-
PDF
- Split View
-
Views
-
Cite
Cite
Jessica L Norris, Lindsey O Rogers, Kara G Pytko, Rachel L Dannenberg, Samuel Perreault, Vikas Kaushik, Sahiti Kuppa, Edwin Antony, Mark Hedglin, Replication protein A dynamically re-organizes on primer/template junctions to permit DNA polymerase δ holoenzyme assembly and initiation of DNA synthesis, Nucleic Acids Research, Volume 52, Issue 13, 22 July 2024, Pages 7650–7664, https://doi.org/10.1093/nar/gkae475
- Share Icon Share
Abstract
DNA polymerase δ (pol δ) holoenzymes, comprised of pol δ and the processivity sliding clamp, PCNA, carry out DNA synthesis during lagging strand replication, initiation of leading strand replication, and the major DNA damage repair and tolerance pathways. Pol δ holoenzymes are assembled at primer/template (P/T) junctions and initiate DNA synthesis in a stepwise process involving the major single strand DNA (ssDNA)-binding protein complex, RPA, the processivity sliding clamp loader, RFC, PCNA and pol δ. During this process, the interactions of RPA, RFC and pol δ with a P/T junction all significantly overlap. A burning issue that has yet to be resolved is how these overlapping interactions are accommodated during this process. To address this, we design and utilize novel, ensemble FRET assays that continuously monitor the interactions of RPA, RFC, PCNA and pol δ with DNA as pol δ holoenzymes are assembled and initiate DNA synthesis. Results from the present study reveal that RPA remains engaged with P/T junctions throughout this process and the RPA•DNA complexes dynamically re-organize to allow successive binding of RFC and pol δ. These results have broad implications as they highlight and distinguish the functional consequences of dynamic RPA•DNA interactions in RPA-dependent DNA metabolic processes.
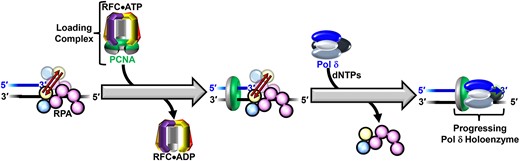
Introduction
In humans, DNA polymerase δ (pol δ) holoenzymes, comprised of pol δ and the processivity sliding clamp, proliferating cell nuclear antigen (PCNA), carry out DNA synthesis during lagging strand DNA replication, the initiation of leading strand DNA replication, as well as the major DNA damage repair and tolerance pathways (1–11). In each of these contexts, pol δ holoenzymes are assembled at primer/template (P/T) junctions and initiate DNA synthesis in a stepwise process (summarized in Figure 1) that involves many factors that all directly interact with P/T junctions; namely, the major single strand DNA (ssDNA)-binding protein complex, replication protein A (RPA), the PCNA loading complex, replication factor C (RFC), PCNA and pol δ (12–22). First, an RPA engages the P/T junction, primarily through interactions with the adjacent template ssDNA. RPA is comprised of three subunits, denoted RPA1–3, that each contain one or more oligonucleotide binding (OB) folds that interact with ssDNA and/or proteins. RPA1 contains four OB folds, three of which (A – C) bind DNA. OBF of RPA1 is primarily involved in protein•protein interactions. RPA2 contains OBD that binds DNA. RPA3 contains OBE that primarily serves as a structural scaffold for individual RPA complexes (23–25). RPA engages a P/T junction in an orientation-specific manner (as depicted in Figure 1). Specifically, RPA1 OBA aligns away from the P/T junction on the template ssDNA downstream and RPA2 OBD aligns closer to the P/T junction such that RPA2 OBD and RPA1 OBC directly contact the 3′ terminus of the primer strand and/or the template ssDNA immediately adjacent to the P/T junction. Collectively, OB folds A – C of the RPA1 subunit and OBD of the RPA 2 subunit engage 30 – 33 nt of the template ssDNA adjacent to a P/T junction (12–19). Next, RFC, with bound ATP, engages the ‘front face’ of a free PCNA, opens the clamp, and the resultant complex, referred to herein as the loading complex, engages a P/T junction such that the ‘front face’ of PCNA is oriented towards the 3′ terminus of the primer (20). Upon engaging a P/T junction, the loading complex adopts an activated conformation in which ATP hydrolysis by RFC is optimized. Herein, the activated conformation of the loading complex is referred to as the activated loading complex. ATP hydrolysis by RFC simultaneously closes PCNA around the DNA and the closed (i.e. loaded) PCNA is subsequently released onto the double stranded DNA (dsDNA) region of the P/T junction. The resultant RFC•ADP complex then releases into solution via dissociation from the resident RPA (20,21,26,27). After completion of PCNA loading, the resident RPA stabilizes loaded PCNA at/near the P/T junction by prohibiting RFC-catalyzed unloading of PCNA and diffusion of PCNA along the adjacent ssDNA (27). Diffusion of loaded PCNA along the upstream dsDNA region is restricted by ‘protein roadblocks,’ such as sub-nucleosomes and high-affinity transcription factors, that rapidly re-engage nascent DNA generated during DNA replication (28–30). Next, pol δ engages the ‘front face’ of loaded PCNA, forming a holoenzyme. Finally, the assembled pol δ holoenzyme engages the P/T junction, aligns an incoming, complimentary dNTP for incorporation, and initiates DNA synthesis (27,31,32).
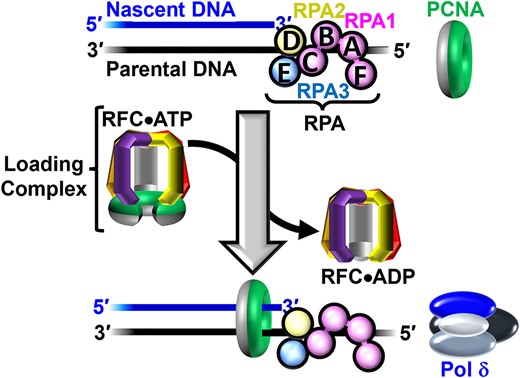
Assembly of pol δ holoenzymes and initiation of DNA synthesis. Nascent DNA (primer) and parental DNA (template) strands are blue and black, respectively. RPA subunits are color-coded and depicted to illustrate the OB-folds (A–E). The ‘front’ and ‘back’ faces of PCNA are green and grey, respectively. A P/T junction is pre-engaged by RPA. RFC utilizes ATP to load PCNA onto a P/T junction such that the 'front face' of the clamp is oriented towards the 3′ terminus of the primer strand. Pol δ then engages the 'front face' of loaded PCNA, forming a holoenzyme and initiates DNA synthesis (not shown).
During assembly of a pol δ holoenzyme and/or initiation of DNA synthesis, RPA, RFC and pol δ all directly engage the 3′ terminus of the primer strand and at least ∼6 nucleotides (nt) of the template ssDNA immediately adjacent to a P/T junction (12–19,31,32). A burning issue that has yet to be resolved is how the mutual exclusivity of these overlapping protein − DNA interactions are accommodated during this process. To address this, we design and utilize novel, ensemble Förster Resonance Energy Transfer (FRET) assays that directly and continuously monitor the interactions of RFC, PCNA, RPA and pol δ with DNA as pol δ holoenzymes are assembled and initiate DNA synthesis. Results from the present study reveal that the conformation of the RPA•DNA complex dynamically re-organizes to vacate the P/T junction while remaining engaged with the template ssDNA downstream of the P/T junction. This permits pol δ holoenzyme assembly and initiation of DNA synthesis and increases the efficiency of this process by stabilizing PCNA at/near the P/T junction.
Materials and methods
Oligonucleotides
Oligonucleotides (depicted in Supplementary Figure S1) were synthesized by Integrated DNA Technologies (Coralville, IA) or Bio-Synthesis (Lewisville, TX) and purified on denaturing polyacrylamide gels. The concentrations of unlabeled DNAs were determined from the absorbance at 260 nm using the provided extinction coefficients. The concentrations of Cy5-labeled DNAs were determined from the extinction coefficient of Cy5 at 650 nm (ϵ650 = 250 000 M−1cm−1). Concentrations of Cy3-labeled DNAs were determined from the extinction coefficient of Cy3 at 550 nm (ϵ550 = 136 000 M−1cm−1). For annealing two ssDNAs (as depicted in Supplementary Figure S1), the primer and corresponding complementary template strand were mixed in equimolar amounts in 1× annealing buffer (10 mM Tris–HCl, pH 8.0, 100 mM NaCl, 1 mM EDTA), heated to 95 °C for 5 min, and allowed to slowly cool to room temperature.
