h after treatment. Immunohistology showed a significant inducement of TNF-α protein expression in the kidney sections of both sepsis models compared with the sham-operated and saline-injected animals (Figure 5A and B), and 3,4-DHB administration before the onset of sepsis significantly reduced TNF-α renal levels relative to CLP alone (Figure 5A and B). TNF-α renal protein levels were also diminished by HIF preconditioning in the PCI sepsis model, but the differences did not reach statistical significance (Figure 5A and B). We also assayed renal expression of Tnf-α in whole kidney mRNA. We found that 3,4-DHB application inhibited significantly the basal level of Tnf-α mRNA compared with the sham-operated non-treated mice (Figure 5C), an effect that was not measured in saline-treated controls. Furthermore, we found an elevated but not significantly increased Tnf-α expression in CLP sepsis compared with sham-operated mice, and HIF pharmacological preactivation showed a mild reduction of Tnf-α levels. On the other hand, PCI septic conditions significantly augmented Tnf-α expression relative to controls in the presence and absence of 3,4-DHB blocker (Figure 5A and C).
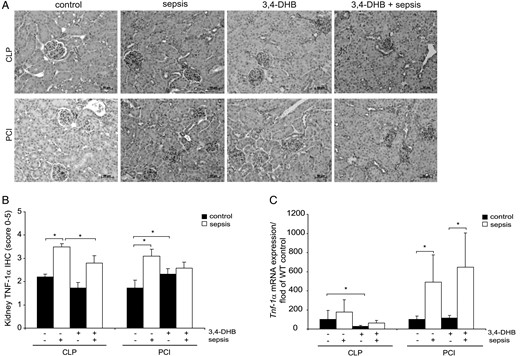
TNF-α protein and gene expression in renal tissue. (A) Immunohistological detection of renal TNF-α protein expression. The nuclei were counterstained with haematoxylin. Representative images are shown. Scale bars correspond to 50 µm. n = 9–12 kidney sections per treatment. (B) Estimation of TNF-α protein expression in kidney by scoring. The graph represents the evaluations of each treatment (CLP or PCI) separately without a comparison between the groups. *P < 0.05, n = 9–12. (C) Relative expression of Tnf-α mRNA studied by quantitative real-time PCR. The data are presented in fold relative to the corresponding wild-type saline control or sham-operated control mice. 3,4-DHB inhibited basal Tnf-α renal expression in the CLP septic group and also decreased, but not significantly, sepsis-dependent Tnf-α expression. In PCI septic animals, 3,4-DHB leads to a significant increase of Tnf-α mRNA expression post-sepsis. The quantifications shown in the graph are presented for each treatment (CLP or PCI) separately without a comparison between the groups. *P < 0.05, n = 9–12 per treatment.
Inhibition of PHDs reduced sepsis-related renal infiltration by T cells
AKI is characterized by a rapid infiltration of T cells into the kidney [38]. Immunohistochemistry was used to evaluate the renal CD3+ T-cell infiltration in sepsis and the influence of HIF preactivation. As expected, both sepsis models were characterized by a significant increase in renal infiltration with CD3+ T cells compared with controls (Figure 6). However, application of 3,4-DHB significantly reduced T-cell infiltration into renal tissue in the CLP but not the PCI sepsis model (Figure 6B).
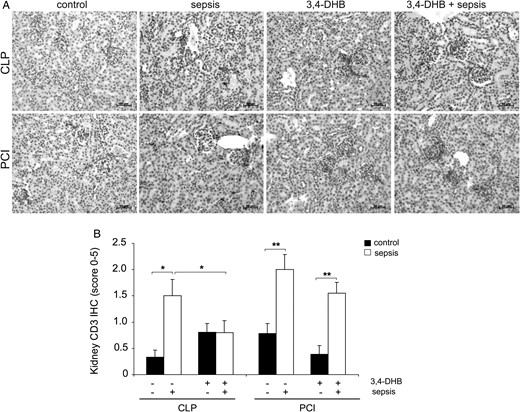
Determination of renal T-cell infiltration. Presence of T cells in the renal tissue was evaluated by CD3 immunohistochemistry. (A) Immunohistological detection of renal CD3-positive T cells. The nuclei were counterstained with haematoxylin. Representative images are shown. Scale bars correspond to 50 µm. n = 9–12 kidney sections per treatment. (B) Quantification of T-cell infiltration in controls and septic mice in the presence or absence of 3,4-DHB by the scoring system. The graph represents the quantification of each treatment (CLP or PCI) separately without a comparison between the groups. T-cell infiltration was elevated in both sepsis models (*P < 0.05 CLP versus sham-operated mice; **P < 0.01 PCI versus saline control), but while 3,4-DHB reduced the number of renal T cells in the CLP model (*P < 0.05 versus CLP alone), this effect was not seen in PCI-treated mice.
