-
PDF
- Split View
-
Views
-
Cite
Cite
Pooja Srinivas, Rebecca E Steiner, Ian J Pavelich, Ricardo Guerrero-Ferreira, Puneet Juneja, Michael Ibba, Christine M Dunham, Oxidation alters the architecture of the phenylalanyl-tRNA synthetase editing domain to confer hyperaccuracy, Nucleic Acids Research, Volume 49, Issue 20, 18 November 2021, Pages 11800–11809, https://doi.org/10.1093/nar/gkab856
- Share Icon Share
Abstract
High fidelity during protein synthesis is accomplished by aminoacyl-tRNA synthetases (aaRSs). These enzymes ligate an amino acid to a cognate tRNA and have proofreading and editing capabilities that ensure high fidelity. Phenylalanyl-tRNA synthetase (PheRS) preferentially ligates a phenylalanine to a tRNAPhe over the chemically similar tyrosine, which differs from phenylalanine by a single hydroxyl group. In bacteria that undergo exposure to oxidative stress such as Salmonella enterica serovar Typhimurium, tyrosine isomer levels increase due to phenylalanine oxidation. Several residues are oxidized in PheRS and contribute to hyperactive editing, including against mischarged Tyr-tRNAPhe, despite these oxidized residues not being directly implicated in PheRS activity. Here, we solve a 3.6 Å cryo-electron microscopy structure of oxidized S. Typhimurium PheRS. We find that oxidation results in widespread structural rearrangements in the β-subunit editing domain and enlargement of its editing domain. Oxidization also enlarges the phenylalanyl-adenylate binding pocket but to a lesser extent. Together, these changes likely explain why oxidation leads to hyperaccurate editing and decreased misincorporation of tyrosine. Taken together, these results help increase our understanding of the survival of S. Typhimurium during human infection.
INTRODUCTION
Accurate protein synthesis is essential for cellular survival and one important family of enzymes involved in this process are the aminoacyl-tRNA synthetases (aaRS). These enzymes add an amino acid to cognate tRNAs at the 3′ terminal adenosine (A76) (1). The addition or charging of tRNAs is extremely accurate (∼10–4) to ensure that correct amino acids are incorporated into proteins in a codon-specific manner (2). To enable this high fidelity, aaRSs discriminate between chemically-similar amino acids and have the ability to proofread and edit the incorrectly added amino acid (3). This proofreading capability allows for accurate translation to proceed.
aaRSs facilitate a two-step reaction to ligate an amino acid on a tRNA (4). First, the aaRS charges an amino acid with an adenylate group from ATP. Second, the amino-adenylate is ligated onto a tRNA. There are two structurally unrelated classes of aaRSs that differ in the location of the ligated aminoacyl group and in their editing functions (5,6). Class I synthetases aminoacylate the 2′-OH of the terminal A76 of a cognate tRNA (7,8), while Class II aaRSs generally aminoacylate tRNA at the 3′-OH of A76 (9). Class I aaRSs contain highly conserved editing domains and perform both pre-transfer editing, in which the misactivated amino acid-adenylate is hydrolyzed, and post-transfer editing, where the mischarged aminoacyl-tRNA is hydrolyzed (10–16). In contrast, class II aaRSs contain structurally diverse editing sites (17–19) and primarily rely on post-transfer editing. The diversity in class II aaRSs impart differences in their response to environmental stressors, such as increased thermal stability (20).
Bacterial PheRS is a class II aaRS that functions as a heterotetramer, composed of two α subunits and two β subunits, (αβ)2 (21). An amino acid, ATP, and tRNA bind in the aminoacylation domain of the α-subunits, and ligation occurs to the 2′-OH of tRNAPhe, distinguishing PheRS from other class II aaRSs. The PheRS β-subunits contain the editing domain, where discrimination between Phe and Tyr occurs post-transfer via hydrolysis of misacylated tRNAPhe to prevent misincorporation of Tyr at Phe codons in the growing peptide chain. Tyr misincorporation rates are high (21), but low error rates can be reconciled by post-transfer editing (8,9,22). One aspect of proofreading is the requirement for Tyr-tRNAPhe to translocate ∼30 Å to the editing domain (22,23). Conserved residue His265 interacts with the aminoacyl group (22), and discrimination between Phe and Tyr occurs by βGlu334 recognition of the Tyr hydroxyl (24). Conserved βArg244 interacts with the tRNAPhe backbone at C75, while two catalytic waters are adjacent to the aminoacyl group that assist with residues βAsn254 and βThr354 to hydrolyze the ester bond between Tyr and the 2′-OH of tRNAPhe A76 (24). Residues βGly318 and βAla356 are also implicated in proofreading activity, as mutations in these residues prevent Tyr-tRNAPhe accommodation into the PheRS editing site (25).