Recombinant human proteins
Human RPA, Cy5-PCNA, exonuclease-deficient pol δ (referred to herein as simply pol δ) and RFC were obtained as previously described (21,33). The concentration of active RPA was determined via a FRET-based activity assay as described previously (34). Human RPA containing a Cy5 label at either RPA2 residue 107 within OBD (RPA-OBD-Cy5) or RPA1 residue 215 within OBA (RPA-OBA-Cy5) was obtained essentially as described for S. cerevisiae RPA (24). Characterization of the FRET signal resulting from the interaction of each Cy5-labeled RPA with the corresponding Cy3-labeled P/T DNA substrate is shown in Supplementary Figure S2. The concentration of active Cy5-labeled RPA is determined by a FRET-based assay that is described in detail in the Supplementary Information and the data presented in Supplementary Figure S3.
Pre-steady state FRET measurements
All experiments were performed at room temperature (23 ± 2°C) and, unless indicated otherwise, in 1× Mg2+/Ca2+ buffer (20 mM HEPES, pH 7.5, 150 mM KCl, 5 mM MgCl2, 5 mM CaCl2) supplemented with 1 mM DTT, 1 mM ATP and the ionic strength adjusted to physiological (200 mM) by addition of KCl. Ca2+ is included to account for experimental conditions in future studies and the presence of Ca2+ does not affect the amount of RPA that binds to ssDNA (Supplementary Figure S4) nor the amount of PCNA loaded onto DNA by RFC (Supplementary Figure S5 and Supplementary Table S1). All experiments were performed in a 16.100F-Q-10/Z15 sub-micro fluorometer cell (Starna Cells) and monitored in a Horiba Scientific Duetta-Bio fluorescence/absorbance spectrometer. Reaction solutions are excited at 514 nm and the fluorescence emission intensities (I) are monitored essentially simultaneously (Δt = 0.235 ms) at 563 nm (I563) and 665 nm (I665) over time, recording I every 0.17 s. For all experiments, excitation and emission slit widths are 10 nm. For all recordings of I665 and I563, the approximate FRET efficiency (EFRET) is estimated from the equation |${E}_{FRET} = \frac{{{I}_{665}}}{{{I}_{665} + {I}_{563}}}$|. All recorded I values are corrected by a respective dilution factor and all time courses are adjusted for the time between the addition of each component and the I recording (Δt ≤ 10 s). To determine the predicted EFRET trace for a Cy5-labeled RPA remaining completely disengaged from a corresponding Cy3-P/T DNA, I665 and I563 for a Cy5-labeled RPA alone and a reaction mixture lacking only the respective Cy5-labeled RPA are each monitored, and EFRET is calculated as follows:
To determine the predicted EFRET trace for a loading complex pre-formed with ATPγS and Cy5-PCNA remaining completely disengaged from a Cy3-P/T DNA•RPA complex, I665 and I563 for the loading complex alone and a reaction mixture lacking only the loading complex are each monitored, and EFRET is calculated as follows:
For each experiment below, the final concentrations of all reaction components are indicated. The concentrations of ATP, ATPγS, dGTP and dCTP in all solutions described below are each 1.0 mM and, hence, this concentration is maintained for each upon mixing. For all PCNA loading reactions, the loading is stoichiometric in that a single PCNA is engaged with each P/T DNA as part of a loading complex or as loaded PCNA (27).
For PCNA loading experiments in the presence of ATPγS, a Cy3-labeled P/T DNA (20 nM, Supplementary Figure S1), NeutrAvidin (80 nM homotetramer), and ATPγS are pre-incubated with an RPA (25 nM heterotrimer, RPA, RPA-OBA-Cy5 or RPA-OBD-Cy5), the resultant solution is transferred to a fluorometer cell, and the cell is placed in the instrument. I665 and I563 are monitored until both stabilize for at least 1 min. Within this stable region, EFRET values are calculated and averaged to obtain the EFRET value observed prior to addition of the loading complex. Finally, loading complexes pre-formed with a PCNA (20 nM homotrimer, Cy5-PCNA or PCNA), RFC (20 nM heteropentamer) and ATPγS are added, the resultant solution is mixed (via pipetting), and I665 and I563 are monitored, beginning 10 s after the addition of loading complex (i.e. Δt = 10 s).
For PCNA loading experiments in the presence of ATP, a Cy3-labeled P/T DNA (20 nM, Supplementary Figure S1), NeutrAvidin (80 nM homotetramer), and ATP are pre-incubated with an RPA (25 nM heterotrimer, RPA, RPA-OBA-Cy5 or RPA-OBD-Cy5). Then, a PCNA (20 nM homotrimer, Cy5-PCNA or PCNA) is added, the resultant solution is transferred to a fluorometer cell, and the cell is placed in the instrument. I665 and I563 are monitored until both stabilize for at least 1 min. Within this stable region, EFRET values are calculated and averaged to obtain the EFRET value observed prior to addition of RFC•ATP complexes. Finally, pre-formed RFC•ATP complexes (20 nM RFC heteropentamer) are added, the resultant solution is mixed, and I665 and I563 are monitored, beginning 10 s after the addition of the RFC•ATP complexes (i.e. Δt = 10 s).
For pol δ holoenzyme formation and initiation of DNA synthesis experiments, a PCNA (20 nM homotrimer, Cy5-PCNA or PCNA) is first loaded onto ddP/5′TCy3 DNA (20 nM, Supplementary Figure S1) in the presence of an RPA (25 nM heterotrimer, RPA or RPA-OBD-Cy5) and ATP and EFRET is monitored, as described above. Next, pol δ (20–80 nM, heterotetramer, ± dGTP or dCTP) is added, the resultant solution is mixed, and I665 and I563 are monitored, beginning 10 s after the addition of pol δ ± dGTP or dCTP (i.e. Δt = 10 s). I665 and I563 are monitored over time until both stabilize for at least 1 min. Within this stable region, EFRET values are calculated from the observed I665 and I563 and averaged to obtain the EFRET value observed at equilibrium after the addition of pol δ ± dGTP or dCTP. For experiments carried out with RPA-OBD, poly(dT)70 (413 nM) is then added, the resultant solution is mixed, and I665 and I563 are monitored beginning ≤10 s after the addition of poly(dT)70 (i.e. Δt = 10 s).
Results
Strategy to directly and continuously monitor ensemble protein–DNA interactions by FRET
The approach utilizes Cy3, Cy5 FRET pairs each comprised of a Cy3-labeled P/T DNA substrate (Supplementary Figure S1) that mimics a nascent P/T junction on a lagging strand template and a Cy5-labeled protein complex. Each P/T DNA is comprised of a template strand (62-mer) annealed to a 29-mer primer strand that contains a biotin at the 5′ terminus. When pre-bound to NeutrAvidin, the resultant biotin•NeutrAvidin complex prevents loaded PCNA from diffusing off the dsDNA end of the substrate, mimicking the aforementioned ‘protein roadblocks’ (27). The lengths of the dsDNA regions of all P/T DNA substrates are identical (29 bp) and in agreement with the requirements for assembly of a single PCNA onto DNA by RFC (21,27,35). The lengths of the template ssDNA regions (33 nt) adjacent to the P/T junctions accommodate 1 RPA at the RPA:DNA ratios utilized (12–14,36).
For a Cy3, Cy5 FRET pair, the gain and loss of FRET are directly indicated only by simultaneous, anti-correlated changes in the fluorescence emission intensities of the Cy3 donor and Cy5 acceptor. Specifically, appearance of (or increase in) FRET is indicated by a decrease (i.e. quenching) in Cy3 donor fluorescence emission intensity (I563) together with a simultaneous increase in sensitized Cy5 acceptor fluorescence emission intensity (I665). Likewise, disappearance of (or decrease in) FRET is indicated by a decrease in sensitized I665 together with a simultaneous increase (i.e. de-quenching) of I563. Therefore, to directly and continuously monitor changes in ensemble FRET over time, we utilize a spectrofluorometer that monitors I563 and I665 essentially simultaneously (Δt = 0.235 ms) and also permits successive additions of components to a given reaction mixture over time. The approximate FRET efficiency, EFRET, is then calculated for each recorded I563, I665 pair. EFRET values are then plotted as a function of time and fit to kinetic models where appropriate. Importantly, inspection of the time-dependent changes in the I563 and I665 traces from which an EFRET trace is calculated allows direct FRET changes arising from increases/decreases in the distance between the cyanine fluorophores to be distinguished from indirect changes arising from nonspecific effects, such as differential fluorescence sensitization or quenching of both fluorophores, the fluorescence sensitization or quenching of only 1 fluorophore, etc. (37). In this approach, the time between the addition of a given component and the resumption of the respective I recording is less than 10 s (i.e. ‘dead time’ = Δt ≤ 10 s). Thus, while direct FRET changes and the extents to which they occur report on a given protein-DNA interaction, accurate rate constants cannot be obtained when ≥∼90% of the observed FRET change occurs within the deadtime (kobs≥ 0.23 s−1). In the present study, this assay is utilized in pre-steady state kinetic experiments to monitor and directly compare overlapping protein•DNA interactions during assembly of pol δ holoenzymes and initiation of DNA synthesis.