Inhibition of PHDs prior to sepsis influences plasma concentrations of EPO and VEGF
Stabilization and activation of HIF-1,2α is associated with the activation of several HIF target genes, including EPO [13, 39, 40] and VEGF [13, 40, 41]. We found that activation of HIFs in healthy animals by PHD inhibition increased the basal levels of EPO and VEGF proteins in the blood plasma relative to the untreated controls (Figure 7). We also detected slight numerical increases of EPO and VEGF plasma concentrations due to septic conditions, but the differences were not statistically significant. Moreover, 3,4-DHB prior to sepsis induction did not affect EPO plasma levels (Figure 7A) but decreased sepsis-dependent VEGF augmentation (Figure 7B).

Effect of HIF preconditioning on EPO and VEGF protein plasma levels after induction of sepsis. Concentrations of EPO and VEGF were measured by ELISA from blood plasma isolated 24 h after the corresponding treatment of the animals, as shown on the graphs. The VEGF ELISA detected all VEGF isoforms in blood plasma. (A) EPO plasma levels, n = 6–12. (B) VEGF plasma levels, n = 6–12.
Effect of 3,4-DHB on HIF-1α expressionin renal tissue
It is well known that the protein levels of HIFs are increased under hypoxic conditions due to suppression of oxygen-sensitive PHD activity [14]. Thus, we evaluated the influence of sepsis and pharmacological suppression of PHDs on HIF-1α renal expression by immunohistochemistry. Upon activation, HIF-1α binds to the HIF-1βisoform and translocates to the nucleus where it induces transcription of the HIF target genes [17, 40, 42]. We observed that septic conditions significantly induced the stabilization and activation of HIF-1α in renal tissue, as HIF-1α protein was mostly detected in the nuclei of the cells (Figure 8A, arrows point the nuclear staining of HIF-1α). In addition, we detected in sham-operated controls increased staining of HIF-1α compared with that of saline-treated mice, which was probably related to the operative intervention, demonstrating that in vivo HIF-1α protein levels could also be elevated to some extent from local inflammatory signals without development of hypoxia or septic conditions. As expected, due to the pharmacological inhibition of HIF-1α protein degradation, the basal HIF-1α expression was significantly raised by 3,4-DHB application relative to that of saline-treated controls (Figure 8B). Interestingly, the pre-septic application of PHD inhibitor did not significantly elevate HIF-1α protein expression above the septic CLP or PCI conditions.
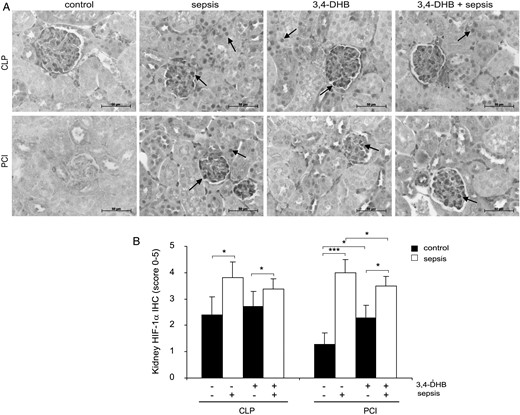
Influence of PHD inhibition on HIF-1α protein expression in murine kidney after sepsis induction. Inhibition of the PHDs was achieved by i.p. administration of 3,4-DHB 4 h prior to sepsis induction. (A) Immunodetection of HIF-1α protein in 4-µm paraffin kidney sections. The staining was analysed using an Axioplan light microscope and Axiovision 4.6 software. Representative images are shown. Scale bars correspond to 50 µm. n = 9–12 kidney sections per treatment. (B) Evaluation of the HIF-1α protein immunostaining in kidney sections by the scoring system. The graph represents the evaluations in every treatment (CLP or PCI) separately without a comparison between the groups. Sepsis induction (*P < 0.05 CLP versus sham-operated mice; ***P < 0.001 PCI versus control) significantly elevated HIF-1α renal expression. The administration of 3,4-DHB in healthy animals increased the basal HIF-1α renal protein (*P < 0.05 versus saline-treated control). CLP and PCI sepsis induced higher HIF-1α protein levels in the kidney and preactivation of HIFs by 3,4-DHB reduced the HIF-1α protein accumulation (*P < 0.05 versus PCI alone).