PheRS proofreading activity is of particular importance when bacteria are subjected to oxidative stress (26,27). During oxidative stress, imbalances of reactive oxygen species damage various cellular components, including both proteins and nucleic acids (28–33). Damages include oxidation of free amino acids that alters endogenous amino acid pools whose limitation affects translational fidelity (29). Specifically, oxidative stress increases concentrations of ortho, para, and meta-Tyr isomers due to Phe oxidation, challenging PheRS proofreading by increasing the likelihood of tRNAPhe misacylation, and subsequent mistranslation (29).
Salmonella enterica serovar Typhimurium is a pathogen that infects the gastrointestinal (GI) tracts of humans and survives exposure to oxidative stress both in the GI tract and in the lysosomes of macrophages (27,34). Previous studies show that oxidized PheRS in Salmonella enterica serovar Typhimurium is hyperaccurate during oxidative conditions, increasing translational fidelity (25). Upon hydrogen peroxide (H2O2) treatment, PheRS displays increased proofreading activity and lower misacylation rates without changes in canonical aminoacylation activity, protecting cells from mistranslation under adverse growth conditions. Several residues located throughout PheRS become oxidized resulting in changes to secondary structural features (25). Here, we solved a 3.6 Å cryogenic-EM (cryo-EM) structure of the oxidized S. Typhimurium editing-deficient PheRS βG318W to assess structural changes that may contribute to increased proofreading. We report that PheRS oxidation induces conformational rearrangements of both the α and β subunits providing a rationale for hyperaccuracy during oxidative stress.
MATERIALS AND METHODS
PheRS expression and purification
Salmonella enterica serovar Typhimurium PheS and PheT βG318W proteins, encoding the α- and β-subunit, respectively, were purified as previously described (25). Briefly, PheRS was affinity purified (TALON), fractions containing protein were pooled, concentrated and dialyzed overnight in sample buffer (50 mM Tris pH 7.5, 100 mM KCl, 5 mM MgCl2, 3 mM 2-mercaptoethanol, 5% glycerol). For storage at −80°C, PheRS was dialyzed 4 h in sample buffer containing 50% glycerol.
Cryo-EM sample preparation
Purified PheRS was dialyzed at 4°C for 4 h in a sample buffer containing 5 mM H2O2. Three μL of oxidized PheRS at 1.5 mg/ml was incubated for 10 s at 4°C on freshly glow-discharged grids (1.2/1.3 300 mesh Cu Quantifoil). Grids were blotted for 3 s at 100% humidity, plunged into liquid ethane with a Vitrobot Mark IV (FEI), and stored in liquid nitrogen.
Electron microscopy, image processing, and data analysis
A 1330 micrograph dataset was acquired using a Talos Arctica transmission electron microscope (ThermoFisher) operating at 200 keV with BioQuantum/K2 Summit direct electron detector (Gatan). Micrographs were collected at a pixel size of 1.04 Å/pixel at a defocus range of −0.5 to −3.5 μm. A total dose of 64.67 e/Å2 per micrograph was fractioned into 48-frame movies with 12 s exposure time.
Image processing was conducted in Relion 3.0 (35). Motion correction and dose weighting was done using MotionCorr2 (36), and contrast transfer function parameters were estimated using Gctf (37). Laplacian of Gaussian algorithm was used to pick particles and generate templates for 2D-referenced based autopicking. Several rounds of 2D-reference based autopicking were conducted, with incorrectly selected particles discarded after reference-free 2D class averaging. An initial three-dimensional refinement with C2 symmetry was conducted using a previously published PheRS structure (PDB code 4P71 (38)) low-pass filtered to 45-Å in Chimera as a reference map (39) (Supplementary Figure S1). Three-dimensional refinement was followed by a 3D classification step, with poorly aligned particles discarded. A total of 204 902 particles were finally selected and subjected to particle polishing, 3D refinement, CTF refinement, and post-processing to yield a final reconstruction of 3.6 Å. Resolution estimations were calculated from Fourier shell correlations (FSC) at 0.143 between the two independently refined half-maps. Local resolution was calculated in Relion. The final map was refined and validated in PHENIX (40) and modeled with PDB code 3PCO (14). Coot (41) and UCSF ChimeraX (42) were used for model building.
Maps were analyzed using UCSF Chimera (39) and ChimeraX (42). Global structural alignments and RMSD calculations were conducted in ChimeraX, using the matchmaker tool which creates a global sequence alignment then fits aligned residue pairs (43). Distance calculations were all conducted in ChimeraX, from the Cα carbon of a residue. Figures were created using UCSF Chimera and ChimeraX.