RPA1 OBA remains engaged with template ssDNA downstream of P/T junctions during PCNA loading
During PCNA loading, the interactions of loading complexes with P/T junctions significantly overlap with those of RPA (12–19). To decipher the interplay between these overlapping interactions, we first monitor and directly compare the interactions of loading complexes and RPA1 OBA with P/T junctions. For the former, we utilize a previously characterized Cy3, Cy5 FRET pair (Figure 2A, left) comprised of a Cy3-labeled P/T DNA substrate (5′ddPCy3/T, Supplementary Figure S1) and Cy5-labeled PCNA (Cy5-PCNA). The primer strand of the 5′ddPCy3/T DNA substrate contains a Cy3 donor near the 5′ end and is terminated at the 3′ end with a dideoxycytidine (ddC). A 3′ dideoxy-terminated primer cannot be extended by a DNA polymerase and is implemented here in consideration of subsequent experiments in the present study that utilize human pol δ. When encircling a P/T junction as part of a loading complex (or as loaded PCNA), the Cy5 acceptor on the ‘back face’ of the outer rim of PCNA is oriented towards the Cy3 donor on the 5′ddPCy3/T DNA substrate, yielding a FRET (21). For interactions of RPA1 OBA with P/T junctions, we utilize a FRET pair (Figure 2A, right) comprised of a Cy3-labeled P/T DNA substrate (ddP/5′TCy3, Supplementary Figure S1) and RPA labeled with Cy5 on RPA1 OBA (RPA-OBA-Cy5). ddP/5′TCy3 DNA is identical to the 5′ddPCy3/T DNA substrate described above except that the Cy3 donor is located on the template ssDNA, 30 nt downstream of the P/T junction. The Cy5 on RPA1 OBA faces the Cy3 on ddP/5′TCy3 when RPA-OBA-Cy5 is engaged with the DNA substrate, yielding a FRET (Supplementary Figure S2A–D) (36). RPA-OBA-Cy5 fully supports RFC-catalyzed loading of PCNA onto P/T junctions (Supplementary Table S1). To exclusively monitor protein•DNA interactions during binding and activation of loading complexes at P/T junctions, experiments are performed with an ATP analog [adenosine 5′-O-(3-thio) triphosphate, ATPγS] in which one of the non-bridging oxygens of the γ-phosphoryl group is substituted for sulfur (Figure 2A). Specifically, a Cy3-labeled P/T DNA substrate is first pre-bound with the respective RPA (RPA or RPA-OBA-Cy5) and EFRET is monitored. Next, the respective loading complex pre-formed with ATPγS and PCNA (either PCNA or Cy5-PCNA) is added and the resultant changes in EFRET are monitored. The sulfur substitution within ATPγS severely inhibits ATP hydrolysis by human RFC and consequently stalls the PCNA loading pathway at activation of the loading complex (21,38). Under these conditions, loading complexes rapidly engage the P/T junctions (kobs inc 1 = 2.09 × 108 – 4.21 × 108 M−1s−1), slowly adopt activated conformations (kobs inc 2), and then the PCNA loading pathways stall prior to ATP hydrolysis (21,27,38,39). Thus, all interactions of PCNA with P/T junctions are monitored with PCNA as part of loading complexes and all interactions of RPA1 OBA with P/T junctions are monitored during binding and activation of loading complexes at P/T junctions.
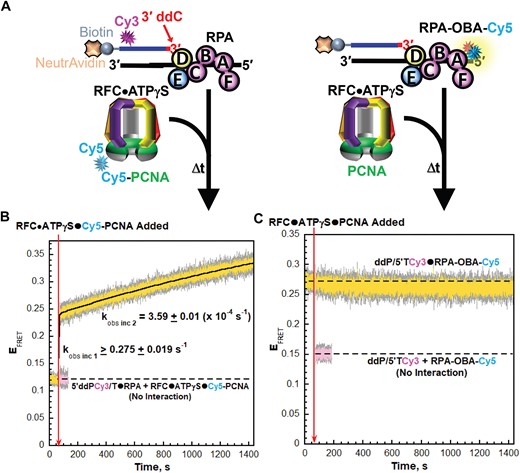
RPA1 OBA interactions with template ssDNA downstream of P/T junctions are maintained during binding and activation of loading complexes. (A) Schematic representations of the FRET pairs and experiments to monitor interactions of; (left) loading complexes with P/T junctions engaged by RPA and; (right) RPA1 OBA with P/T junctions during binding and activation of loading complexes (B, C). FRET data. Each EFRET trace is the mean of at least three independent traces with the S.E.M. shown in grey. The time at which the respective loading complexes are added is indicated by a red arrow. (B) FRET data for interactions of loading complexes with P/T junctions engaged by RPA. The EFRET trace observed after addition of the loading complexes is fit to a double exponential rise and the observed rate constants are reported in the graph. The predicted EFRET trace (pink) for no interaction between 5′ddPCy3/T•RPA complexes and loading complexes is offset to the time after the loading complexes are added and fit to a flat line. (C) FRET data for interactions of RPA1 OBA with P/T junctions during binding and activation of loading complexes. The EFRET trace observed prior to the addition of loading complexes is fit to a flat line that is extrapolated to the axis limits. The predicted EFRET trace (pink) for no interaction between RPA-OBA-Cy5 and ddP/5′TCy3 DNA is offset to the time after loading complexes are added and fit to a flat line.
As observed in Figure 2B for interactions of loading complexes with P/T junctions, the EFRET trace rapidly increases upon addition of the respective loading complex to values significantly above the EFRET trace predicted for no interaction between the Cy5-PCNA•RFC•ATPγS complexes and the 5′ddPCy3/T DNA•RPA complexes. The EFRET increase is comprised of two phases, as expected, with an observed rate constant for the second phase of kobs inc 2 = 3.59 ± 0.01 (×10−4 s−1). As predicted based on the reported values of kobs inc 1 discussed above, the majority of this phase occurs within the dead time and, hence, an accurate kinetic rate constant cannot be obtained due to relatively few time points within its lifetime. The direct FRET changes observed in the present study agree with that observed in a previous, pre-steady state kinetic study that analyzed PCNA loading under similar conditions with ATPγS by monitoring sensitized Cy5 fluorescence emission intensities via stopped flow (21). For interactions of RPA1 OBA with P/T junctions, a significant constant EFRET is observed prior to the addition of the respective loading complex (Figure 2C) due to the stable interaction of RPA-OBA-Cy5 with the ddP/5′TCy3 DNA (Supplementary Figure S2A–D). Upon addition of the respective loading complex, the EFRET trace is maintained at the significantly elevated level above the EFRET trace predicted for no interaction between RPA-OBA-Cy5 and ddP/5′TCy3 DNA (Figure 2C). Directly comparing these results to those presented in Figure 2B reveals that interactions of RPA1 OBA with template ssDNA downstream of P/T junctions are maintained during binding and activation of loading complexes.
Next, we performed similar assays with these FRET pairs utilizing ATP to monitor the remainder of the PCNA loading pathway (Figure 3A). A Cy3-labeled P/T DNA substrate is first pre-bound with the respective RPA (RPA or RPA-OBA-Cy5) and then the respective PCNA (PCNA or Cy5-PCNA) is added and EFRET is monitored over time. Finally, pre-formed RFC•ATP complexes are added, and EFRET is monitored. Under these conditions, RFC•ATP complexes must first bind ‘free’ PCNA in order for loading to proceed and, consequently, PCNA loading is biphasic. First, all steps up to and including release of the closed PCNA clamps on the P/T junctions are rate-limited by a kinetic step (kobs inc 1) along the pathway that occurs prior to and much slower than binding of the loading complexes to P/T junctions. Second, the loaded PCNA repositions relatively slowly (kobs inc 2) on the P/T junctions concurrent with release of RFC•ADP complexes into solution via their dissociation from the resident RPA (27). Thus, for experiments with Cy5-PCNA, EFRET reports on interactions of loaded PCNA with P/T junctions, which are influenced by the release of RFC•ADP complexes into solution. For experiments with RPA-OBA-Cy5, EFRET reports on the interactions of RPA1 OBA with P/T junctions during release of RFC•ADP complexes into solution.
