-
PDF
- Split View
-
Views
-
Cite
Cite
Madeline E Clark, Andrew Farinha, Alan R Morrison, George P Lisi, Structural, biological, and biomedical implications of mRNA interactions with the master regulator HuR, NAR Molecular Medicine, Volume 2, Issue 1, January 2025, ugaf002, https://doi.org/10.1093/narmme/ugaf002
- Share Icon Share
Abstract
Human antigen R (HuR) is a ubiquitously expressed RNA-binding protein (RBP) that has been implicated in a vast range of biological processes including stress response, angiogenesis, cell proliferation, and differentiation. Dysregulation of HuR has been linked to a number of pathological disorders including vascular disease, inflammation, and cancers such as those of the breast and colon. Like many RBPs, HuR is composed of multiple RNA-recognition motif (RRM) domains; however, HuR and the three other members of the Hu family (HuB, HuC, and HuD) possess a unique structural composition with two RRMs separated from a third C-terminal RRM by a long, unstructured hinge region. While there has been extensive research on the role of HuR in cellular, molecular, and developmental biology, there are fewer structural and biochemical studies of HuR and many questions still remain about the molecular mechanisms of HuR. In this review, we endeavor to synthesize existing HuR research spanning the last three decades in order to define known mechanistic roles of each domain, highlight remaining uncertainties, and provide a backdrop for ongoing research into the chemistry and biology of HuR and similar multi-RRM containing proteins.
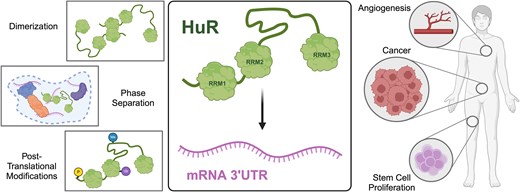
Introduction
Tight control of messenger RNA (mRNA) stability and translation is critical to maintaining cell homeostasis and regulating biochemical pathways in eukaryotes. RNA-binding proteins (RBPs) are an important facet of post-transcriptional regulation throughout the genome, and the study of RBP–RNA interactions is foundational to our understanding of healthy and disease-state gene expression. Human antigen R (HuR) is a ubiquitously expressed RBP that has been implicated in various cellular processes including stress response [1], angiogenesis [2], cell proliferation [3], differentiation [4], and tumorigenesis [5]. HuR binds and regulates a wide variety of mRNA targets via adenylate-uridylate-rich elements (AU-rich elements, AREs) in 3′ untranslated regions. AREs are well-studied cis elements that defer a short cellular half-life to mRNAs and bias them toward degradation. AREs also serve as substrates for a class of RBPs known as ARE-binding proteins (AUBPs). Despite HuR being one of the most well-characterized AUBPs, many questions regarding the molecular details of its RNA-regulating mechanism remain. Thus, a more complete understanding of the structure–function interaction paradigm of HuR is critical to continued efforts to target HuR as a therapeutic for cancer, cardiac health, and other diseases [5–7].
HuR is one of four mammalian members of the Hu family, which also includes HuB, HuC, and HuD. HuR is the only ubiquitously expressed Hu protein, while the rest are primarily neuronal [8] with some studies reporting expression in areas such as lens fiber cells [9] and spermatogonial stem cells [10]. The Hu family of proteins is also referred to as Elav-like (ELAVL) proteins due to their similarity to a family of RBPs first described in Drosophila [11]. Mutations at this genetic locus in Drosophila result in embryonic lethality and/or issues in eye development, hence the name embryonic lethal, abnormal vision system (elav) [12]. Many biological functions have been ascribed to the Hu proteins, including neuronal development, neuronal plasticity and memory [11], and telomerase activity [11] for HuB, HuC, and HuD. Existing evidence suggests that all Hu proteins function by binding and regulating mRNA targets [13, 14].
Every Hu protein contains three canonical RNA recognition motif (RRM) domains, with the first two RRMs existing in tandem and the final RRM being separated by a long, unstructured, basic hinge region (Fig. 1A). While crystal structures have been solved of all three RRM domains [15, 16] of HuR and two of the RRMs in HuD [17], no full-length structure has been solved for any of the Hu proteins, likely due to the unstructured and dynamic nature of this hinge region. AlphaFold-predicted structures of all four Hu proteins include the RRM domains with high accuracy and confidence [likely due to their presence in the Protein Data Bank (PDB)]; the remaining linker regions are unstructured and predicted with low confidence [18]. All three RRMs are highly conserved across the Hu family, especially at the RNA-binding sites. The hinge region has some sequence similarity but varies in length dramatically across the Hu family, ranging from 57 residues in HuR to 84 in HuD. While all Hu hinge regions are predicted to be unstructured and very basic (isoelectric points ranging from 10.5 to 11.6), they have diverse amino acid compositions and are therefore not low-complexity or prion-like. Conversely, the N-terminal unstructured region preceding RRM1 in all four Hu proteins displays almost no sequence conservation across the family.
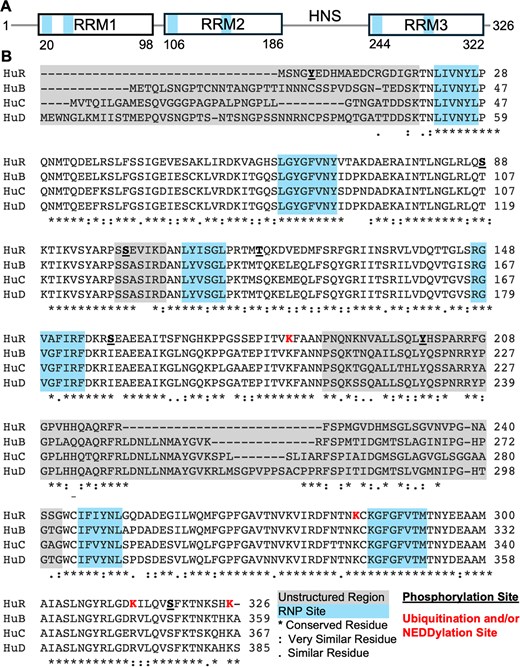
Domain organization of HuR. (A) Domain boundaries of HuR, with connecting lines representing unstructured/linker regions. (B) Sequence alignment of HuR, HuB, HuC, and HuD conducted using Clustal Omega (https://www.ebi.ac.uk/jdispatcher/msa/clustalo). Locations of unstructured linker regions between RRM domains and canonical RNP sites are noted in blue and gray, respectively.