Influence of PHD suppression on animal survival in sepsis
The systemic effect of PHD suppression prior to sepsis induction by CLP or PCI was further investigated by studying the survival rate of the mice. The animals were monitored over a 72-h period. We found that in contrast to the preservation of the renal structure, reduced apoptosis in the PCI septic model and the anti-inflammatory properties of HIF pharmacological activation prior to sepsis initiation, the survival rate in CLP and PCI septic mice that underwent 3,4-DHB administration was reduced relative to the septic mice that did not received 3,4-DHB treatment (Figure 9).
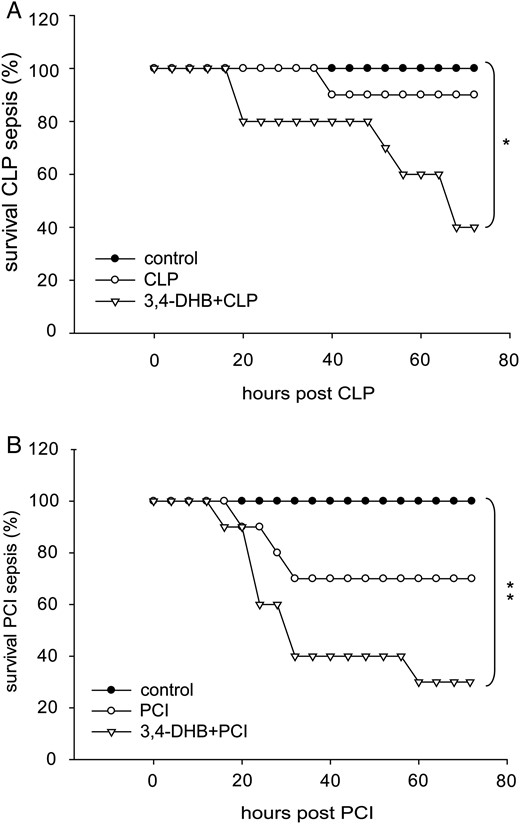
Effect of 3,4-DHB pretreatment on survival outcome in mice after polymicrobial sepsis induction. Survival was monitored every 6 h over a 72-h period. The mice were separated randomly into control groups representing the sham-operated mice or saline-injected control mice. CLP group, mice subjected to CLP sepsis; PCI, mice that underwent PCI sepsis; 3,4-DHB + CLP or 3,4-DHB + PCI, mice pretreated with 3,4-DHB inhibitor for 4 h, followed by CLP or PCI sepsis induction. Significance was calculated by Kaplan–Meier log-rank test. (A) Survival outcome in CLP-treated mice. PHD inhibition reduced survival (*P < 0.05 versus sham-operated mice). n = 10 per treatment. (B) Survival outcome in PCI-treated mice. Similar to CLP septic mice, preactivation of HIFs prior to sepsis was associated with worsening survival ability of the animals (*P < 0.05 versus saline control). n = 10 per treatment.
Effect of HIF preconditioning on the liver
To further address a potential cause for the increased mortality in septic animals pretreated with 3,4-DHB during survival studies, we investigated liver function, morphology and apoptosis. We analysed the plasma levels of ALT and AST as markers for liver injury [43]. We found that ALT levels were elevated 24 h after CLP and PCI sepsis induction and a pharmacological preactivation of HIFs further worsened liver function, as we found significantly increased plasma ALT activity compared with controls (Figure 10A). In the CLP sepsis model, 3,4-DHB administration significantly enhanced the ALT level above CLP alone (Figure 10A). The AST levels were also raised in CLP septic mice, while PCI sepsis had a lesser effect on AST activity (Figure 10B). Using the PAS reaction on frozen liver sections 72 h after sepsis induction, we detected that CLP sepsis was associated with a strong reduction of PAS-positive hepatocytes, showing increased liver destruction and glycogen loss compared with the sham-operated mice, and HIF preactivation completely abolished the PAS reactivity. A similar effect was observed in PCI septic mice, but to a lesser extent. Furthermore, we analysed liver apoptosis 72 h after treatments using the TUNEL assay and found a significant increase on the apoptotic cells in all experimental models and suppression of PHDs prior to CLP or PCI sepsis initiation did not protect the liver tissue from sepsis-induced apoptosis (Figure 10C). 3,4-DHB, treatment of control mice without sepsis exhibited no significant effects on liver parameters compared with control mice without 3,4 DHB indicating that the substance alone was not liver toxic (Figure 10).