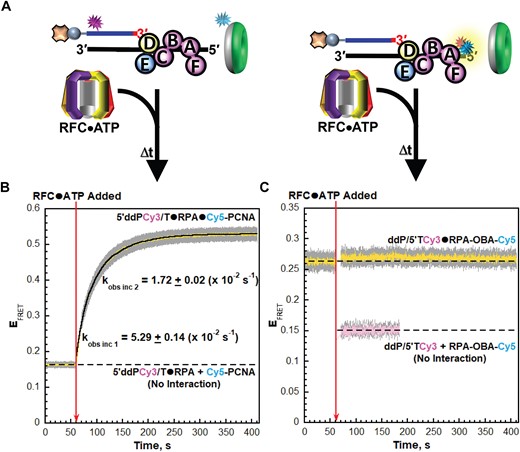
RPA1 OBA interactions with template ssDNA downstream of P/T junctions are maintained as RFC•ADP complexes engages and release from RPA. (A) Schematic representation of the FRET pairs and experiments to monitor interactions of; (left) loaded PCNA and RFC•ADP with P/T junctions engaged by RPA and; (right) RPA1 OBA interactions with P/T junctions engaged by loaded PCNA and RFC•ADP. (B, C) FRET data. Each EFRET trace is the mean of at least three independent traces with the S.E.M. shown in grey. The time at which RFC•ATP complexes are added is indicated by a red arrow. (B) FRET data for interactions loaded PCNA and RFC•ADP with P/T junctions engaged by RPA. The EFRET trace observed prior to the addition of RFC•ATP complexes represents the complete absence of interactions between the 5′ddPCy3/T•RPA complexes and Cy5-PCNA and is fit to a flat line that is extrapolated to the axis limits. The EFRET trace observed after the addition of RFC•ATP complexes is fit to a double exponential rise and the observed rate constants are reported in the graph and Supplementary Table S1. (C) FRET data for interactions of RPA1 OBA with P/T junctions engaged by loaded PCNA and RFC•ADP. The EFRET trace observed prior to the addition of the RFC•ATP complexes is fit to a flat line that is extrapolated to the axis limits. The predicted EFRET trace (pink) for no interaction between RPA-OBA-Cy5 and ddP/5′TCy3 DNA is offset to the time after RFC•ATP complexes are added and fit to a flat line.
For interactions of PCNA with P/T junctions, a low, constant EFRET is observed prior to the addition of RFC•ATP complexes due to the presence of both the Cy3 donor and the Cy5 acceptor (Figure 3B). EFRET values observed during this period are a true experimental baseline representing the absence of any interactions between Cy5-PCNA and the 5′ddPCy3/T DNA•RPA complexes. Upon addition of RFC•ATP complexes, the EFRET trace rapidly increases to values significantly above the EFRET trace observed for no interaction between Cy5-PCNA and the 5′ddPCy3/T•RPA complexes (Figure 3B). The rapid increase in EFRET is biphasic, as expected, with observed rate constants of kobs inc 1 = 5.29 ± 0.14 (×10−2) s−1 and kobs inc 2 = 1.72 ± 0.02 (×10–2) s−1 (Supplementary Table S1). kobs inc 2 agrees very well with the values reported in a previous, pre-steady state study that analyzed PCNA loading under similar conditions by monitoring sensitized Cy5 fluorescence emission intensities via stopped flow. kobs inc 1 observed in the present study is ∼2-fold slower than that reported in the previous studies and this deviation is due to small differences in the buffers utilized (Supplementary Information, Supplementary Figure S5 and Supplementary Table S1). For interactions of RPA1 OBA with P/T junctions, a significant constant EFRET is observed prior to the addition of RFC•ATP complexes (Figure 3C). Upon addition of RFC•ATP complexes, the EFRET trace is maintained at the significantly elevated level above the EFRET traces predicted for no interaction between ddP/5′TCy3 DNA and RPA-OBA-Cy5. Directly comparing these results to those presented in Figure 3B reveals that interactions of RPA1 OBA with template ssDNA downstream of P/T junctions are maintained as loaded PCNA repositions on the P/T DNA and RFC•ADP complexes engage and dissociate from the resident RPA. Together, the results presented in Figures 2 and 3 reveal that RPA1 OBA remains engaged with template ssDNA downstream of P/T junctions throughout loading of the resident PCNA. Thus, RPA complexes remain on P/T DNA throughout PCNA loading, in agreement with observations from previous studies (27,40). Next, we repeated the assays presented in Figures 2 and 3 with different Cy3, Cy5 FRET pairs to monitor and directly compare the interaction(s) of RPA2 OBD and loading complexes with P/T junctions during PCNA loading.
RPA2 OBD transiently releases from P/T junctions during PCNA loading
To establish and monitor interactions of loading complexes with P/T junctions during PCNA loading, we utilized the aforementioned Cy5-PCNA and a Cy3-labeled P/T DNA substrate (5′PCy3/T, Supplementary Figure S1) in which the primer strand contains a Cy3 donor near the 5′ end; the 3′ terminus of the primer is not modified (Figure 4A, left). For interactions of RPA2 OBD with P/T junctions (Figure 4A, right) we utilize a Cy3-labeled P/T DNA substrate (3′PCy3/T, Supplementary Figure S1) and RPA labeled with Cy5 on RPA2 OBD (RPA-OBD-Cy5). The 3′PCy3/T DNA substrate is identical to the 5′PCy3/T DNA substrate described above except that the Cy3 donor is located on the 3′ terminus of the primer strand, directly at the P/T junction. The Cy5 on RPA2 OBD faces the Cy3 on the 3′PCy3/T DNA substrate when RPA-OBD-Cy5 is engaged, yielding a FRET (Supplementary Figure S2E–S2H) (24). RFC-catalyzed loading of PCNA onto P/T junctions is fully supported by RPA-OBD-Cy5 (Supplementary Table S1) and the 3′PCy3/T DNA substrate as well (Supplementary Information, Supplementary Table S1 and Supplementary Figure S6).
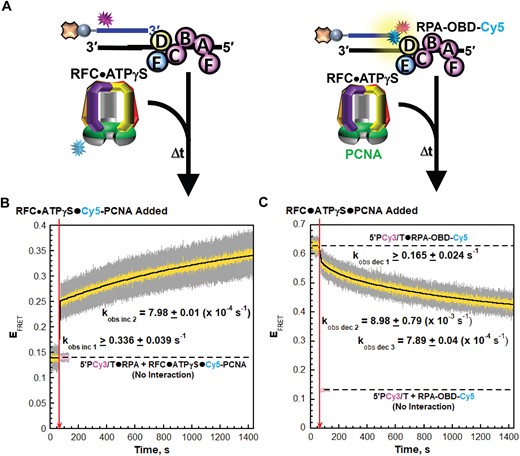
RPA2 OBD releases from P/T junctions during binding and activation of loading complexes. (A) Schematic representations of the FRET pairs and experiments to monitor interactions of; (left) loading complexes with P/T junctions engaged by RPA and; (right) RPA2 OBD with P/T junctions during binding and activation of loading complexes. (B, C) FRET data. Each EFRET trace is the mean of at least three independent traces with the S.E.M. shown in grey. The time at which the respective loading complexes are added is indicated by a red arrow. (B) FRET data for interactions of loading complexes with P/T junctions engaged by RPA. The EFRET trace observed after addition of the respective loading complexes is fit to a double exponential rise and the observed rate constants are reported in the graph. The predicted EFRET trace (pink) for no interaction between 5P′Cy3/T•RPA complexes and the loading complexes is offset to the time after the loading complexes are added and fit to a flat line. (C) FRET data for interactions of RPA2 OBD with P/T junctions during binding and activation of loading complexes. The EFRET trace observed prior to addition of the respective loading complexes is fit to a flat line that is extrapolated to the axis limits. The predicted EFRET trace (pink) for no interaction between RPA-OBD-Cy5 and the P/5′TCy3 DNA is offset to the time after loading complexes are added and fit to a flat line.
First, we perform PCNA loading experiments in the presence of ATPγS (Figure 4A). As expected, the changes in EFRET observed in Figure 4B upon addition of the respective loading complexes are nearly identical to those presented in Figure 2B for the 5′ddPCy3/T DNA substrate. For interactions of RPA2 OBD with P/T junctions, a significant constant EFRET is observed prior to the addition of the respective loading complex (Figure 4C) due to the stable interaction of RPA-OBD-Cy5 with 3′PCy3/T DNA (Supplementary Figure S2E–S2H). Upon addition of the respective loading complexes, EFRET decreases over time but remains significantly elevated above the predicted EFRET trace for no interaction between RPA-OBD-Cy5 and the P/5′TCy3 DNA substrate. Inspection of the time dependent changes in I563 and I665 traces from which the EFRET trace is calculated indicates that the EFRET decrease observed in Figure 4C is direct and results from an increase in the distance between the Cy3 on the 3′PCy3/T DNA substrate and the Cy5 on RPA-OBD-Cy5 (Supplementary Figure S7).