RESULTS
Global structural impact of oxidation on PheRS βG318W
Treatment of S. Typhimurium wild-type (WT) and editing-deficient PheRS βG318W with H2O2 results in different residues becoming oxidized (25). The PheRS βG318W variant has a 7.5-fold reduction in proofreading due to the prevention of Tyr-tRNAPhe binding in the editing site, likely by steric hindrance (24). Both S. Typhimurium oxidized WT and PheRS βG318W show increased proofreading capacity, with PheRS βG318W displaying the most pronounced effect with a >3-fold increase in Tyr-tRNAPhe proofreading (25). Several PheRS βG318W residues were oxidized across both the α- and β-subunits but outside of catalytic regions, and thus it is unclear how increased proofreading results. To define the structural changes that PheRS undergoes during oxidative stress, we solved a 3.6-Å resolution structure of oxidized PheRS βG318W by single particle cryo- EM (Figure 1A; Supplementary Table S1, Supplementary Figure S1). PheRS adopts a heterotetrametric conformation composed of two α-subunits and two β-subunits (αβ)2 with the α-subunit containing the aminoacylation catalytic domain (residues 108–307) and the β-subunit containing the editing domain (residues 216–354) (14,44–47) (Figure 1C and D). Due to the resolution, it is not possible to resolve the oxidation of specific residues (Figure 1B), but mass spectrometry revealed extensive oxidation under treatment conditions (25).
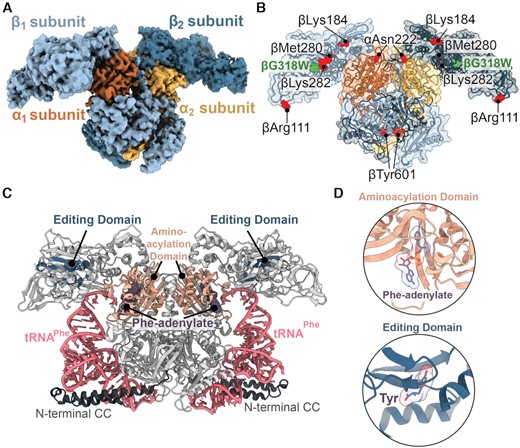
Overview of the PheRS βG318W structure. (A) The 3.6 Å map of the oxidized S. Typhimurium editing-deficient PheRS βG318W tetramer. PheRS is composed of two α-subunits (orange and yellow) and two β-subunits (light blue and dark blue). (B) Oxidized PheRS βG318W (green) with oxidized residues, as determined previously (25), shown as red spheres. (C) T. thermophilus PheRS (grey) containing Phe-adenylate (purple, surface) and tRNAPhe (pink) (PDB code 2IY5). The N-terminal coiled-coil extensions (N-terminal CC (dark grey); residues 1–88) in the α-subunits are resolved when bound to tRNA. (D) Aminoacylation (peach) and editing domains (dark blue) are shown with respective ligands, Phe-adenylate and Tyr, bound (purple, PDB code 2AMC).
PheRS undergoes a conformational change upon tRNAPhe binding (44,45), including the ordering of the N-terminal coiled-coil domain of the α-subunit (residues 1–88) (Figure 1C) (48). This region is not resolved in our structure likely because this structure is in the absence of tRNAPhe (Figure 1A). S. Typhimurium PheRS is similar to the previously published Pseudomonas aeruginosa apo-PheRS structure (apo-PaPheRS) (PDB code 4P71; root mean square deviation (RMSD) of ∼2.9 Å comparing the Cα of 790 residues) (38). S. Typhimurium PheRS and Pa PheRS share high sequence identity between both subunits (51.5% for the β-subunits and 65.7% for the α-subunits) and adopt identical tertiary folds (Supplementary Figures S2A and S3). For these reasons, and because our structure is also apo, all future comparisons will be made to apo-PaPheRS unless otherwise noted.