HuR: a tripartite RRM-containing AUBP
The RRM is a domain structure which is present in over 50% of RBPs in the human proteome [19]. The canonical RRM is ∼90 amino acid residues in length and composed of four β-strands packed against two α-helices in a highly conserved β1α1β2β3α2β4 topology. The major RNA-binding sites of canonical RRMs are denoted RNP1 (located on the β3 strand) and RNP2 (β1 strand), and each RRM typically binds 2–5 nucleotides [20]. The three RRMs of HuR are nearly structurally identical despite significant sequence divergence (Fig. 2A and B). Each of the RRMs diverge from the canonical ribonucleoprotein (RNP) sequences in unique ways, primarily in the composition of aromatic residues in RNP1 and RNP2; e.g. RNP2 of RRM1 is missing a canonical aromatic residue while RNP2 of RRM3 contains an additional aromatic residue compared to the canonical sequence (Fig. 2A and C). These differences likely allow for the differential binding affinities for various RNA substrates reported for each individual RRM [15, 16]. Immunoprecipitation of HuR–RNA complexes from human RKO cells followed by a complementary DNA (cDNA) array hybridization screen identified a 17- to 20-nucleotide long U-rich consensus motif that was found in nearly all mRNA targets known at the time [21]. An “all-or-nothing” method of HuR target recognition was proposed wherein two requirements must be met: (i) the target must contain a minimum of nine nucleotides with a consensus motif of N-N-U-U-N-N-U-U-U, and (ii) this region must be in a single-stranded conformation in order for HuR to access the binding site [22]. However, these early studies did not dissect the role of individual RRM domains in binding the presented RNA motifs. The α-helical region of RRMs has been predicted to serve as an interface for protein–protein interactions, especially with other RRMs [20].
![Structure and sequence of HuR RRM domains. (A) Sequence alignment of RRM1, RRM2, and RRM3 conducted using Clustal Omega. Secondary structure based on existing crystal structures (PDB: 4EGL and 6GD1) shown as cartoons. Bolded red sequences represent canonical RNP sequences with aromatic residues underlined. Consensus sequences for canonical RNP2 and RNP1 based on [14] shown below wherein X represents any amino acid. (B) Structural overlay of RRM1 (black), RRM2 (blue), and RRM3 (yellow) (PDB: 4EGL and 6GD1) using Pymol. In some RRMs, there is a β-hairpin between α2 and β4 that can provide additional hydrogen bonding interactions to RNA substrates. In HuR, this structural element is seen in the crystal structures of RRM1 and RRM3 but not RRM2. (C) Representative RNP1 and RNP2 sequences shown in cyan mapped on RRM1 (PDB: 4EGL).](https://oup.silverchair-cdn.com/oup/backfile/Content_public/Journal/narmolmed/2/1/10.1093_narmme_ugaf002/2/m_ugaf002fig2.jpeg?Expires=1747873748&Signature=sw~~JOBC7Bx2G5iCl7XYaYEp7dA8~1SojDu9VmxERc6btDmSHGmOluilSSuhAGKK2tT2fsYXp8h7aVobuzOW6DpsLbt-9wmEHORaZH3r1qi7RKmztIeUIv-xIrCOE13B15N6hj9kaXopxBLF7bIPAiSq~b-3WZgIBdpwITEZAzV2TWi2oQV3EoIdZ8y4FEcshGu8xDIF~uUSRW6w-u7TSGEpjBwrgzQaBfxXPeiuNm27FAhCtzEtWh5QvFCrWRFiXVXeJtiPrUlNayJ1xPyAon-dUWmwjhAJdsvfQN43Y79KG9EgxBfC7avVpxYOYg~YoeNxOAOxKQGsfcvYx5MwrA__&Key-Pair-Id=APKAIE5G5CRDK6RD3PGA)
Structure and sequence of HuR RRM domains. (A) Sequence alignment of RRM1, RRM2, and RRM3 conducted using Clustal Omega. Secondary structure based on existing crystal structures (PDB: 4EGL and 6GD1) shown as cartoons. Bolded red sequences represent canonical RNP sequences with aromatic residues underlined. Consensus sequences for canonical RNP2 and RNP1 based on [14] shown below wherein X represents any amino acid. (B) Structural overlay of RRM1 (black), RRM2 (blue), and RRM3 (yellow) (PDB: 4EGL and 6GD1) using Pymol. In some RRMs, there is a β-hairpin between α2 and β4 that can provide additional hydrogen bonding interactions to RNA substrates. In HuR, this structural element is seen in the crystal structures of RRM1 and RRM3 but not RRM2. (C) Representative RNP1 and RNP2 sequences shown in cyan mapped on RRM1 (PDB: 4EGL).
The roughly 60-residue long unstructured linker between RRM2 and RRM3, known as the hinge region, does not appear to contribute to RNA binding [16] but rather plays a critical role in the cellular localization of HuR. Within the hinge region is the unique HuR nucleocytoplasmic shuttling sequence (HNS), which is critical for nuclear export and localization of HuR [25]. By one mechanism, the HNS is recognized by transportin 2 (Trn2) for export from the nucleus, possibly as an export adaptor for target mRNAs like c-fos [23]. Under stress conditions or heat shock, however, HuR interacts with acidic, leucine-rich phosphoprotein ligands pp23 and APRIL (acidic protein rich in leucine) to be exported by nuclear export protein CRM1 (chromosome maintenance 1). This mechanism requires both the HNS and RRM3, but the molecular details of these protein–protein interactions remain undefined [24]. The HNS spans 33 residues (205–237) of the hinge region and is fairly conserved across the Hu family. The neuronal Hu proteins contain additional nucleotides compared to HuR (Fig. 1B), but the purpose of this difference is not clear. While the HNS accounts for nearly 70% of the HuR hinge region, other residues flanking the HNS appear to be critical to HuR regulation via post-translational modifications.