The EFRET decrease observed in Figure 4C upon addition of the respective loading complexes is comprised of at least three phases; kobs dec 1, kobs dec 2 = 8.98 ± 0.79 (×10−3 s−1) and kobs dec 3 = 7.89 ± 0.04 (×10−4 s−1). kobs inc 1 cannot be accurately fit due to relatively few time points within its lifetime. Interestingly, the EFRET changes observed in Figures 4C are anti-correlated to the EFRET changes observed in Figure 4B and occur with very similar kinetics. In particular, kobs dec 3 for the EFRET decrease observed in Figure 4C, which accounts for 67.4 ± 2.50% of the observed decrease, is nearly identical to kobs inc 2 for the EFRET increase observed in Figure 4B. Altogether, these results reveal that RPA2 OBD releases from the 3′ termini of primer strands and the template ssDNA immediately adjacent to P/T junctions during binding and activation of loading complexes. Next, we utilize these FRET pairs in PCNA loading assays with ATP (Figure 5A) to monitor the remainder of the PCNA loading pathway. These assays are identical to those presented above in Figure 3A.
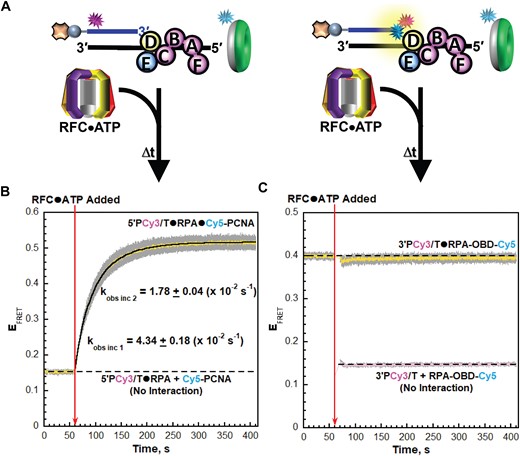
RPA2 OBD interactions with P/T junctions are re-established prior to and much faster than release of RFC•ADP complexes. (A) Schematic representation of the FRET pairs and experiments to monitor interactions of; (left) loaded PCNA and RFC•ADP complexes with P/T junctions engaged by RPA and; (right) RPA2 OBD interactions with P/T junctions engaged by loaded PCNA and RFC•ADP complexes. (B, C) FRET data. Each EFRET trace is the mean of at least three independent traces with the S.E.M. shown in grey. The time at which the RFC•ATP complexes are added is indicated by a red arrow. (B) FRET data for interactions of loaded PCNA and RFC•ADP complexes with P/T junctions engaged by RPA. The EFRET trace observed prior to the addition of RFC•ATP complexes represents the complete absence of interactions between 5′PCy3/T•RPA complexes and Cy5-PCNA and is fit to flat line that is extrapolated to the axis limits. The EFRET trace observed after the addition of RFC•ATP complexes is fit to a double exponential rise and the observed rate constants are reported in the graph and Supplementary Table S1. (C) FRET data for interactions of RPA2 OBD with P/T junctions engaged by loaded PCNA and RFC•ADP complexes. The EFRET trace observed prior to the addition of RFC•ATP complexes is fit to a flat line that is extrapolated to the axis limits. The predicted EFRET trace (pink) for no interaction between RPA-OBD-Cy5 and the 3′PCy3/T DNA is offset to the time after RFC•ATP complexes are added and fit to a flat line.
For interactions of PCNA with P/T junctions, a low, constant EFRET is observed prior to the addition of RFC•ATP complexes (Figure 5B). Again, EFRET values observed during this period are a true experimental baseline representing the absence of any interactions between Cy5-PCNA and the 5′PCy3/T DNA•RPA complexes. Upon addition of RFC•ATP complexes, the EFRET trace rapidly increases to values significantly above the EFRET values observed for no interaction between Cy5-PCNA and the 5′PCy3/T•RPA complexes (Figure 5B). As expected, the rapid increase in EFRET is biphasic with kobs inc 1 = 4.34 ± 0.18 (×10−2) s−1 and kobs inc 2 = 1.78 ± 0.04 (×10−2) s−1 (Supplementary Table S1). Each of the observed rate constants agrees very well with the values reported in Figure 3B above. For interactions of RPA2 OBD with P/T junctions, a significant, constant EFRET is observed prior to the addition of RFC•ATP complexes (Figure 5C). Under the conditions of the assay, kinetic steps along the PCNA loading pathway up to and including release of the closed PCNA clamp on DNA are kinetically invisible. Only release of the RFC•ADP complexes into solution is visible and this step immediately follows ATP hydrolysis by RFC within the activated loading complexes (and concomitant closure of the sliding clamp rings) or release of the closed PCNA clamps from the RFC•ADP complexes (27). The results presented in Figure 4C indicate that RPA2 OBD releases from the 3′ termini of primer strands and the template ssDNA immediately adjacent to P/T junctions during binding and activation of loading complexes. This raises at least three scenarios for the EFRET trace observed in Figure 5C after the addition of RFC•ATP complexes. First, if the relinquished RPA2 OBD interactions are not subsequently re-established following release of RFC•ADP complexes into solution, EFRET will instantaneously decrease upon addition of RFC•ATP complexes and then remain at a reduced level for the remainder of the time course. Second, if the relinquished RPA2 OBD interactions are re-established prior to and much faster than release of RFC•ADP complexes into solution, then changes in EFRET will not be observed upon addition of RFC•ATP complexes. Third, if the relinquished RPA2 OBD interactions are re-established simultaneous with or subsequent to release of RFC•ADP complexes into solution, then EFRET will instantaneously decrease upon addition of RFC•ATP complexes and then increase over time to the EFRET values observed prior to the addition of RFC•ATP complexes. As observed in Figure 5C, the EFRET trace observed prior to the addition of RFC•ATP complexes is maintained at the significantly elevated level above the EFRET trace predicted for no interactions between 3′PCy3/T DNA and RPA-OBD-Cy5. This agrees with the second scenario described above, indicating that the relinquished interactions of RPA2 OBD with the 3′ termini of primer strands and the template ssDNA immediately adjacent to P/T junctions are re-established prior to and much faster than release of RFC•ADP complexes into solution. Together, the results presented in Figures 4 and 5 reveal that RPA2 OBD transiently releases from the 3′ termini of primer strands and the template ssDNA immediately adjacent to P/T junctions to accommodate binding and activation of loading complexes during loading of the resident PCNA.
RPA remains engaged with P/T junctions during formation of pol δ holoenzymes and initiation of DNA synthesis
In the next steps of human pol δ holoenzyme assembly and initiation of DNA synthesis, pol δ engages the ‘front face’ of loaded PCNA, forming a holoenzyme, engages the P/T junction, and aligns an incoming, complimentary dNTP at the 3′ terminus of the primer strand to initiate DNA synthesis (Figure 1). During the latter, the interactions of pol δ with P/T junctions significantly overlap with those of RPA (12–18,31,32). To investigate the interplay between these overlapping interactions, we first had to distinguish and isolate pol δ holoenzymes that are disengaged from P/T junctions from those that have initiated DNA synthesis. To do so, we design a FRET assay (depicted in Figure 6A) based on the dynamics of loaded PCNA and pol δ•PCNA interactions, as follows. Loaded PCNA rapidly and randomly diffuses along the dsDNA regions of P/T junctions (27,41). These diffusive movements along the 29 bp (∼100 Å) dsDNA regions of the P/T DNA substrates in the present study are kinetically invisible. Thus, the constant EFRET values observed at the plateau of PCNA loading assays performed with ATP (Figures 3B and 5B) report on the average distance between loaded Cy5-PCNA and the 5′ terminus of the Cy3-labeled primer strand. Pol δ has significantly high affinity for PCNA encircling P/T junctions (KD < 10 nM) but dramatically low affinity, if any, for P/T junctions in the absence of dNTPs (22,27,31,42,43). Thus, in the absence of dNTPs, interaction of pol δ with loaded PCNA does not tether the engaged clamp to a defined region of the dsDNA region of a P/T junction. Accordingly, the constant EFRET values observed at the plateau of PCNA loading assays performed with ATP are expected to persist upon the subsequent addition of pol δ alone. In the initiation state for DNA synthesis, pol δ (as part of a holoenzyme) engages the P/T junction, occupying ∼10 bp of the dsDNA region immediately upstream of the P/T junction (27,31,32). In the most populated structures of the initiation state (∼65%), pol δ tethers the engaged PCNA ∼34 Å closer to the 5′ terminus of the primer strand in essentially a vertical orientation relative to the dsDNA (32). In the remaining populations (∼35%), the orientation of the engaged PCNA varies in range relative to the dsDNA by up to ∼20o. Such tilting orients the outer rim of the engaged PCNA an additional ∼30 Å closer to 5′ terminus of the primer strand. Thus, adoption of the initiation state for DNA synthesis decreases the distance between loaded PCNA and the 5′ terminus of the primer strand. Accordingly, we reason that the constant EFRET values observed at the plateau of PCNA loading assays performed with ATP increase further upon adoption of the initiation state for DNA synthesis.