Oxidation induces conformational changes in the PheRS βG318W catalytic domains
Different residues are oxidized in wild-type versus the PheRS βG318W editing-deficient variant, likely due to differences in the overall architecture (25). Six residues become oxidized in PheRS βG318W, which are located across the enzyme domains, with five residues in the β-editing domain and one residue in the α-aminoacylation domain (25) (Figure 1B; Supplementary Table S2). Comparison of PheRS βG318W with apo-PaPheRS reveals that oxidized βMet280 and βLys282 undergo large changes in the position of their backbone (6.7 and 8.6 Å, respectively) while their side chain conformation is minimally impacted (Figure 2B and C). These residues are in the β-catalytic domain (Figure 2A) located adjacent to the editing site (Figure 1D). The entire β-strand surrounding the editing domain moves in towards the tRNA binding site in the β-subunit, likely contributing to the shift and conformational changes to editing site residues. Other residues within the β-subunit become oxidized yet this has little to no impact on their conformations. For example, ox-βLys184, ox-βTyr60 and ox-αAsn222 all have limited movement (Supplementary Figure S4), so it is unlikely that these rotamer changes play a significant role in the hyperaccuracy phenotype. In contrast, ox-βArg111 has a ∼180° rotation compared to EcPheRS complexed with Phe-AMP (Supplementary Figure S4F), and a 16.6 Å movement inwards towards the editing domain compared to apo-PaPheRS (Supplementary Figure S4E). However, since βArg111 is on the surface of the β-subunit, this difference may be a result from how apo-PaPheRS packs in the crystal lattice (Supplementary Figure S5). Together, these data demonstrate that oxidation has a differential effect on local conformation of the PheRS residues, and oxidation of a given residue does not necessarily entail conformational change in that region.
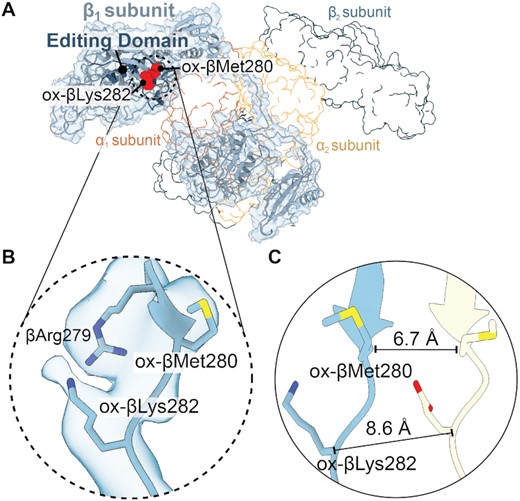
PheRS βMet280 and βLys282 residues located outside the editing domain move substantially upon oxidation. (A) Overview of the β1 subunit map and model (light blue) with the editing domain (dark blue) and oxidized residues (red spheres). The other PheRS subunits are shown as outlines. (B) Map and model of ox-βMet280 and ox-βLys282 located in the editing domain. (C) Comparison of ox-βMet280 and ox-βLys282 (corresponding residue in P. aeruginosa is Glu281) with P. aeruginosa apo-PheRS (yellow, PDB code 4P73) revealing significant movements of ∼6.7 and ∼8.6 Å, respectively.
PheRS oxidation causes structural rearrangements of its editing domain
After PheRS misacylates tRNAPhe with a Tyr, Tyr-tRNAPhe translocates to the editing domain in the β-subunit (Figure 3A) (22,24). In the editing domain, many residues surround Tyr to recognize and edit including βArg244, βAsn254, βHis265, βGlu334 and βThr354 (Figure 3B). The tRNA is typically stabilized by interactions of βArg244 with nucleotide C75, while βAsn254 and βThr354 are in proximity to the aminoacyl ester bond and involved in substrate binding and hydrogen bonding with catalytic waters (Figure 3B and C) (24). These residues were not demonstrated to be oxidized yet they display altered conformations (Figure 3D). βArg244, βAsn254 and βThr354 all shift away from the tRNA binding location at distances between ∼5.4 and 7.2Å (Figure 3D; Supplementary Figure S6A). Notably, the βArg244 flips ∼180° towards the editing site (Figure 3D).
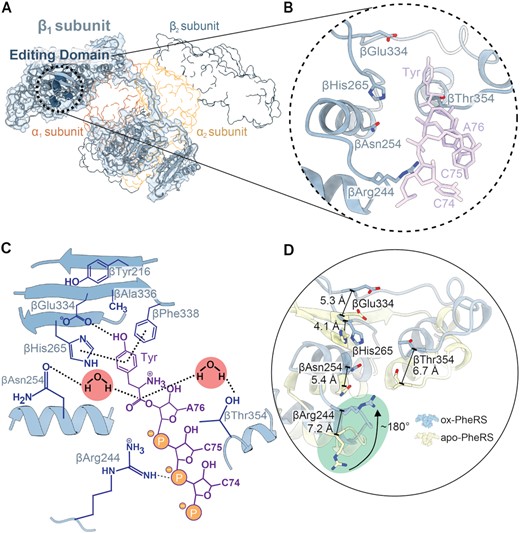
Localized active site conformational changes of the PheRS editing domain. (A) Oxidized S. Typhimurium editing-deficient PheRS βG318W β1-subunit with corresponding map (light blue) and active site residues in the editing domain shown as spheres (dark blue). The other PheRS subunits are shown in outline. (B) Closeup of editing site residues with Tyr-tRNAPhe docked into the editing site (PDB code 3HFZ, purple). (C) Overview of the editing site residues (blue) and interactions with a Tyr-tRNAPhe CCA end (purple), and the two catalytic waters that carry out the reaction. (D) Residues of the editing domain of the oxidized S. Typhimurium PheRS compared to the P. aeruginosa apo-PheRS (yellow, PDB code 4P73) undergo a significant shift, particularly βArg244 which is shifted ∼7.2 Å and flipped ∼180° inwards towards the editing site (green circle).