Four highly conserved residues of the hinge region have been reported to undergo phosphorylation that impacts interactions with intracellular transport machinery: Y200, S202, S221, and S242 [26, 27]. Phosphorylation of Y200 by JAK3 excludes HuR from stress granules and diminishes its affinity for target mRNAs SIRT1 and VHL [28]. Phosphorylation of S202 by Cdk1 retains HuR in the nucleus and promotes its association with adaptor protein 14-3-3θ, thereby reducing the mRNA-regulating function of HuR [29]. Phosphorylation of S242 by an unknown kinase also retains HuR in the nucleus [24]. Conversely, protein kinase Cδ-mediated phosphorylation of S221 promotes HuR export from the nucleus [27].
Methylation of arginine residues, while not significantly impacting the pKa of the residue, can significantly impact its charge distribution, hydrogen bonding properties, hydrophobicity, and steric effects—all of which are integral to RNA binding [30, 31]. The conserved R217 within the HNS can be methylated by coactivator-associated arginine methyltransferase 1 (CARM1) with cell type-dependent outcomes. In a nonsmall cell lung carcinoma model, increased HuR methylation was positively correlated with its higher cytoplasmic accumulation, as well as with better prognosis for patients [32]. In HeLa cells, methylation of HuR did not impact cytoplasmic shuttling but increased association with the target mRNA p16 specifically relative to other mRNA targets like SIRT1, cyclin A, cyclin B1, and c-fos [33].
The conserved D226 within the HNS is a site of proteolytic cleavage by caspases 3 and 7 during apoptotic stress [26]. During myogenesis, one of the cleavage products (HuR-CP1, containing RRM1, RRM2, and the N-terminal portion of the hinge region) sequesters the HuR nuclear import factor TRN2, allowing uncleaved HuR to accumulate in the cytoplasm [34].
Structure and biology of RRM1 and RRM2
Crystal structures of RRM1 and 2 show the first two RRMs of HuR to be remarkably similar with an RMSD value of 0.92 Å [15]. The interdomain linker, composed of residues Y95–A106, forms a short α-helix and maintains a distance between the two domains of 13 Å, with no interdomain contacts [15]. Molecular dynamics (MD) simulations have shown an interdomain distance as long as 28.13 Å, suggesting a high plasticity of the linker [35]. The first 18 residues of RRM1, invisible in the crystal structure, are likely disordered and possess no clear or established function to date. The sequence of this N-terminal region is unique across the Hu family, and nuclear magnetic resonance (NMR) studies show that these residues are highly dynamic in solution and remain flexible upon binding to RNA, suggesting they are not directly involved in binding [36]. A recent study by Díaz-Moreno et al. showed via NMR, MD simulations, and cell-based assays that phosphorylation of Y5 within the disordered N-terminus perturbed HuR dynamics, interdomain contacts, cellular localization, and oligomerization [37]. Structurally, phosphorylation or phospho-mimetic mutation of Y5 increases the N-terminal flexibility and weakens interdomain contacts between the N-terminus and the β2-β3 loop and α1 of RRM1. In the context of full-length HuR, MD simulations indicate that phosphorylation of Y5 diminishes crosstalk between RRM1 and the second half of the RRM2–RRM3 hinge (residues 219–244), suggesting that the solvent exposure of the hinge region is dependent upon the phosphorylation and dynamics of the N-terminal disordered region.
RRM1 is understood to drive RNA-binding, both in the context of full-length HuR and truncated RRM1–2 construct [15, 16]. Li et al. measured the binding affinity of RRM1–2 to an 11-mer RNA to be 169 nM, compared to 4.88 μM for RRM1 alone and an affinity too weak to quantify for RRM2 alone, suggesting that RRM2 plays a more supportive role in binding while RRM1 plays a driving role. The crystal structure of RRM1–2 bound to RNA reveals RRM1 interacting with 5 uracil nucleotides, while the interdomain linker and RRM2 only contact 1 and 2 uracil nucleotides, respectively [15]. Outside of the N-terminal modification previously described, RRM1 undergoes phosphorylation at several other residues, each of which either causes a structural change (in order to accommodate the negative charge of the phosphate group) or impacts the affinity of HuR for target mRNAs [26]. The functional impact of phosphorylation at or near the RRMs is varied. For example, in intestinal epithelial cells, phosphorylation-deficient mutation of S88 increased binding to c-Myc RNA, while mutation of S100 in the RRM1–2 linker decreased binding [38]. The latter result was recapitulated in WI-38 fibroblasts, indicating that phosphorylation of the RRM1–2 linker at S100 may reduce affinity for at least some HuR target mRNAs [39], possibly due to electrostatic repulsion between the RNA and phosphate group. JAK3 phosphorylates Y68, located in the RNP1 site of RRM1, and Y200, located in the hinge region, but the biological function of this modification remains unclear [28].
The RRM2 of HuR has not been studied extensively in isolation. However, the significant difference in affinity for RNA substrates between RRM1–2 and RRM1 alone indicates that RRM2 provides additional contacts that directly improve RNA binding. In fact, the comparison of the apo and RNA-bound crystal structures of RRM1–2 demonstrates that RRM2 undergoes a substantial conformational change upon RNA binding, rotating a total of 137.3 degrees to create a two-sided binding cleft that sandwiches the RNA substrate between β faces of each RRM, common in RBPs containing multiple RRMs [15, 20]. This also results in new interdomain contacts at the protein level, including between RRM2 and the RRM1–2 linker, favoring the closed conformation as opposed to the open, unbound conformation in which there are no strong interactions between the domains or the domains and the linker [15].
As with RRM1, RRM2 is regulated via phosphorylation. A residue on the α-helical face of RRM2, T118, is phosphorylated by Chk2 in WI-38 fibroblasts, enhancing binding to the target mRNA COX2 [39]. Another residue on the α-helical face, S158, is phosphorylated by PKCɑ leading to cytoplasmic accumulation and thus increased stability of COX2 mRNA [32]; however, thermal stability studies show that this modification slightly destabilizes the RRM1–2 structure [40, 41]. Overall, however, phosphorylation in response to heat shock has been suggested to protect HuR from proteasomal degradation. While cell-based assays suggest that some phosphorylation events of HuR promote target mRNA stability, it is unclear whether this occurs due to changes to binding biochemistry or due to cytosolic accumulation leading to closer proximity to target mRNAs [42]. RRM2 also houses a ubiquitination site, at L182 on the β face, which appears to control this heat shock–mediated degradation [43].