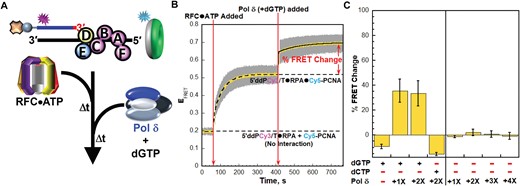
Adoption of the initiation states of pol δ holoenzymes tethers the engaged PCNA closer to the 5′ end of primer strands. (A) Schematic representation of the FRET pair and experiment to monitor interactions of loaded PCNA with P/T junctions during formation of pol δ holoenzymes and initiation of DNA synthesis (B) FRET data for experiments performed with dGTP. Each EFRET trace is the mean of at least three independent traces with the S.E.M. shown in grey. The times at which the RFC•ATP complexes and Pol δ (+ dGTP) are added are indicated by red arrows. The EFRET trace observed prior to the addition of RFC•ATP complexes is fit to a dashed flat line that is extrapolated to the axis limits to depict the average EFRET value for no interaction between Cy5-PCNA and the 5′ddPCy3/T•RPA complexes. The EFRET trace observed after the addition of RFC•ATP complexes is fit to a dashed double exponential rise that is extrapolated to the axis limits to depict the average EFRET value for complete loading of Cy5-PCNA onto the 5′ddPCy3/T•RPA•Cy5-PCNA complexes. The EFRET traces observed after the addition of Pol δ (+ dGTP) is fit to a double exponential rise and the % FRET is depicted in red. (C) Characterization of the observed % FRET change. FRET experiments were repeated in the presence/absence of a dNTP (either dGTP or dCTP) and with varying concentrations of Pol δ (0–100 nM heterotetramer) and the % FRET change observed upon the ultimate addition was measured. Each column is the mean of at least three independent replicates with the S.E.M. shown in black.
For the assay depicted in Figure 6A, Cy5-PCNA is first loaded onto the 5′ddPCy3/T DNA substrate in the presence of ATP exactly as described in left panel of Figure 3A. A low, constant EFRET is observed prior to the addition of RFC•ATP, representing a true experimental baseline signal (Figure 6B). As expected, the EFRET trace rapidly increases upon addition of RFC•ATP complexes to values significantly above the EFRET trace observed for no interaction between Cy5-PCNA and 5′ddPCy3/T•RPA complexes (Figure 6B). At the plateau, a PCNA is loaded onto each P/T junction and stabilized on the duplex region. Next, dGTP is added together with pol δ at a stoichiometric ratio of pol δ to DNA and PCNA (i.e. DNA:PCNA:pol δ = 1:1:1). Under these conditions, pol δ engages PCNA encircling the P/T junction with a rapid bimolecular rate constant and adopts the initiation state for DNA synthesis where pol δ engages loaded PCNA, the P/T junction, and aligns dGTP at the 3′ terminus of the primer strand in a complimentary bp with the template nucleotide (a Cytosine) immediately 5′ of the P/T junction (27,31,32). Both extension of the primer (via pol δ DNA polymerase activity) and degradation of the primer (via pol δ exonuclease activity) are prohibited due to the 3′ dideoxy-terminated primer and the use of exonuclease-deficient pol δ (27). Upon the simultaneous addition of pol δ and dGTP, the EFRET trace increases further and plateaus at values significantly above the EFRET values observed for stoichiometric loading of PCNA. Inspection of the time dependent changes in I563 and I665 traces from which the EFRET trace is calculated indicates that the further increase in EFRET observed in Figure 6B is direct and results from a further reduction in the distance between the Cy3 donor near the 5′ end of the primer and the Cy5 acceptor on the ‘back face’ of the outer rim of loaded Cy5-PCNA (Supplementary Figure S8). The new plateau in EFRET observed upon the simultaneous addition of pol δ and dGTP is referred to herein as the enhanced FRET state and is highlighted in Figure 6B by the ‘% FRET Change’. The appearance of the enhanced FRET state is comprised of at least two phases; a relatively fast phase (kobs inc 1) that is too rapid to accurately fit and a relatively slower phase (kobs inc 2) with an observed rate constant of 8.41 ± 0.19 (×10−3 s−1). Given that most of kobs inc 1 occurs within the dead time of the experiment, this phase likely represents that the rapid biomolecular rate constant for the association of pol δ with PCNA encircling the P/T junctions. The results presented in Figure 6B agree with the expectations described above and suggest that adoption of the initiation state for DNA synthesis significantly decreases the distance between loaded PCNA and the 5′ end of the primer strand. To confirm this, we characterize the enhanced FRET state by repeating these experiments under various conditions and monitoring the % FRET change.
For reference, the % FRET change observed in Figure 6B is reported in Figure 6C (+dGTP, –dCTP, 1× pol δ). Doubling the concentration of pol δ in the presence of dGTP (+dGTP, –dCTP, 2× pol δ) does not affect the % FRET change, indicating that the enhanced FRET state is saturated at stoichiometric pol δ. Next, dGTP is omitted. Under these conditions, pol δ saturates loaded PCNA to form pol δ holoenzymes but engages P/T junctions with dramatically low affinity, if at all (21,22,31,42–44). Inclusion of only pol δ (–dGTP, –dCTP, 1× pol δ) does not yield a % FRET change and this behavior persists at increasing concentrations of pol δ (up to a DNA:PCNA:pol δ ratio of 1:1:4). These results agree with the expectations described above and indicate that the enhanced FRET state requires dGTP. Furthermore, these results reveal that pol δ alone does not displace RPA from a P/T junction upon engaging the resident loaded PCNA, as follows. A large protein, such as pol δ, simply binding to loaded PCNA decreases its diffusion constant along dsDNA only 2.1-fold (41). Thus, in the absence of dNTPs, if pol δ displaces RPA from the 5′ddPCy3/T DNA upon engaging loaded Cy5-PCNA, the resultant Cy5-PCNA•pol δ complex would rapidly diffuse off the ssDNA end of the 5′ddPCy3/T DNA simultaneously with RPA displacement. This would lead to a rapid decrease in EFRET (rate-limited by displacement of RPA) and a subsequent, relatively slow increase in EFRET as free Cy5-PCNA is reloaded by RFC. However, a % FRET change is not observed in Figure 6C when only pol δ is included, indicating that loaded PCNA persists on P/T junctions upon pol δ holoenzyme formation and, hence, RPA remains engaged with P/T junctions throughout pol δ holoenzyme formation. This is directly investigated and confirmed in Figure 7. A slight, negative % FRET change is observed when only dGTP (+dGTP, –dCTP, –pol δ) is included and also when pol δ and an incorrect dNTP (dCTP) are included (–dGTP, +dCTP, 2× pol δ). The observed changes in the EFRET values from which the % FRET changes are calculated for these conditions are direct (Supplementary Figure S9 and S10) and result from dNTP inhibition of PCNA re-loading by RFC (Supplementary Information and Supplementary Figure S11) (45). Altogether, the results presented in Figure 6C indicate that formation of the enhanced FRET state requires both the correct dNTP (dGTP) and pol δ. Hence, the enhanced FRET state represents the initiation states for DNA synthesis. Furthermore, these results confirm that adoption of the initiation states for DNA synthesis significantly decreases the distance between loaded PCNA and the 5′ terminus of the primer strand. Next, we utilize the conditions highlighted in Figure 6 above and the RPA-OBA-Cy5, Cy3 DNA FRET pair to investigate the interactions of RPA with P/T junctions during formation of pol δ holoenzymes and initiation of DNA synthesis.
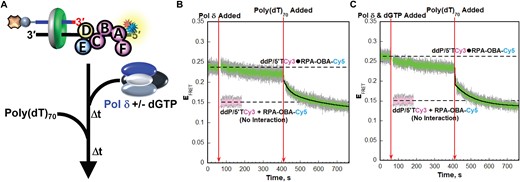
RPA remains engaged with P/T junctions throughout formation of the resident pol δ holoenzymes and initiation of DNA synthesis. (A) Schematic representation of the FRET pair and experiment to monitor interactions of RPA-OBA with P/T junctions during formation of pol δ holoenzymes and initiation of DNA synthesis. (B, C) FRET data. Each EFRET trace is the mean of at least three independent traces with the S.E.M. shown in grey. The times at which the Pol δ (±dGTP) and poly(dT)70 are added are indicated by red arrows. The EFRET trace observed prior to the addition of the Pol δ (±dGTP) is fit to a dashed flat line that is extrapolated to the axis limits to depict the average EFRET value for the interaction between RPA-OBA-Cy5 and ddP/5′TCy3. The predicted EFRET trace (pink) for no interaction between RPA-OBA-Cy5 and the ddP/5′TCy3 DNA is fit to a flat line that is extrapolated to the axis limits. The EFRET trace observed after the addition of the poly(dT)70 is fit to a double exponential decline. Shown in panels B and C are the results for experiments carried out in the absence and presence of dGTP, respectively.