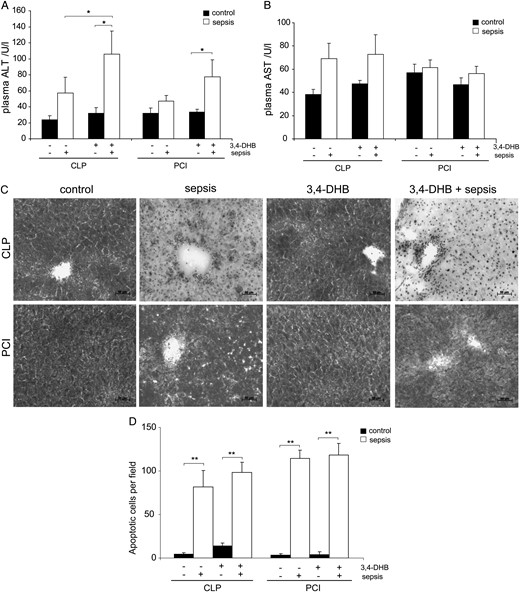
Analysis of liver function, structure and apoptosis. (A) Detection of plasma ALT 24 h post-sepsis as a marker for liver function. n = 9. 3,4-DHB significantly increased ALT concentrations in animals with CLP sepsis compared with mice receiving no 3,4-DHB (*P < 0.05 versus CLP alone). 3,4-DHB exhibited no significant effect on control mice without CLP. 3,4-DHB application increased ALT in septic mice of PCI model (*P < 0.05 versus control). (B) Detection of AST in blood plasma 24 h post- treatment. n = 9. A numerically, but not statically significant increase in AST was found in both models in septic animals receiving 3,4-DHB compared with septic animals without treatment. 3,4-DHB did not increase AST in normal control mice. (C) Analysis of HIF preconditioning in sepsis on liver morphology and the accumulation of glycogen in hepatocytes by PAS reaction on 10-µm liver sections 72 h post-treatment. 3,4-DHB application prior to sepsis further elevated the sepsis-dependent liver destruction as detected by severe reduction of glycogen accumulation in the liver. However, 3,4-DHB had no obvious toxic effects in healthy control mice without sepsis. Representative images are shown. Magnification ×200. Bars 50 µm. Liver sections from at least three animals per treatment were analysed by PAS. (D) Assessment of liver apoptosis by TUNEL assay on liver sections 72 h post- treatment. TUNEL-positive cells were analysed using an Axioplan light microscope and Axiovision 4.6 software. The images were taken under ×400 magnification; the apoptotic cells were counted and are graphically presented (**P < 0.01). Liver sections from at least three animals per treatment were analysed. 3,4-DHB treatment further numerically increased sepsis-associated apoptosis in the liver, whereas the PHD inhibitor had no significant effect on non-septic control mice.
