-
PDF
- Split View
-
Views
-
Cite
Cite
Weihui Li, Meng Li, Lihua Hu, Jingpeng Zhu, Zhiwei Xie, Jiarui Chen, Zheng-Guo He, HpoR, a novel c-di-GMP effective transcription factor, links the second messenger’s regulatory function to the mycobacterial antioxidant defense, Nucleic Acids Research, Volume 46, Issue 7, 20 April 2018, Pages 3595–3611, https://doi.org/10.1093/nar/gky146
- Share Icon Share
Abstract
Cyclic di-GMP (c-di-GMP) is a global signaling molecule that widely modulates diverse cellular processes. However, whether or not the c-di-GMP signal participates in regulation of bacterial antioxidant defense is unclear, and the involved regulators remain to be explored. In this study, we characterized HpoR as a novel c-di-GMP effective transcription factor and found a link between the c-di-GMP signal and the antioxidant regulation in Mycobacterium smegmatis. H2O2 stress induces c-di-GMP accumulation in M. smegmatis. High level of c-di-GMP triggers expression of a redox gene cluster, designated as hpoR operon, which is required for the mycobacterial H2O2 resistance. HpoR acts as an inhibitor of the hpoR operon and recognizes a 12-bp motif sequence within the upstream regulatory region of the operon. c-di-GMP specifically binds with HpoR at a ratio of 1:1. Low concentrations of c-di-GMP stimulate the DNA-binding activity of HpoR, whereas high concentrations of the signal molecule inhibit the activity. Strikingly, high level of c-di-GMP de-represses the intracellular association of HpoR with the regulatory region of the hpoR operon in M. smegmatis and enhances the mycobacterial H2O2 resistance. Therefore, we report a novel c-di-GMP effective regulator in mycobacteria, which extends the second messenger’s function to bacterial antioxidant defense.
INTRODUCTION
Transcriptional regulation is essential for every living organism to rapidly adapt to changing environments. The oxidative stress is an unavoidable by-product of aerobic processes that can damage biological macromolecules (1,2). To counter oxidative stress, bacteria have evolved an ability to deliver the stress signal into increased expression of antioxidant enzymes. However, the mechanisms by which bacteria adaptively trigger antioxidant regulation are poorly understood. Cyclic diguanylate monophosphate (c-di-GMP), synthesized from two molecules of guanosine triphosphate (GTP), is an important and well-conserved second messenger in bacteria (3,4). It participates in the regulation of a wide range of cellular processes, such as biofilm formation (5,6), bacterial motility (7,8), virulence (9,10), cell-cycle progression and cell development (11–13) and host immune responses (14–16). Oxidative stress is a common extracellular and intracellular environment cue for bacteria, but whether or not the c-di-GMP signal is linked to antioxidant regulation is unclear.
Upon responding to environment signals, c-di-GMP drives bacteria to achieve physiological adaptation critically through its downstream receptors (3,17). Several types of c-di-GMP receptors have been successfully identified from different bacterial species. PilZ proteins were the first identified c-di-GMP receptors (18,19). GGDEF and/or EAL domain-containing proteins (11,20) represent most receptor proteins characterized so far. In addition, c-di-GMP binds to riboswitches situated in the 5′-untranslated regions of various mRNAs (21,22). Strikingly, transcription factors have recently been identified to act as receptors of the second messenger (8–10). c-di-GMP can regulate various physiological functions through its receptor transcription factors. For example, FleQ, VpsT and MrkH modulate biofilm formation and bacterial motility (8,19,23). Both Clp and Bcam1349 are involved in the regulation of bacterial virulence (24,25). LtmA regulated lipid metabolism and transport in M. smegmatis (26), and BldD regulates bacterial development (12).
Mycobacteria are slow-growing bacteria, including pathogens and saprophytes, with a unique antioxidant capacity. For example, Mycobacterium tuberculosis, the causative agent of tuberculosis (TB), thrives in oxidative environments such as the macrophage during infection and leads to millions of human deaths worldwide each year (27). Mycobacterium smegmatis is a non-pathogenic mycobacterium that demonstrates a unique antioxidant ability. Recent studies have used M. smegmatis as a model to study the transcription regulatory mechanisms of virulent mycobacterial species (28). Interestingly, M. smegmatis contains the c-di-GMP signaling system and the enzymes that metabolize c-di-GMP (29,30), which is required for the long-term survival of the mycobacteria under stressful conditions (30). Recently, LtmA has been successfully characterized as the first c-di-GMP receptor regulator in mycobacteria by screening a library of ∼500 predicted M. smegmatis transcription factors (26). It senses c-di-GMP signal and modulates the expression of lipid transport and metabolism genes (26). However, the c-di-GMP-responsive transcription factors that regulate the expression of antioxidant enzymes remain to be explored.
Mycobacteria possess a thick cell wall (31,32), a millimolar concentration of mycothiol (33,34), detoxification enzymes such as catalase (KatG) (35,36)and superoxide dismutases (SodA and SodC) (37,38), and DNA-binding proteins such as Lrs2 (39). However, no oxidative stress regulators homologous to SoxR, OxyR and OhrR in mycobacteria have been described to date. The mechanism by which transcription factors in mycobacteria regulate antioxidant stress remains to be elucidated. Whether or not the c-di-GMP signal is linked to the expression regulation of antioxidant enzymes in mycobacteria is still unclear, and the involved signaling pathway remains to be explored.