Structure and biology of RRM3
Despite high structural similarity to the other RRMs (RMSD values of 0.91 and 1.3 Å to RRM1 and RRM2, respectively), biophysical studies of RRM3 have been hampered by solubility issues [16, 43, 44]. Crystallization of RRM3 was only possible after fusing the protein to a thioredoxin tag [16]. This crystal structure reveals that RRM3, like RRM1, contains an additional four-residue β-strand between α2 and β4 that is not observed in RRM2. NMR titrations of isolated RRM3 into RRM1–2 do not produce any significant structural or dynamic changes, supporting the model that RRM1–2 and RRM3 behave as two distinct functional units [43]. While these RRMs tumble in solution and bind RNA independently of each other, small-angle X-ray scattering (SAXS) and paramagnetic relaxation enhancement NMR experiments revealed that RRM1–2 does engage in transient interdomain contact with RRM3; specifically at the β face of RRM3 predicted to mitigate entropy loss during the transition from a loose, independently tumbling protein to a more rigid RNA-bound conformation [16].
While RRM1–2 has been generally agreed upon to drive target RNA binding, the role of RRM3 has been more controversial. HuR was reported to bind poly (A) stretches of RNA that are a minimum of 80 nucleotides long, with saturation being reached at 300 nucleotides [45]. Affinity for poly (A)300 was measured at 146 nM, compared to binding of the 3′ untranslated region (UTR) of HuR target, c-Myc, at 4 nM; however, it has been suggested that HuR can bind both of these RNAs simultaneously [43]. Studies of HuR suggest its RRM3 houses a DxD motif, which binds ATP and imbues Mn2+-dependent terminal adenosyltransferase activity [46]. However, more recent RNA-binding studies of RRM3 have shown a preference for U-rich rather than AU-rich RNA, and a preference for AU-rich RNA over poly (A) sequences [16, 44]. The affinity of RRM3 for RNA is consistently lower than that of full-length HuR or RRM1–2, supporting the model of RRM1–2 driving target RNA binding with RRM3 playing a secondary role [16, 44].
Despite its solubility issues, RRM3 appears to be significantly stabilized by RNA, as a crystal structure of RNA-bound RRM3 was solved without the thioredoxin tag [16, 44]. Isothermal titration calorimetry (ITC) studies of AU-rich RNA binding to RRM3 reveal a Kd of 0.65 μM and surprisingly, a stoichiometry of 2:1 RRM3:RNA [44]. A combination of NMR and ITC studies support a consensus recognition sequence of (A/U)-U-U- (A/U), with the first and fourth binding pockets of RRM3 being highly dynamic with degenerate specificity for A and U nucleotides [44]. RRM3 also contains additional aromatic residues Y249 in RNP2 and F279 in the β2–3 loop that contribute to RNA binding. RNP2 is suspected to be its major RNA binding site, as RNP2 mutations abolish RRM3–RNA binding [16]. Overall, weaker RNA affinities lead to more promiscuous binding of RRM3 to target RNAs, clouding the exact biological role of this domain in HuR function.
As with RRM1 and RRM2, RRM3 can be post-translationally modified via phosphorylation, ubiquitination, and NEDDylation. As discussed earlier, HuR phosphorylation alters its cellular localization, structural stability, and stabilization of target mRNAs. In RRM3 specifically, phosphorylation of S318 in β4 promotes binding to multiple target RNAs such as COX-2, cyclin A, and cyclin D1 without the changes to cytoplasmic shuttling that are seen in other examples of HuR phosphorylation [27, 47]. Via a likely separate mechanism, HuR can be ubiquitinated by two forms of ubiquitin, short K29-linked polyubiquitin chains or longer K48-linked chains; K48-linked chains target HuR for proteome-mediated degradation while K29-linked chains, attached to K313 and K326, function as a nondegradative signal and instead promote interaction with the p97–UBDX8 remodeling complex which can release HuR from a bound target mRNA [48]. Finally, HuR can be NEDDylated at three conserved lysine residues within RRM3; K283, K313, and K326 [49]. NEDDylation is the covalent attachment of NEDD8, a small ubiquitin-like protein, to proteins in a process in order to regulate their function in a variety of ways [50]. Addition of NEDD8 to HuR by Mdm2, especially to K326, promotes nuclear localization and thus protection from proteome-mediated decay [49].
The question of dimerization
HuR is consistently reported to exist as an oligomer, however, explanations of the mechanism and biological relevance of this process are varied [16, 36, 43, 44, 51–53]. The HuB, HuC, and HuD were shown to form heterodimers, via a yeast two-hybrid screen, and HuR dimers were identified via a crosslinking assay [54]. Two major models of Hu dimerization involve either the tandem RRM1–2 domains, mostly facilitated by RRM1 (Fig. 3A), or dimerization of RRM3 (Fig. 3B). Both models are supported by literature, though little discussion exists about their contradictory or cooperative nature.
![Working models of HuR dimerization. (A) Model of RRM1-mediated dimerization resulting from changed interdomain contacts caused by phosphorylation of Y5, based on findings in [29]. (B) Model of RRM3- and RNA binding-mediated dimerization, based on findings in [16, 36]. Created in BioRender. Clark, M. (2025); https://BioRender.com/e31h639.](https://oup.silverchair-cdn.com/oup/backfile/Content_public/Journal/narmolmed/2/1/10.1093_narmme_ugaf002/2/m_ugaf002fig3.jpeg?Expires=1747873748&Signature=1ccDN5EM98-3emwNe97qIQlwr8BTSwCl~haipp79u~-QHLNX9oUe-is8SA~OUD4t2SVKqBwI8q~VU9Il~waShz1MIchy~Pva3MvK3UhjWyqyVe5hgST1XMB2oJ81K6C0GYuTXk8y~PepOYzllVt02FvRnoBM6JovYEGku19ewzqlpDJjscAfQD8eZ4hkeyQcGGEaF9GLaQLF6LuFPhpFwSTbnWS9bicQGfbJzdTlyojGzmNYsARMuoq11lA6PjDmJBjkDVzUNtwmFB6BM-v3V5O-AeH7A52ogzr8gVH4LbuTSlhLZJKxIsEbg08ao7kI9h-fBH1FFuS1DnT36kbkGQ__&Key-Pair-Id=APKAIE5G5CRDK6RD3PGA)
Working models of HuR dimerization. (A) Model of RRM1-mediated dimerization resulting from changed interdomain contacts caused by phosphorylation of Y5, based on findings in [29]. (B) Model of RRM3- and RNA binding-mediated dimerization, based on findings in [16, 36]. Created in BioRender. Clark, M. (2025); https://BioRender.com/e31h639.