DISCUSSION
Septic conditions are well known causes of AKI [8] and contribute to tissue hypoxia [1, 44]. The stabilization of HIFs in renal tissue has been found under septic conditions as well as in AKI [6, 45, 46]. HIFs are the principal regulators of cellular adaptation to hypoxia through transcriptional enhancement of gene expression in the hypoxic state [40]. The stabilization of HIF proteins in hypoxia is caused by suppression of PHD enzymatic activity, the major oxygen sensors in the cell [14, 47]. It has been demonstrated that the pharmacological induction of HIFs by application of PHD inhibitors is renoprotective in several kidney injuries [48–51]. Consequently, preconditioned HIF activation has attracted much attention as a promising therapeutic target in AKI treatment. Extensive research has shown that PHD inhibitors—L-Mimosin [52], ICA [21], FG-4487 [16] or FG-4497 [20]—efficiently protect renal tissue from damage under ischaemic conditions. However, the question of whether HIF activation by suppression of PHDs is beneficial in sepsis-related kidney injury is less clear and studies are rare. In this investigation, we report that preconditioned activation of HIFs by administration of 3,4-DHB (a non-specific PHD inhibitor) before the onset of sepsis protects the kidney against sepsis-related renal injury. We found that CLP and PCI sepsis led to overt histological damage characterized by dilation and vacuolization and a reduction of the brush border of proximal tubular epithelial cells in septic mice. Intriguingly, the suppression of PHDs attenuated the renal injury in both bacterial sepsis models. In addition, we showed that preactivation of HIFs preserved kidney morphology and significantly decreased renal apoptosis in the PCI sepsis model, but not in the CLP model. At present, there is a need for specific markers to detect early kidney injury under septic conditions [53]. Levels of plasma creatinine [35, 54] and urinary ACR have been used as parameters of renal function. Our data demonstrate that the above functional parameters were differentially affected depending on the model type. Urine ACR and plasma creatinine were induced by CLP sepsis, and the preconditional HIF activation did not improve either parameter, thus showing no beneficial effect for renal function in this sepsis model. On the other hand, PCI septic conditions also increased the ACR, but HIF preactivation attenuated microalbuminuria and improved renal function. In addition, we found that PCI septic animals did not show increased plasma creatinine levels 24 h after the onset of sepsis, which could be increased later during PCI sepsis. Therefore, it is obvious that improvement of the renal structure and reduced inflammation, presumably due to the detected lower renal levels of TNF-1α in CLP septic mice that underwent pharmacological HIF activation, did not correlate with improvement of renal function in this murine model. Furthermore, PHD suppression before sepsis did not affect plasma creatinine levels, while it could normalize the ACR. These findings demonstrate that the evaluation of renal function during septic conditions should include more than one parameter, as our data show that the measurement of only plasma creatinine at early time points probably did not show the early renal injury before reduced GFR is observed. In agreement with this, we found that the levels of KIM-1, a marker for early detection of kidney tubular injury [36, 37], were increased in renal tubuli in both sepsis models. Moreover, even tubuli, which under PAS analyses are evaluated as intact and undamaged, have shown positive KIM-1 immunoreactivity, thus suggesting early tubular damage that cannot be evaluated by PAS reaction or plasma creatinine measurements. On the other hand, although some studies have shown that pharmacological pre-ischaemic PHD suppression reduced AKI-associated fibrosis in murine models [55], we detected a small decrease in KIM-1 tubular expression in preseptic activation of HIFs compared with the sepsis treatment alone. The inhibition of PHDs prior to sepsis also did not attenuate KIM-1 urinary levels; in contrast, we detected an elevation of the KIM-1 protein in 24-h-collected urine samples. Of course, this effect could be related to accumulation of the KIM-1 protein in urine during the 24-h post-sepsis period, but the plasma KIM-1 concentrations were also unaffected by preseptic inhibition of PHDs in the CLP sepsis model. Interestingly, we found that PCI septic conditions did not impact the KIM-1 plasma levels and are independent from HIF preactivation during sepsis, an effect that we observed for plasma creatinine. KIM-1 expression was found to be sensitive to ischaemia, thus hypoxia preconditioning could be to some extent associated with an increase in renal KIM-1 levels, which needs to be further investigated in more detail. In other studies, it has been reported that the application of ICA, a different PHD inhibitor used in a rat model of ischaemia-reperfusion, reduced creatinine plasma concentrations [21]. The different effects of PHD blockers could be inhibitor specific as well as dose dependent or due to differences between septic and non-septic AKI mechanisms. Recent studies, not surprisingly, have reported that creatinine values in septic mice do not correlate with the severity of the kidney injury [54]. We also did not find significant differences in the plasma creatinine or KIM-1 levels of PCI septic mice, although severe renal histological damage was detected. Our data also show that the induction of sepsis correlates with an increased accumulation of HIF-1α protein in the tubular and glomerular regions of the kidney. The application of 3,4-DHB augmented significantly HIF-1α basal protein expression compared with the corresponding controls and showed a different impact on HIF-1α protein levels when injected before the onset of sepsis. Furthermore, basal plasma concentrations of the HIF targets EPO or VEGF were increased 2-fold, which demonstrates that the inhibitor was effectively activating HIFs on a systemic level. Previous reports have found that the administration of EPO after endotoxemia or CLP sepsis improved animal survival [22], but the elevation by preconditioned HIF by administrating 3,4-DHB was not correlated with a further augmentation of the EPO plasma levels compared with the CLP or PCI septic animals without 3,4-DHB. Our findings agree with previous studies using 3,4-DHB in a rat model, as the authors also did not observe a significant accumulation of HIFs in renal tissue [18] after 3,4-DHB administration. However, these authors detected stimulation of angiogenesis due to elevation of the HIF target genes [18]. We also measured the plasma concentrations of the cytokines IL-6 and INF-γ in our experimental settings, but we did not observe a beneficial effect of HIF pharmacological activation before sepsis on these parameters (data not shown).