The changes in residues located in the PheRS editing domain alter how these residues interact with Tyr and the tRNA backbone of C75. βGlu334 plays an important role in tyrosine discrimination via the formation of hydrogen bonds with the hydroxyl group of Tyr as assessed by molecular docking studies (24). In our structure, βGlu334 shifts away from the editing site ∼5.3 Å (Figure 3D). The βHis265 stabilizes substrate binding through π-stacking interactions with the Tyr (Figure 3C) (23,24). Other well-conserved editing site residues form an antiparallel β-sheet that upon oxidation, appears to be disrupted (Figure 4A and B) (24). None of the editing site residues are oxidized but this global oxidation disrupts the hydrogen bonding network of the β-sheet, shifting the β-sheet away from the editing site by ∼4.5 Å (Figure 4C; Supplementary Figure S6B) (38). This movement creates a larger binding surface (increases from ∼204 Å2 to ∼295 Å2) (42) for the incorrect amino acid to be edited (Figure 4D and E). Despite the relatively moderate changes in the locations of the oxidized residues (Figure 2; Supplementary Figure S4), there is extensive rearrangement of β-subunit residues involved with editing activity that result in a larger editing domain.
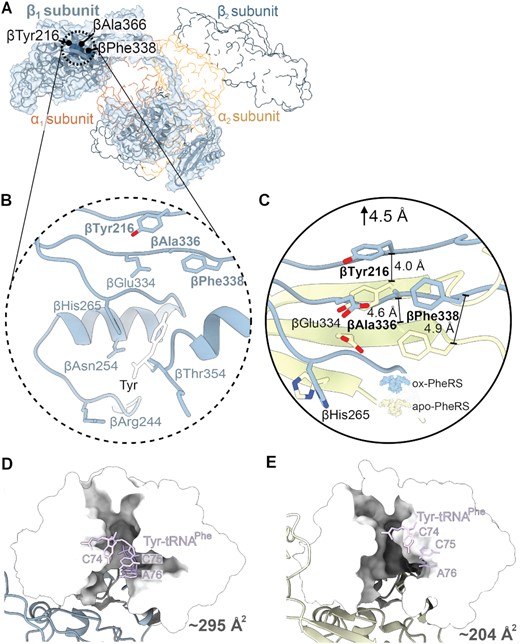
Oxidization of PheRS βG318W opens the editing domain leading to hyperaccuracy. (A) Structure of oxidized S. Typhimurium PheRS βG318W β1-subunit with corresponding map density (blue) and catalytic residues that are implicated in editing activity shown as dark blue spheres, with other subunits shown in outline. (B) Closeup of the catalytic region with Tyr overlaid from a T. thermophilus PheRS-Tyr structure (white, PDB code 3HFZ). (C) The catalytic residues βTyr216, βAla336, and βPhe388 of the oxidized S. Typhimurium PheRS undergo a shift averaging ∼4.5 Å away from the editing domain compared to the P. aeruginosa apo-PheRS (PDB code 4P73, yellow). (D) Editing domain of oxidized PheRS βG318W shown as surface (white) with Tyr-tRNAPhe docked in site (PDB code 3HFZ, purple). (E) Editing site residues of apo-PheRS shown as surface (white) with Tyr-tRNAPhe docked into the editing site (PDB code 3HFZ, purple).
PheRS oxidation induces structural changes in the α-subunit active site
The catalytic domain of the PheRS α-subunits form interactions with Phe and AMP, allowing for the specific addition of a phenylalanyl-adenylate to tRNAPhe (Figure 5A and B; Supplementary Figure S7A). The active site is composed of three regions: the motif 2 loop (residues 194–205), the second helical loop (h2, residues 145–158), and the phenylalanine-proline-phenylalanine (FPF) loop (residues 246–254) (Figure 5B−E; Supplementary Figure S7) (14,44,45). The only α-subunit residue that is oxidized is αAsn222, which is located outside the active site but its backbone conformation is not affected by oxidation (25) (Supplementary Figure S2B). However, global oxidation results in conformational differences within the phenylalanyl-adenylate binding pocket (Figure 5C−E).