Auer et al. measured HuR homodimer formation to be tight (KD of 10–100 pM) with a nonreducing gel showing ∼25% dimeric form for both full-length HuR and a truncated construct consisting of only RRM1–2 [51]. In a later study, the same group measured the homodimer formation again with a KD of 100 pM but noted that no dimers were observed when the first 17 residues of RRM1 were removed, implicating the N-terminal disordered region of RRM1 in dimerization [52]. An N-terminal C13A mutation prevented dimerization, implicating a disulfide bond in the formation of HuR dimers that has since been proposed to be a cysteine-dependent redox-sensing mechanism [52]. The model of RRM1-driven dimerization was supported by a study of oligomeric transitions of RRM1 using solution NMR. The overall correlation time (tc) of RRM1 varied slightly with concentration, from 8 ns at 1.5 mM to 7.1 ns at 0.25 mM, indicating slower tumbling at higher concentrations, which can signify greater molecular weight [36]. Furthermore, electrospray ionization–ion mobility spectrometry–mass spectrometry (ESI–IMS–MS) of RRM1 revealed the presence of a population of dimers which mostly disappear upon binding a DNA substrate, suggesting that this dimerization occurs at the expense of the nucleic acid binding capability of RRM1 [36].
However, the ESI–IMS–MS findings also challenged a cysteine-dependent mechanism presented by Auer et al., since dimers formed even in the presence of 5 mM reducing agent [36]. Furthermore, when RRM1 was titrated into itself by NMR, the largest chemical shift perturbations mapped to an ɑ-helical face and β-hairpin distal to the expected N-terminal disordered region. However, it is important to note that these chemical shift perturbations were relatively small (<0.05 ppm) [36]. While a dimerization constant was not reported, it was suggested that RRM1 dimers represented a small population of HuR, even at concentrations as high as 1.5 mM, and dimers were not observed via size-exclusion chromatography at low μM concentrations [36].
Data describing the dimerization of RRM3 are much more robust. NMR signals of RRM3 broaden with increasing concentration, and the signals for residues W261–T271 within ɑ1 of RRM3 are missing entirely [43]. This was hypothesized to be oligomerization leading to intermediate timescale conformational exchange that is not visible by NMR, supported by the HuR homolog ELAV in Drosophila that dimerizes by the homologous tryptophan residue on ɑ1 of its third RRM. This latter process is dependent upon RNA binding [55]. Analytical ultracentrifugation (AUC) of isolated RRM3 suggests an association constant for dimers of 18 mM, with a small fraction of the population forming ∼80 kDa aggregates; however, mutation of W261 to negatively charged glutamate led to the disappearance of monomer–dimer equilibrium on AUC (while still forming high molecular weight aggregates) and the appearance of the W261–T271 NMR signals with no changes to overall structure [43]. MD simulations show that ɑ1 (a putative dimerization interface) is flexible, and during simulation trajectories, W261 rotates by 160 degrees, supporting its potential rearrangement for monomer–monomer interactions.
The RRM3 ɑ1 helix was again implicated in dimerization through NMR studies of isolated RRM3 where concentration-dependent chemical shifts mapped to the first half of ɑ1 and to the RNP1 RNA-binding site. Concentration-dependent NMR spectral changes were also abolished by a W261E mutation [44]. These results were recapitulated in a separate study of full-length HuR with the hinge region replaced by Gly-Gly-Ser repeats, which in conjunction with SAXS showed that full-length HuR is dimeric even at concentrations as low as 14 μM and that dimers are abolished by the W261E mutation [16]. Measurements of tc also indicated the presence of oligomers, with values of 9.05, 13.6, and 7.31 ns for WT RRM3 at 80 mM, WT RRM3 at 300 mM, and the W261E mutant at 300 mM, respectively [44]. The crystal structure of RRM3 indicates that dimerization likely occurs through stacking of the W261 residue on each monomer as well as hydrogen bonding between several other residues of neighboring ɑ1 helices [44]. The tc value of WT RRM3 at 80 mM, when compared to the W261E mutant, indicates that even at low protein concentrations, some population of oligomer exists [44].
Calorimetry revealed increased affinity for 11-mer RNA compared to 6-mer RNA for WT RRM3 (0.7 mM versus 3.58 mM), with stoichiometries indicating that the 11-mer could accommodate two RRM domains. However, at RNA lengths >11 nucleotides, WT RRM3 had significantly higher affinity for RNA compared to the dimerization-deficient mutant, suggesting a link between dimerization and RNA affinity [44]. This is consistent with the concentration-dependent NMR chemical shifts of RRM3 mapped to the ɑ1 helix and RNP1 motif and implies allosteric coupling of RRM3 dimerization and RNA binding [44]. Filipowicz et al. demonstrated via gel-based experiments that HuR required its hinge region or RRM3 to oligomerize along RNA substrates and relieve RNA targets of the miRNA-induced silencing complex (miRISC), suggesting a biologically relevant role for C-terminal dimerization of HuR [56]. Studies of HuR dimerization in Huh7 cells using a luciferase reporter assay found that overexpression of the full-length W261E mutant or of a full-length mutant with an RNA binding-deficient RRM3 promoted target mRNA stability, while this effect was abolished by binding-deficient mutations in RRM1–2 [44]. These results suggest not only that RRM3–RNA binding is dependent upon RRM3 dimerization in Huh7 cells (as the RRM3 mutant deficient in both dimerization and RNA binding showed no difference compared to dimerization-deficient alone), but that RRM3 dimerization may play an auto-inhibitory role to reverse the stability conferred by RRM1–2 binding, possibly by competition with RRM1–2. It is important to note that these effects may be cell line-dependent, as results differed slightly in C3H/10T1/2 cells [44].