We utilized two polymicrobial sepsis models and clearly observed local renoprotective effects and reduced inflammatory markers, as well as diminished levels of TNF-α in renal tissue and reduced T-cell infiltration after 3,4-DHB pretreatment. Yet, overall survival did not seem to benefit; in fact, treatment with 3,4-DHB correlated with increased animal mortality. It seems clear that the initial EPO or VEGF increase before the onset of sepsis was not protective on a systemic level. Furthermore, our data also agree with early reports demonstrating that anti-TNF-α therapy increased survival in the lipopolysaccharide (LPS) model, but not in CLP-induced sepsis [28, 56]. Therefore, it should be noted that data on the protective or beneficial effects observed only in endotoxemia models should be considered with great caution. In this regard, anti-TNF-α therapy did not provide patients any clinical improvement [57]. Our findings are supported in part by the study from Kiss et al. [58], demonstrating that genetic manipulation of PHD3 in PHD3−/− animals is associated with decreased survival in different abdominal sepsis models due to early organ dysfunction [58]. Using 3,4-DHB, we suppressed the activity of all PHD isoforms. Thus, we cannot answer whether there is an isoform-specific effect on the sepsis signalling cascades. Moreover, we assumed that the administration of 3,4-DHB induced the stabilization of all HIF-α subunits, thus raising the possibility of HIF subunit-specific roles in sepsis pathophysiology. This hypothesis has also been supported by the findings that targeted removal of HIF-1α in T cells improved septic mice survival in different models of abdominal sepsis [59], as well as in studies by Peysonnaux et al. [60] revealing that conditional gene targeting of HIF1-α in macrophages is associated with a reduced mortality in LPS murine models [60]. Despite the local renoprotective effects of 3,4-DHB, we detected a worsening in liver function and elevated liver apoptosis, which could be responsible in part for the higher mortality in septic mice that underwent preconditional HIF activation compared with septic conditions alone. In addition, injury to other organs such as the lung and heart could contribute to the negative systemic outcome of 3,4-DHB pretreatment. A limitation of our study is that 3,4-DHB may have other effects besides inhibition of various PHDs [18]. However, 3,4-DHB had no apparent liver toxic effects in healthy control mice without sepsis. These findings strongly indicate that PHD inhibition in the liver during sepsis may lead to liver failure (and likely enhance liver inflammation/apoptosis) and an increase in mortality. Clearly, further studies beyond the scope of this initial work are necessary to evaluate the pathophysiological mechanisms of how PHD inhibition leads to liver failure in sepsis. In summary, the application of 3,4-DHB for pharmacological PHD suppression apparently induces more severe injury in other organs during sepsis, despite the local renoprotective effects.
In conclusion, the pharmacological activation of HIFs by 3,4-DHB administration, although it showed some renoprotective effects in sepsis-related kidney injury, induced more severe problems in other organs such as the liver during sepsis, leading to increased mortality.
AUTHORS’ CONTRIBUTION
K.S., T.B. and G.W. designed the study. K.S., T.B., C.S., R.A.C. and S.F. performed the experiments. K.S., T.B. and G.W. drafted the manuscript. G.W. conceived the study. All authors read and approved the final manuscript.
FUNDING
This study was supported by grants from the German Federal Ministry of Education and Research within the Center for Sepsis Control and Care (grant 01EO1002, project D1.3 to G.W.).
CONFLICT OF INTEREST STATEMENT
None declared.
REFERENCES
Author notes
These authors contributed equally to this study.
Comments