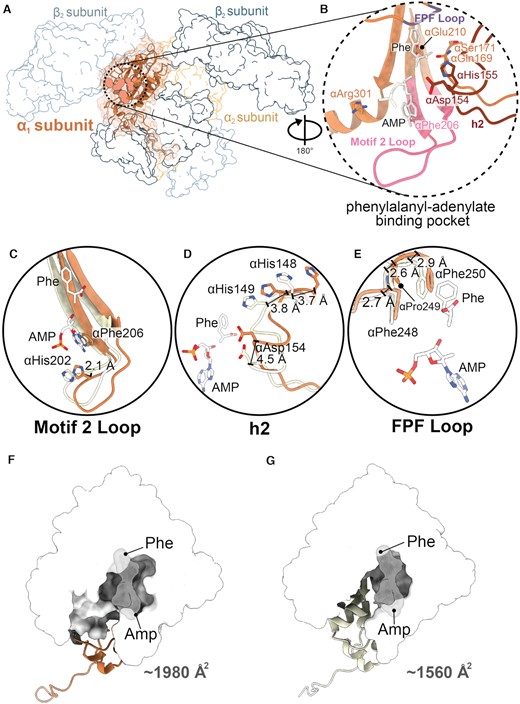
Conformational changes of the aminoacylation domain. (A) Structure of oxidized S. Typhimurium PheRS βG318W α1-subunit and map (orange). Aminoacylation active site residues shown as spheres, with other subunits shown in outline. (B) Active site residues in the phenylalanyl-adenylate binding pocket (orange) with modeled AMP and Phe (PDB code 3PCO, white). The motif 2 loop (pink), helix 2 loop (h2, dark red), and FPF loop (purple) are shown. (C) The motif 2 loop shifts outwards by ∼2.1 Å from P. aeruginosa apo-PheRS (PDB code 4P73, yellow). (D) The h2 of oxidized PheRS has conformational rearrangements, 3.7 Å and 3.8 Å at αHis148 and αHis149, respectively, and 4.5 Å at αAsp154, away from the phenylalanyl-adenylate binding pocket compared to apo-PheRS (PDB code 4P73, yellow). (E) The FPF loop contains minor conformational changes, with 2.7, 2.6 and 2.9 Å shifts of the αPhe248, αPro249 and αPhe250, respectively, away from the phenylalanyl-adenylate binding pocket compared to apo-PheRS (PDB code 4P73, yellow) (F) Enlargement of the aminoacylation domain shown with surface (white) of oxidized PheRS βG318W, with AMP and Phe groups docked in and shown with surfaces (PDB code 3PCO, grey). (G) Enlargement of the aminoacylation domain shown as surface (white) of the apo-PaPheRS (PDB code 4P73, yellow), with AMP and Phe groups docked in shown as surfaces (PDB code 3PCO, grey).
In the motif 2 loop, αHis202, which normally forms hydrogen bonds with AMP, moves ∼2.1 Å away from the Phe-adenylate (Figure 5C; Supplementary Figure S7B). However, not all catalytic residues in the motif 2 loop are affected. For example, αPhe206, which forms π-stacking interactions with the adenosine, adopts the same conformation as non-oxidized PheRS (Figure 5C). The h2 and FPF loop of the phenylalanyl-adenylate binding pocket also undergo conformational shifts (Figure 5). The second helical loop (h2), a segment that undergoes rearrangement upon Phe and AMP binding (14,45), undergoes conformational changes upon oxidization despite that none of the h2 residues are oxidized (Figure 5D; Supplementary Figure S7C). Both αHis148 and αHis149 shift away from the active site ∼3.7 and ∼3.8 Å, respectively (Figure 5D). Normally, tRNAPhe, and specifically the last nucleotide A76, is recognized by contacts from the side chain of αAsp154 located within h2 (Figure 5D; Supplementary Figure S8). In oxidized PheRS βG318W, αAsp154 shifts ∼4.5 Å away from the tRNA binding site. The FPF loop, which is conserved across all bacterial species (14), forms π-stacking interactions with Phe (Supplementary Figure S8). Within the FPF loop, αPhe248 shifts ∼2.7 Å away from where Phe binds, which would prevent this interaction (Figure 5E; Supplementary Figure S7D). These movements in the motif 2, h2 and FPF loops enlarge the phenylalanyl-adenylate binding pocket from ∼1560 to ∼1980 Å2 (Figure 5F and G).
Upon oxidation, there is a 30% decrease in misacylation of tRNAPhe with Tyr (25). Discrimination between Phe and Tyr is facilitated by αGlu210 within h2 which hydrogen bonds to the bound amino acid to facilitate ligation (Figure5B and D; Supplementary Figure S8). In our structure, the position of αGlu210 moves to a position that would directly overlap with the hydroxyl group of Tyr (14) (Supplementary Figure S9) as compared to a Escherichia coli PheRS structure containing Phe and AMP (PBD code 3PCO). This new αGlu210 position may explain why misacylation decreases upon PheRS oxidation.