Current data indicates that RRM1–2 (via the RRM1 domain) and RRM3 are able to dimerize in vitro, with possible contributions from the hinge region in some mechanisms [16, 36, 43, 44, 51, 56]. While RRM3-mediated dimerization is better substantiated, further investigation is needed in the context of the full-length protein to determine biologically relevant oligomeric states of HuR in cells and tissues. It is possible that both models of dimerization could exist under different conditions, such as in response to unique cellular stimuli or post-translational modifications. For example, phosphorylation of Y5 in the N-terminal disordered region enhances HuR dimerization by an order of magnitude biophysically and in HeLa cells, but this dimerization is ablated under reducing conditions [37]. It is even postulated that unmodified HuR dimerizes exclusively via RRM3, while HuR phosphorylated at Y5 undergoes a conformational change (which disrupts interactions between the hinge region and the α1 and the β2–β3 loop of RRM1) that permits RRM1-mediated dimerization through the α-helical face [37]. This presents a possible model in which all existing data can be leveraged to explain biologically relevant HuR dimerization, pending further validation.
HuR and biomolecular phase separation
Like many RBPs, HuR has a known role in stress granules, a form of liquid–liquid phase separation that results in RNA and RNA-associated proteins sequestered within the cytosol [57]. As their name suggests, stress granules appear in response to stimuli such as heat, oxidative stress, or hyperosmolarity. It is hypothesized that stress granules exist as a triage mechanism for translation, wherein the structure and composition of translation-stalled mRNA molecules are evaluated and sorted to be degraded, re-initiated, or packaged into stable messenger ribonucleoprotein (mRNP) complexes [58]. In a cellular context, the purpose of stress granules appears to be to halt translation of so-called “housekeeping” mRNAs and promote the translation of stress-response and repair-mediating mRNAs, which is accomplished through the manipulation of relative mRNP concentrations within the granules themselves, as opposed to in the cytosol.
HuR is primarily localized to the nucleus but is exported into the cytoplasm upon various triggers. In response to stress, HuR often localizes to stress granules [59]. Silencing or inhibiting the tyrosine kinase JAK3 prevents HuR from localizing to stress granules in response to arsenite treatment, a cellular stressor that causes oxidative stress [28]. Specifically, JAK3 phosphorylates HuR at Tyr200, which is within the unstructured RRM2–3 hinge region. This modification excludes HuR from stress granules as well as promotes dissociation from target mRNAs such as SIRT1 and VHL.
HuR has also been implicated in processing bodies (P-bodies), a related type of cytoplasmic RNP granule. Like stress granules, P-bodies rely on a network of RNA–protein interactions and liquid–liquid phase separation to form. Unlike stress granules, which sequester translation-stalled mRNAs with translation machinery, P-bodies primarily contain machinery associated with translational repression and mRNA decay. However, the two have been known to dock with one another and exchange mRNPs [60, 61]. HuR has been implicated in the “rescue” of at least one target mRNA from association with the microRNA-induced silencing complex (miRISC) in P-bodies during stress. While CAT-1 mRNA is typically sequestered into P-bodies by miR-122 in Huh7 cells, upon amino acid starvation-induced stress, the mRNA colocalizes to the cytosol with HuR. This relocation is dependent upon HuR as well as an AU-rich portion of the CAT-1 3′ UTR [61, 62]. A later study by Yu et al. showed that HuR overexpression prevents the localization of a different target mRNA, E-cadherin, to P-bodies in Caco-2 cells; however, the exact mechanism of this preclusion is unclear. It is possible that HuR accomplishes this simply by out-competing other RBPs such as CUGBP1 which would localize the mRNA to P-bodies [63]. With the vast number of stress-related mRNAs targeted by HuR, it is not out of the realm of possibility that HuR may shuttle mRNAs out of P-bodies and possibly to stress granules to manipulate their translation. As mentioned, it has been suggested that polysomes, stress granules, and P-bodies constitute an mRNP cycle wherein mRNAs are exchanged throughout; mRNAs sequestered in P-bodies can return to translation, likely due to changes in the mRNP formed. Furthermore, while proteins have been observed to translocate from P-bodies to stress granules during times of stress, no evidence has been found of the reverse. Overall, HuR has been reported to have interactions with cytoplasmic bodies such as stress granules and P-bodies, and is even considered a marker of stress granules [64].
Biomedical relevance
The physiological impact of HuR on development was first appreciated in studies of Drosophila, demonstrating its essential role in embryonic development of the eye and optic lobe and earning the early gene name, embryonic lethal abnormal visual system (elav) [11]. Embryonic lethality was also evident at stage E9.5 in the murine model of Elavl1 gene-deletion [65]. Elavl1+/− were bred to obtain Elavl1−/− newborns which manifest placental defects involving impaired morphogenesis and vascularization of the placental labyrinth critical for fetal support [59]. In addition to the impact on the placental labyrinth vasculature and morphogenesis, there were defects in skeletal ossification and splenic ontogeny.
Much of the HuR biology centers on recognizing and binding to certain AREs on RNA transcripts. HuR commonly binds to AREs found in the 3′ UTR of RNA transcripts to enhance transcript stability. Seed sites specific to microRNAs (miRNAs) are often found within the vicinity of HuR binding sites, supporting the potential for crosstalk between HuR and miRNA regulatory activity [66]. HuR can bind to intronic sites of RNA transcripts with a preference for splice sites suggesting a role for HuR in generation of alternative splice variants of the transcript [66]. To test alternative splkicing effects of HuR, PCR analysis was performed in a HuR knockdown testing for expression of different exons. Exon 10 of PTBP2, which is flanked by HuR binding sites, had a 65% increase in expression compared to the flanking exon 9 and 11. Further PCR analysis revealed that HuR did impact splice variants for four of six tested candidate exons, confirming HuR function in RNA processing [66]. miRNA precursor coding regions exist in introns, with their expression being connected to expression of the host gene or to RBPs during RNA processing. Since HuR was found to bind intronic sites and is an RBP, it was predicted that HuR could regulate miRNA biogenesis. Investigation of different miRNAs in HuR knockdown conditions identified miR-7 as being strongly regulated by HuR, supporting the role of HuR in miRNA biogenesis [66].