For the assays depicted in Figure 7A, the ddP/5′TCy3 DNA substrate is pre-bound with RPA-OBA-Cy5 and PCNA is loaded by RFC in the presence of ATP exactly as described in the right panel of Figure 3A. After completion of RFC-catalyzed loading of PCNA, pol δ is added in the absence of dGTP or simultaneously with dGTP and is present at a 2-fold excess to DNA and PCNA to ensure all loaded PCNA is engaged by pol δ. Finally, to demonstrate RPA occupancy of P/T junctions following the addition of pol δ, a large excess of poly(dT)70 ssDNA is added to release RPA-OBA-Cy5 from the ddP/5′TCy3 DNA substrates (via facilitated exchange) and prohibit re-binding (Supplementary Information and Supplementary Figure S12) (23,24,46,47). Prior to the addition of pol δ, a significant, constant EFRET is observed (Figure 7B). Upon addition of pol δ alone, the EFRET trace appears to slowly and minimally decrease over time but remains within experimental error of the EFRET values observed prior to the addition of pol δ and significantly elevated above the EFRET trace predicted for no interaction between ddP/5′TCy3 DNA and RPA-OBD-Cy5. Inspection of the time dependent changes in the I563 and I665 traces from which the EFRET trace is calculated indicates that the apparent decrease in EFRET is indirect and due to nonspecific effects (Supplementary Figure S13A–C). This suggests that formation of pol δ holoenzymes at P/T junctions does not alter the distances between the Cy3 FRET donors on the ddP/5′TCy3 DNA substrates and the Cy5 FRET acceptors on the engaged RPA-OBA-Cy5 complexes. Upon addition of excess poly(dT)70, EFRET rapidly decreases over time to the EFRET trace predicted for no interaction between ddP/5′TCy3 DNA and RPA-OBA-Cy5, indicating complete release of RPA-OBA-Cy5 from ddP/5′TCy3 DNA. Together, this suggests that nearly all (≥85.1 ± 11.6%, based on the observed EFRET changes), if not all, RPA remains engaged with P/T junctions throughout formation of the resident pol δ holoenzymes and this is due, at least in part, to persistent interactions of RPA1 OBA with the template ssDNA downstream of the P/T junctions. This agrees with the results discussed above for Figure 6C.
Next, we repeated the experiments described in Figure 7A by adding dGTP simultaneously with a 2-fold excess of pol δ compared to DNA and PCNA. Here, excess DNA polymerase ensures all loaded PCNA is engaged in pol δ holoenzymes that have adopted initiation states for DNA synthesis (Figure 6). Prior to the addition of pol δ and dGTP, a significant, constant EFRET is observed (Figure 7C). Upon addition of pol δ and dGTP, the EFRET trace appears to slowly and minimally decrease but remains within experimental error of the EFRET values observed prior to the addition of pol δ and dGTP and significantly elevated above the EFRET trace predicted for no interaction between ddP/5′TCy3 DNA and RPA-OBD-Cy5. Inspection of the time dependent changes in the I563 and I665 traces from which the EFRET trace is calculated indicates that the apparent decrease in EFRET is indirect and due to nonspecific effects (Supplementary Figure S13D–F). This suggests that adoption of initiation states by pol δ holoenzymes does not alter the distances between the Cy3 FRET donors on the ddP/5′TCy3 DNA substrates and the Cy5 FRET acceptors on the engaged RPA-OBA-Cy5 complexes. Upon addition of poly(dT)70, EFRET rapidly decreases over time to the EFRET trace predicted for no interaction between ddP/5′TCy3 DNA and RPA-OBA-Cy5, indicating complete release of RPA-OBA-Cy5 from ddP/5′TCy3. Together, this indicates that nearly all (≥77.3 ± 5.7% based on the observed changes in EFRET), if not all, RPA remains engaged with P/T junctions during initiation of DNA synthesis by the resident pol δ holoenzymes and this is due, at least in part, to persistent interactions of RPA1 OBA with the template ssDNA downstream of the P/T junctions.
Discussion
During assembly of a pol δ holoenzyme and/or initiation of DNA synthesis, RPA, RFC and pol δ all directly engage the 3′ terminus of the primer strand and at least ∼6 nt of the template ssDNA immediately downstream of a P/T junction (12–19,31,32). In the present study, we design and utilize novel, ensemble FRET assays to investigate how the mutual exclusivity of these overlapping protein−DNA interactions are accommodated during this process. Results from the present study reveal that the conformation of the RPA•DNA complex dynamically re-organizes to remain engaged with the template ssDNA and vacate the P/T junction to RFC and pol δ (Figure 8). These results highlight and distinguish the functional consequences of dynamic RPA•DNA interactions during pol δ holoenzyme assembly, initiation of DNA synthesis, and other RPA-dependent DNA metabolic processes, as discussed below.
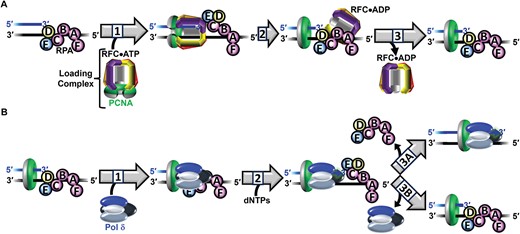
Stepwise assembly of the human Pol δ holoenzyme and initiation of DNA synthesis. (A) PCNA loading. 1) Binding and activation of a loading complex at a P/T junction. 2) Hydrolysis of ATP by RFC (within activated loading complex). 3) Release of RFC•ADP into solution. (B) Initiation of DNA synthesis. 1) Formation of a pol δ holoenzyme. 2) Initiation of DNA synthesis. 3A) Processive dNTP incorporation. 3B) Dissociation of pol δ following initial dNTP incorporation.
RFC and RPA2 OBD reversibly exchange at a P/T junction during PCNA loading
The ssDNA immediately adjacent to a P/T junction is initially engaged by an RPA heterotrimer in an orientation-specific manner. Specifically, the ssDNA is engaged with a defined 5′→3′ polarity of the RPA OBs (depicted in Figure 1) and OBD and OBC directly contact the 3′ terminus of the primer strand and/or the template ssDNA immediately adjacent to the P/T junction (12–19). During PCNA loading, a loading complex binds a P/T junction in a high affinity state, and upon activation, RFC shelters the 3′ terminus of the primer strand and 12 nt of the template ssDNA immediately downstream of the P/T junction (20,31). In the present study, results from novel, ensemble FRET assays (Figures 2–5) reveal how the significant overlap of these protein•DNA interactions is accommodated during PCNA loading (Figure 8A). RPA2 OBD releases from the 3′ terminus of the primer strand and the template ssDNA immediately adjacent to the P/T junction to permit binding and activation of a loading complex while RPA1 OBA remains engaged with the template ssDNA further downstream of the P/T junction, distal to the activated loading complex ssDNA footprint (Figure 8A, Step 1). Based on the time-dependent change in EFRET observed in Figure 4C, it is estimated that the average distance between RPA2 OBD and the 3′ terminus of the primer strand increases by 18.9 ± 2.0 Å during binding and activation of a loading complex at a P/T junction (Supplementary Information, Supplementary Table S2) (48). Furthermore, given the ssDNA footprint of the activated loading complex discussed above, it is likely that other RPA OBs also release from the template ssDNA immediately adjacent to the P/T junction (31). For P/T DNA substrates in the present study, the template ssDNA is 33 nt in length. Accordingly, binding and activation of a loading complex at a P/T junction leaves 21 nt of ssDNA at the 5′ end of the template strand for at least RPA1 OBA to remain engaged with. However, other RPA OBs are likely to remain engaged as well as this ssDNA length is sufficient for both OBA and OBB of the RPA1 subunit to engage the RPA complex in a tight interaction (KD < 5 nM) (49). The relinquished interactions of RPA2 OBD (and likely other OBs) are re-established after ATP hydrolysis by RFC within the activated loading complex (Figure 8A, Step 2) and prior to release of the RFC•ADP complex into solution via its dissociation from the resident RPA (Figure 8A, Step 3). Thus, the present study reveals that RPA remains engaged with a P/T junction throughout loading of the resident PCNA and does so by dynamically re-organizing to accommodate binding and activation of a loading complex at a P/T junction. This confirms the model proposed from previous indirect studies that suggested that RPA does not dissociate from P/T junctions during loading of the resident PCNA (27,40).