Similarities between oxidized S. Typhimurium PheRS and the E. coli PheRS–Phe–AMP complex
Oxidation causes both larger editing and aminoacylation sites as compared to apo-PaPheRS as previously described (an increase of 204–295 Å2 (Figure 4D and E) and an increase of 1560–1900 Å2 (Figure 5F and G), respectively). Although all comparisons to this point have been of oxidized apo-PheRS βG318W to apo-PaPheRS, interestingly, specific regions of oxidized apo-PheRS are slightly more similar to the structure of EcPheRS complexed with ligands Phe and AMP (PDB code 3PCO (14), RMSD of ∼1.7 Å). While binding of Phe and AMP to EcPheRS increases the editing (∼272 Å2) and Phe-adenylate binding pocket (∼1700 Å2), these sites are still smaller than when PheRS is oxidized (Supplementary Figures S10D and S11B).
One of the most striking changes between oxidized apo-PheRS and EcPheRS bound to Phe and AMP is the placement of βMet280 and βLys282, residues that both become oxidized but are located outside the two catalytic sites (Figure 2). Normally the backbone of these residues dramatically changes upon binding of Phe and AMP (changes of 9.4 Å (βMet280) and 10.3 Å (βLys282)). Oxidation of βMet280 and βLys282 also influences their positions however, now these residues adopt similar positions as when Phe and AMP bind (Supplementary Figure S10A) (14). This movement places these residues closer towards the editing domains where tRNA binds despite not containing any ligands.
Comparison of Phe-AMP bound EcPheRS with oxidized apo-PheRS also reveals changes of editing site residues that appear to enlarge the binding pocket and change how oxidized PheRS would engage either the amino acid or AMP (Supplementary Figure S10). First, the side chain of βHis265 flips away from the amino acid binding site (Supplementary Figure S10B), likely influencing recognition of the incorrect Tyr. This flipping may occur as PheRS transitions to prepare for hydrolysis of incorrectly bound Tyr. The βArg244 sidechain, which normally recognizes the tRNA backbone of nucleotide C75, is rotated ∼180° away in the absence of tRNA (Figure 3C). Oxidation of PheRS causes βArg244 to more resemble EcPheRS-Phe-AMP despite lacking the tRNA (Supplementary Figure S10B). Lastly, oxidation cause the entire β-sheet comprising the editing domain to shift by ∼2.7–3.7 Å and rotate ∼45° thus placing it further away from the aminoacylated-tRNA binding site (Supplementary Figure S10C). Collectively, these changes create a larger editing site but with active site residues positioned in conformations to allow for the increase in editing activity of the oxidized PheRS.
DISCUSSION
To prevent protein mistranslation, aminoacyl-tRNA synthetases pair correct amino acids to their cognate tRNAs with high fidelity to ensure the correct addition of amino acids into proteins. Environmental stress, including oxidative stress, alters cellular amino acid pools and this change can affect the fidelity of gene expression. During oxidative stress, oxidation of Phe converts it to Tyr, increasing Tyr isomer levels and leads to an overall increase in tRNAPhe misacylation (29). However, oxidation of PheRS compensates by increasing proofreading to reduce misacylated Tyr-tRNAPhe from participating in protein synthesis. Several PheRS residues are oxidized and these modifications are found throughout PheRS and not localized solely at the active site (29). Since oxidation causes a hyperaccurate phenotype but there is no oxidation at the active sites, this suggests that allosteric changes may contribute to PheRS acquiring hyperaccuracy consistent with previous results that demonstrated global changes (25). Based upon these results, we set out to determine the cryo-EM structure of oxidized PheRS. Overall, we find that oxidation results in structural changes mainly in the β-subunit editing domain and to a lesser extent, the α-subunit aminoacylation active site. The β-subunit editing domain is enlarged where mischarged Tyr-tRNAPhe would bind and its surface area increases by 50%. Oxidization also enlarges the phenylalanyl-adenylate binding pocket in the α-subunit by 30%. These enlargements are predicted to contribute to faster and efficient binding, while additional conformational changes of catalytic residues suggest an improvement in Tyr hydrolysis required to ligate the correct Phe. These results shed light on how S. Typhimurium is able to survive and grow in adverse environments.