Investigation of HuR has also shown its importance in hematopoietic stem cell growth and maturation. Murine bone marrow HuR deletion demonstrated a decrease in hematopoietic stem cell populations but had no impact on mature cell populations [67]. B-cell progenitor cells also demonstrated increased evidence of apoptosis and necrosis, which was not found in the mature B cells. The T-cell progenitor population was similarly affected, specifically in double negative (CD4− CD8−) cells. To understand why loss of HuR caused a significant impact on hematopoietic stem cell populations, a microarray analysis was performed to identify transcript expression changes. Altered expression for transcripts involved in hematopoiesis (Sox2), apoptosis (c-Myc), and cell cycle (p21) were identified [67]. Transcripts involved in regulating the p53 pathway were found to be upregulated upon loss of HuR, suggesting a role for HuR in p53 suppression. Specifically, HuR was shown through immunoprecipitation to bind to the ARE of Mdm2, an upstream regulator of p53, through an immunoprecipitation assay. In murine embryonic fibroblasts, HuR knockdown caused an increase in the mRNA, p19ARF, an inhibitor of Mdm-2 function. Therefore, while loss of HuR led to repression of Mdm-2, it is possible that the induction of p53 was due to the p19ARF inhibitory function. While the focus was on the transcripts involved in the p53 pathway, it is likely that HuR can bind to partner proteins and regulate additional pathways [68].
Further work identified HuR as a key factor in T-cell activation and maturation which may play into its impact on splenic development. Conditional genetic ablation of HuR in early stage thymocytes resulted in a reduction in peripheral T-cells that was exacerbated by aging the mice [69]. The loss of HuR led to a reduction in p53 protein but no change in p53 mRNA levels in double negative (CD4− CD8−) cells during T-cell receptor selection [67]. This differs from what was found previously [67] which may reflect differences in the cell types studied, thymocytes [69] and total bone marrow cells [65]. HuR-deleted T-cells were also defective in egress from the thymus, leading to reductions in the peripheral T-cell population [69]. Localization of HuR to the nucleus appears to be an important regulatory event. At baseline, HuR is primarily localized to the nucleus until cellular stimulation whereby it is translocated to the cytoplasm [70]. Lymphocyte function-associated antigen 1 (LFA-1), a T-cell β2 integrin adhesion receptor, was identified as one such stimulus that activates T-cells, leading to translocation of HuR from the nucleus to the cytoplasm [70]. LFA-1 engagement was identified to activate the Rac1 and Rac2 GTPases as signaling intermediaries leading to activation of HuR and consequent stabilization of mRNA by HuR in an MKK3-dependent manner [71]. These studies established HuR as having an essential role in bone marrow progenitor cell survival as well as B-cell and T-cell maturation [67].
HuR has also been studied in post-developmental angiogenesis and arteriogenesis. Many angiogenic factors like vascular endothelial growth factor A (VEGF-A) are encoded by mRNAs bearing AREs that confer intrinsically short half-lives, limiting protein translation potential. RBPs, and in particular HuR, can bind to and stabilize ARE-bearing transcripts, prolonging mRNA half-life and increasing translation [72]. In inflammatory macrophage populations, engagement of leukocyte β2 integrins triggered a rapid molecular switch that results in the HuR-mediated stabilization of multiple pro-angiogenic transcripts, including GM-CSF, VEGF-A, and MMP-9 [70, 70, 73, 74]. Signaling of the major monocyte chemokine CCL2 (MCP-1) through its receptor, CCR2, activates β2 integrins and is vital to arteriogenesis in animal models of hind limb ischemia [75–77]. CCL2 stimulation both promoted and synergized with β2 integrin adhesion to rapidly activate myeloid HuR-dependent VEGF-A expression and consequent inflammatory arteriogenesis signaling through a Rac2–Myosin IIA-mediated signaling mechanism [70]. Studies have demonstrated that HuR and microRNAs like miR200b can play antagonistic roles in the regulation of VEGF-A mRNA expression [78]. However, examples of cooperation between HuR and miRNAs to downregulate mRNA expression have also been identified, indicating more complexity to the regulator potential of the HuR-miRNA axis with respect to mRNA stability [79]. Examples include miRNAs let-7 and miR19 that function with HuR to repress c-Myc and RhoB, respectively [80, 81].
In patients with ischemic heart failure and in a coronary artery ligation model of ischemic heart failure, expression of HuR was found to be reduced in the left ventricular muscle tissue [82]. This reduction of HuR corresponded with downregulation of SCN5A, the major ⍺ subunit comprising the cardiac sodium ion channel. In fact, HuR stabilized SCN5A mRNA and that the overexpression of HuR rescued the expression of SCN5A in the mouse model, indicating a potentially important role for HuR in preventing arrhythmogenic potential in the failing heart [82]. In studies of cardiac microvasculature and microvascular dysfunction in the context of diabetes, endothelial cells exhibited reduced HuR expression in both preclinical models and patient samples [83]. Consequently, HuR binding to and stabilization of connexin 40 (Cx40) mRNA was identified as a key impairment associated with endothelial dysfunction in the context of diabetes. Cx40 serves as a major a gap junction protein important for regulating endothelial migration, survival, vessel integrity, and endothelial-dependent relaxation. Further, overexpression of Cx40 in HuR-deleted human cardiac endothelial cells led to increased capillary network formation and improved coronary flow velocity reserve [83]. In sum, the regulation of HuR levels is critical for supporting healthy cardiovascular function.