The contacts of RPA2 OBD with a P/T junction may be released during PCNA loading by at least two pathways. First, a loading complex may actively displace RPA2 OBD from the 3′ terminus of the primer strand and the template ssDNA immediately adjacent to the P/T junction. Alternatively, given the dynamics of RPA•ssDNA interactions revealed by recent studies, a loading complex may passively capture a P/T junction that has been transiently vacated by RPA2 OBD. The human RPA complex engages ssDNA with exceptionally high affinity but exists in microscopically dissociated states due to the dynamics of its individual OB folds (46,47,50). In particular, RPA2 OBD has been directly observed to continuously dissociate from and rapidly re-bind to ssDNA while the RPA complex remains engaged with the ssDNA through other OBs (23). These continuous microscopic dissociation events within an RPA complex permit free ssDNA-binding proteins to gain access to ssDNA (46,50). Considering this, we propose that a loading complex captures a P/T junction that is exposed during microscopic dissociation of at least RPA2 OBD from the 3′ terminus of the primer strand and the template ssDNA immediately adjacent to the P/T junction. This will be validated in future studies.
The transient dissociation of RPA2 OBD during PCNA loading that is revealed in the present study highlights and distinguishes the functional consequences of dynamic RPA•DNA interactions during disparate DNA metabolic process. For example, in the repair of dsDNA breaks via homologous recombination, RPA must be exchanged for another high-affinity ssDNA-binding protein, the Rad51 recombinase, along the entire length of the ssDNA. During this process, microscopic dissociations of OBs within an RPA complex expose small sections of the underlying ssDNA, allowing Rad51 to engage the ssDNA in a tight interaction (KD ∼ 200 nM) (51). These initial microscopic dissociation events of RPA subsequently manifest to its macroscopic dissociation through mass action of Rad51, which indiscriminately nucleates and polymerizes to filaments that encircle the length of the ssDNA. Here, dynamic RPA•DNA interactions permit exchange of RPA for Rad51, which is required for homologous recombination. During PCNA loading, release of at least RPA2 OBD from the 3′ terminus of the primer strand and the template ssDNA immediately adjacent to the P/T junction permits binding of a single loading complex in a high affinity state and its subsequent activation at the P/T junction (Figure 8A, Step 1) (20). However, these microscopic dissociation events do not manifest to macroscopic dissociation of RPA as additional loading complexes do not engage the remaining template ssDNA to displace RPA. Rather, the RPA complex remains engaged with P/T DNA throughout the entire PCNA loading pathway via interactions of at least RPA1 OBA with the template ssDNA downstream of the P/T junction, distal to the activated loading complex ssDNA footrprint. Furthermore, the relinquished interactions of RPA2 OBD (and likely other OBs) are re-established with a P/T junction after ATP hydrolysis by RFC within the activated loading complex (Figure 8A, Step 2), which converts the clamp loader to a low affinity DNA-binding state. RFC•ADP then vacates the P/T junction and subsequently releases into solution via its dissociation from the resident RPA (Figure 8A, Step 3) (20,27). Hence, the ATP hydrolysis-dependent affinity of RFC for P/T junctions permits released RPA2 OBD to re-engage the P/T junction. Altogether, the results from the present study reveal that, during PCNA loading, dynamic RPA•DNA interactions and the ATP hydrolysis-dependent affinity of RFC for P/T junctions permit the reversible exchange of RPA2 OBD and RFC at a P/T junction while the RPA complex remains engaged with P/T DNA. The former is required for PCNA loading and the latter increases the efficiency of this process by prohibiting diffusion of loaded PCNA along the template ssDNA as well as RFC-catalyzed unloading of PCNA from DNA (27,35).
RPA2 OBD vacates the P/T junction during initiation of DNA synthesis while the RPA complex remains engaged with the downstream template ssDNA
Following loading of PCNA onto a P/T junction, pol δ engages the ‘front face’ of loaded PCNA, forming a pol δ holoenzyme. The results presented in Figure 7B indicate that at least RPA1 OBA, and likely other OBs of the RPA complex, remain engaged with the P/T DNA during formation of the pol δ holoenzyme. In the absence of dNTPs (i.e. no DNA synthesis), pol δ engages P/T junctions with dramatically low affinity, if at all (21,22,31,42–44). Given the instability of these interactions, we propose that pol δ (as part of a holoenzyme) does not stably capture a P/T junction that is exposed during microscopic dissociation of at least RPA2 OBD from the from the 3′ terminus of the primer strand and the adjacent template ssDNA (Figure 8B, Step 1). In the presence of dNTPs, pol δ (as part of a holoenzyme) engages a P/T junction and aligns an incoming, complementary dNTP for incorporation at the 3′ terminus of the primer strand (27,31,32). Adoption of the initiation state for DNA synthesis decreases the distance between loaded PCNA and the 5′ terminus of the primer strand (Figure 6B, C) (32). Based on the time-dependent change in EFRET observed in Figure 6B above, it is estimated that the average distance between loaded PCNA and the 5′ terminus of the primer strand decreases by 8.91 ± 2.04 Å during adoption of the initiation state for DNA synthesis (Supplementary Information, Supplementary Table S2) (48).
In the initiation state for DNA synthesis, pol δ directly engages a P/T junction in a manner that shelters the 3′ terminus of the primer strand and ∼6 nt of the template ssDNA immediately downstream of the P/T junction (31,32). These interactions significantly overlap with those of RPA at a P/T junction (12–18,31,32). In the present study, results from novel, ensemble FRET assays provide unprecedented insights into how the significant overlap of these protein•DNA interactions is accommodated during initiation of DNA synthesis by pol δ holoenzymes (Figure 8B, Steps 2 and 3). Specifically, the results presented in Figure 7C reveal that at least RPA1 OBA remains engaged with the template ssDNA downstream of a P/T junction, distal to the pol δ ssDNA footprint. Given the significant overlap of the interactions of pol δ (in the initiation state) and RPA with P/T DNA as well as the dynamics of RPA2 OBD during binding and activation of loading complexes (discussed above), we propose that pol δ captures a P/T junction that has been transiently vacated by at least RPA2 OBD (via during microscopic dissociation) and rapidly aligns an incoming, complimentary dNTP for incorporation (Figure 8B, Step 2) (27,31,32). Based on the ssDNA footprint of pol δ in the initiation state (discussed above), other RPA OBs may also release from the template ssDNA immediately adjacent to the P/T junction (31). For the P/T DNA substrates utilized in the present study, initiation of DNA synthesis at the P/T junction leaves ∼27 nt of ssDNA at the 5′ end of the template strand for at least RPA1 OBA to remain engaged with. However, other RPA OBs are likely to remain engaged as well as this ssDNA length is sufficient for OBA, OBB and OBC of the RPA1 subunit to engage the RPA complex in a tight interaction (KD < 5 nM) (49,52,53). Upon catalysis of the initial dNTP incorporation, pol δ may continue to catalyze processive dNTP incorporation, converting the template ssDNA to dsDNA. The affinity of human RPA for dsDNA is approximately 3 orders of magnitude weaker than its affinity for ssDNA (54,55) (Supplementary Information, Supplementary Figure S14). Hence, DNA synthesis by a DNA polymerase such as pol δ indirectly releases RPA into solution (Figure 8B, Step 3A) by converting optimal RPA substrates (template ssDNA, KD≤ 100 pM, Figure S14B) to poor RPA substrates (dsDNA, KD = 0.663 ± 0.082 μM, Supplementary Figure S14C) (56,57). Alternatively, pol δ may dissociate into solution upon the initial dNTP incorporation, permitting the relinquished interactions of RPA2 OBD (and likely other OBs) to re-establish with a P/T junction (Figure 8B, Step 3B). In this scenario, the engaged RPA complex increases the efficiency of DNA synthesis by prohibiting diffusion of loaded PCNA along the ssDNA template and RFC-catalyzed unloading of PCNA from DNA, allowing pol δ to re-engage loaded PCNA and re-initiate DNA synthesis.
Data availability
Exact sequences, exact details of chemical modifications at any position, and source of synthetic nucleic acid oligonucleotides are included in the main text and Supplementary Material.
Supplementary data
Supplementary Data are available at NAR Online.
Acknowledgements
We would like to thank all members of the Hedglin and Antony labs for their efforts in reviewing/proofreading this manuscript.
Funding
National Institutes of Health [F99CA274696 to S.K., R01 GM130756, R01 GM133967, R35 GM149320, S10 OD030343 to E.A., R35 GM147238-02 to M.H.]. Funding for open access charge: NIGMS [R35 GM147238-02 to M.H.].
Conflict of interest statement. None declared.
Comments