Based on high structural homology between bacterial PheRS (49,50), it is likely that most bacterial PheRS enzymes have a similar hyperaccuracy phenotype under oxidative stress. Although there is typically high homology among mitochondrial and bacterial synthetases (51), PheRS is an exception as the mitochondrial and bacterial synthetase are divergent (52). Mitochondrial PheRS is a monomer and lacks an editing domain found in the tetrameric bacterial PheRS (52), and as a consequence, is unable to proofread misacylated tRNAPhe (52). Although mitochondrial PheRS is oxidized during oxidative stress, this does not result in hyperaccuracy and oxidation can be reversed (25), restoring normal function.
While oxidation of other synthetases including ThrRS and AlaRS can occur, this does not result in hyperaccuracy, in contrast to PheRS. ThrRS is known to misacylate tRNAThr with Ser and contains a catalytic cysteine in the editing domain (53). Under oxidative conditions, this reactive cysteine is oxidized and thus prevents ThrRS editing ability (54), the opposite phenotype to what is observed with PheRS (25,53). AlaRS also relies on a critical cysteine residue to carry out editing activity upon misacylation of a Ser- or Gly-tRNAAla (55), but changes in AlaRS editing activity upon oxidation have not yet been reported. Changes in AlaRS editing activity are significantly more determinantal for bacteria viability compared to ThrRS (56). From a fitness perspective, maintenance of AlaRS editing activity is more important for bacterial survival, suggesting possible divergence in editing response under oxidation as compared to ThrRS. Under normal growth conditions, PheRS editing activity is not vital for bacterial viability, but becomes required for growth under oxidative conditions (29). Translation accuracy under oxidative stress is vital for cellular survival, but among the class II aaRSs, only PheRS undergoes a gain of function change. The benefit of PheRS hyperaccuracy under conditions that directly increase the likelihood of mistranslation serves as a direct compensatory mechanism to decrease the likelihood of Tyr misincorporation; while ThrRS and possibly AlaRS are susceptible to oxidative stress, the Ser or Gly amino acid pools are not expected to change significantly, placing less selective pressure on these synthetases to compensate for misacylation under oxidative conditions.
Oxidation is a well characterized signal for proteins directly involved in the oxidative stress response including redox-operated switches that activate transcription of oxidative response elements (57). However, these mechanisms are indirect and contribute to a regulatory pathway that allows for increased cell survival under adverse conditions. PheRS represents one of the few examples of a functional protein that self-regulates as a feedforward mechanism to directly compensate against an adverse condition.
Responses to stress are typically analyzed at the level of gene expression (58,59). With oxidative stress, several genes including oxyR, oxyS, or perR, are directly regulated by oxidation of several cysteine residues allowing for transcription factor binding to promote the expression of proteins involved in metabolism or clearance of oxidative stress (60). The hyperaccuracy phenotype of PheRS under oxidative stress illustrates how important pathways in protein synthesis work to maintain proper gene expression. This ensures successful expression of oxidative response genes, which relies on accurate amino acid additions to growing nascent chain on the ribosome. Oxidized PheRS hyperaccuracy ensures that correct gene expression is not compromised by elevated and potentially toxic Tyr isomer misincorporation under oxidative stress. Hyperaccurate aminoacylation thus helps to increase survival, particularly for organisms regularly present in oxidative environments like S. Typhimurium, which can thrive in environments like a macrophage despite a host reactive oxygen species attack. Overall, the proper activation of stress responses, including increased proofreading activity of PheRS, increases S. Typhimurium survival and contributes to the overall fitness of the bacterium.
DATA AVAILABILITY
Atomic coordinates and maps for the reported cryo-EM structures have been deposited with the Protein Data bank under accession number 7N8Y and Electron Microscopy Data bank under accession number EMD-24249.
SUPPLEMENTARY DATA
Supplementary Data are available at NAR Online.
ACKNOWLEDGEMENTS
We thank Dunham lab member Dr Alexandra Kuzmishin Nagy for critical reading of the manuscript and Ha An Nguyen for technical support.
FUNDING
National Institutes of Health [R01GM065183 to M.I. and C.M.D., R01 GM093278 to C.M.D., 5T32 GM008602 to P.S., T32 AI106699 to P.S.]; Burroughs Wellcome Fund Investigator in the Pathogenesis of Infectious Disease award (to C.M.D.); Robert P. Apkarian Integrated Electron Microscopy Core (IEMC) at Emory University, which is subsidized by the School of Medicine and Emory College of Arts and Sciences. Funding for open access charge: National Institutes of Health.
Conflict of interest statement. None declared.
Notes
Present address: Puneet Juneja, Cryo-EM Facility, University of Iowa, Ames, IA 50011-1079 USA; Michael Ibba, Schmid College of Science and Technology, Chapman University, Orange, CA 92866, USA.
Present address: Rebecca E. Steiner, College of Osteopathic Medicine, Lake Erie College of Osteopathic Medicine, Bradenton, FL 34211, USA.
Comments