Dysregulated HuR expression and activity has negative implications in several cancers. HuR expression is tightly regulated through multiple processes including self-regulation where HuR binds to and facilitates stability of its own mRNA [84]. Conversely, miR-519 [85] and miR-125a [86] bind to the HuR mRNA and inhibit its translation. Because HuR often increases the stability of growth factor or cytokine mRNAs, it may promote tumor development and/or tumor survival in some disease states. For example, while HuR promotion of VEGF-A expression is important for vascular function, the increased VEGF-A expression associated with HuR binding to and stabilizing of VEGF-A mRNA led to an exacerbation of the severity of astrocytic tumors [87]. In a colorectal cancer model, HuR was found to be elevated in the cytoplasm of cancer cells and consequently bound to the ARE of COX-2 mRNA, leading to increased COX-2 expression, which is common to this cancer type [88, 89]. A similar model has been suggested in ovarian carcinoma, whereby HuR-mediated stabilization of COX-2 led to increased COX-2 protein expression associated with the cancer [90, 91]. Of note, COX-2 is expressed at basal levels under normal conditions but it is induced by growth and inflammatory factors, leading to changes in prostanoid synthesis that can be associated with cancer development [88]. In a breast cancer model, HuR increased the mRNA stability of the cyclin E1, which has been associated with many types of cancer because of its important role in binding to Cdk2 to trigger transition between G1 and S phase [92, 93]. In direct correlation with the increased measures of mRNA stability, a common thread in many of these studies was enhanced HuR translocation to the cytoplasm, supporting dysregulation of HuR translocation as a potential common disease mechanism as well as a diagnostic marker of cancer onset and/or severity. Consequent HuR binding to target mRNAs often leading to RNA stabilization is a critical outcome of cytosolic translocation but more importantly has a major impact in cancer cell survival and biology.
Due to the implication of HuR in different diseases and cancers, targeting HuR is an attractive candidate for future targeted therapies. For example, HuR has been implicated in breast cancer and increases in its cytoplasmic translocation have been associated with higher-grade malignancies, indicating that targeted inhibition might help mitigate disease severity [94–96]. Due to HuR binding to the ARE of mRNA transcripts often preventing the degradation of the transcript, one therapeutic option would be to utilize a small molecule inhibitor that would disrupt the interaction between HuR and mRNA transcripts within cancer cells. One such inhibitor, KH-3, disrupts the interaction between HuR and FOXQ1 mRNA leading to a decrease in tumor growth and metastasis [96]. A similar small molecule inhibitor of HuR, SRI-42127, inhibited HuR translocation in microglia cells while also suppressing inflammatory cytokines leading to a decrease neuroinflammation [97]. Increase in neuroinflammation has been associated with worsening of neurodegenerative diseases [98]. While promising, few inhibitors have been viable as therapies in the clinic due to off-target effects and due to the ubiquitous expression of HuR in multiple cell types throughout the body [99]. Another approach is to utilize proteolysis targeting chimeras (PROTAC) to inhibit HuR. This is a system where a heterobifunctional molecule binds the protein of interest while connecting the protein to a ligand of the E3 ligase [100]. This leads to the degradation of the protein of interest through the ubiquitin proteasome system machinery [101]. This is a promising new therapy strategy with a number of PROTACs entering phase 3 trials [102]. Recently, a PROTAC has been identified that can bind and degrade HuR and could be used in highly targeted future therapies [103].
Broader applications of HuR research
While over half of all human RBPs contain at least one RRM domain, there is incredible versatility in the functionality and interactions of these domains, especially in proteins that contain multiple RRMs [19]. A recent study found that of the 230 known RRM-containing RBPs, at least 37% contain more than one RRM; however, of the multi-RRM RBPs, less than half contain more than two RRMs [100]. While many proteins containing three or more RRMs have been studied in recent years, structural information spanning the entire protein and therefore all of the RRMs remains very limited, with most research focusing on isolated constructs of single RRMs.
For example, RNA-binding protein 45 (RBM45) has a similar overall domain architecture to HuR, with two N-terminal RRMs separated from a third RRM by a long unstructured linker; however, the linker regions are much longer than in HuR (15 residues and 197 residues between RRM1–2 and RRM2–3, respectively). The crystal structure of RBM45 RRM1–2 complexed with RNA reveals that the two RRMs do not create a sandwiched binding cleft as seen in HuR, but instead each bind five nucleotides of different RNA molecules [105]. A follow-up study of the isolated third RRM revealed a similar binding orientation with a slightly different nucleotide preference [106]. However, no structural models currently exist to explain how the three RRMs interact with RNA in the context of the full-length protein or a full-length target RNA molecule.
Other multi-RRM-containing RBPs include polypyrimidine tract-binding protein (PTB), which has four RRMs with linker lengths of 41, 103, and 43 residues between RRM1–2, RRM2–3, and RRM3–4, respectively. Mickleburgh et al. [107] used tethered hydroxyl radical probing to demonstrate an unequal division of labor across RRMs when binding target pre-mRNA FAS and that, importantly, RRM3 packs together with RRM4 to create a structure that provides an extended positively charged binding surface. Therefore, despite having the lowest inherent binding affinity of any PTB domain, RRM3 is the most important for function.
Overall, proteins with three or more RRM domains appear to display great variety in domain architecture, organization, and interaction between the sibling RRMs. The majority of these proteins are comprised of over 300 residues [104], thus current biophysical techniques are limited in their ability to provide a full molecular “picture” of their structures, functions, and mechanisms. HuR represents a multi-RRM protein with significant biomedical, cellular, and biophysical research on both the full-length protein and all three of its functional domains. While many questions still remain, HuR represents an example of divide-and-conquer biochemistry that has provided insight into how multi-domain RBPs function by leveraging a unique architecture, as well as dynamics of each protein region and how these mechanisms impact homeostatic and disease states.
Acknowledgements
Graphical abstract was created in BioRender. Clark, M. (2025); https://BioRender.com/u93v925.
Author contributions: M.E.C. and A.F. wrote the first draft of the manuscript. A.R.M. and G.P.L. revised the initial drafts. The final version of the manuscript was written through contributions from all authors.
Conflict of interest
None declared.
Funding
This work was supported by the NIH grant R01 HL163005 to A.R.M. and G.P.L. Funding to pay the Open Access publication charges for this article was provided by theDivision of Intramural Research grant R01 HL163005.
Data availability
No new data were generated or analyzed in support of this research.
Comments