In the current paper, we report that a novel c-di-GMP effective transcription factors, HpoR, regulate the expression of a novel redox gene cluster in M. smegmatis, designated as the hpoR operon, whose regulation is required for the mycobacterial resistance to hydrogen peroxide (H2O2). This study found a link between c-di-GMP signaling and bacterial antioxidant regulation. Our finding extends the second messenger's regulatory function to bacterial defense against oxidative stress.
MATERIALS AND METHODS
Expression and purification of recombinant proteins
The related genes were amplified by polymerase chain reaction (PCR) using their respective primer pairs (5′-ATAT GAATTCGCGTGTCCAGCGATGCAGTG-3′ and 5′-AGATTCTAGATC AT CGGTCCTCTCCCAGGA-3′ for hpoR, 5′-GCGCGAATTCAAATGAT CAAG A AGACAACG-3′ and 5′-GGCCTCTAGATTAAACTCGGTTAATCAC-3′ for ydeH, 5′-TTATCG CTACGG GGGCGCAGAATTTATCATTATTG-3′ and 5′-CAATAATG AT AAATTCT GCGCCCCCGTAGCGATAA-3′ for ydeH (E208, 209A) 5′-ATCGG AATTCGCATGGGCAAAGGGGCGGCGTT-3′ and 5′-ATCTGATCTA GATCACC GACACGCCTCCGCCG-3′ for Rv1049) from genomic DNA of M. smegmatis mc2 155, M. tuberculosis or Escherichia coli K-12. Mutant genes were produced by site-directed mutagenesis and overlapping extension PCR. The amplified DNA fragments were cloned into the modified pET28a expression vectors or pMV261 overexpression vectors to produce recombinant plasmids (Supplementary Table S1). Escherichia coli BL21 cells transformed with the recombinant plasmid was grown in 200 ml of LB medium up to an OD600 of 0.6. Protein expression was induced by the addition of 0.3 mM Isopropyl β-D-1- thiogalactopyranoside. Harvested cells were resuspended and sonicated in buffer (100 mM Tris–HCl, pH 8.0, 500 mM NaCl and 10 mM imidazole) and lysate was centrifuged at 10 000 × g for 30 min. The cleared supernatant was loaded onto an affinity column and washed with wash buffer (100 mM Tris–HCl, pH 8.0, 500 mM NaCl and 100 mM imidazole). The protein was then eluted using elution buffer (100 mM Tris–HCl, pH 8.0, 500 mM NaCl and 250 mM imidazole). The eluate was then dialyzed against the buffer (20 mM Tris–HCl, 100 mM NaCl, 1 mM DL-Dithiothreitol (DTT), 10% glycerol) overnight and stored at −80°C. Recombinant plasmids, such as pMV261-hpoR and pMV261-ydeH, and the corresponding recombinant strains were constructed (Supplementary Table S1).
Transcriptomic analysis
Strains were grown in 7H9 medium where indicated at 37°C with 160 rpm shaking. The cells were cultured until mid-logarithmic phase (optical density at 600 nm [OD600], 1.0) and harvested. Total RNA was isolated using RNeasy mini kit (Qiagen, Germany). Strand-specific libraries were prepared using the TruSeq® Stranded Total RNA Sample Preparation kit (Illumina, USA) following the manufacturer’s instructions. Briefly, ribosomal RNA was removed from total RNA using Ribo-Zero rRNA removal beads. Following purification, the mRNA is fragmented into small pieces using divalent cations under 94°C for 8 min. The cleaved RNA fragments are copied into first strand cDNA using reverse transcriptase and random primers. This is followed by second strand cDNA synthesis using DNA Polymerase I and RNase H. These cDNA fragments then go through an end repair process, the addition of a single ‘A’ base and then ligation of the adapters. The products are then purified and enriched with PCR to create the final cDNA library. Purified libraries were quantified by Qubit® 2.0 Fluorometer (Life Technologies, USA) and validated by Agilent 2100 bioanalyzer (Agilent Technologies, USA) to confirm the insert size and calculate the mole concentration. Cluster was generated by cBot with the library diluted to 10 pM and then were sequenced on the Illumina HiSeq 2500 (Illumina, USA). The library construction and sequencing was performed at Shanghai Biotechnology Corporation.
Determination of mycobacterial growths
To determine mycobacterial growth curves and the effect of H2O2, the recombinant strains were grown for overnight in 7H9 medium at 37°C shaker incubator. Cells were grown to mid-logarithmic phase, and each culture was diluted (4:100) in 100 ml of fresh 7H9 broth containing the indicated concentration of H2O2. The cultures were then allowed to grow further at 37°C with shaking at 160 rpm. At different time points, serial dilutions of samples were plated on 7H10 plates for counting colony-forming units (cfu). Aliquots were taken at the indicated times. To determine the growth curves of the pre-stressed bacteria by H2O2, M. smegmatis cells were grown overnight at 37°C in 7H9 medium until log phase and 10 mM H2O2 were added for induction for 4 h. The bacterial cells were harvested and washed using fresh 7H9 medium. Then the produced bacterial cells were diluted in 100 ml of fresh 7H9 broth containing the indicated concentration of H2O2 for counting cfu. Aliquots were taken at the indicated times.
Bacterial one-hybrid assays
hpoR was cloned into the pTRG vector (Stratagene). Promoters of the M. smegmatis genes were amplified using appropriate primers and cloned into the pBXcmT vector (40). The promoter Ms5860p was amplified by PCR using a pair of primers (5′-CGAACA CGCCTTTCCGGCCTGCGACGAGTTT-3′ and 5′-AGCAT CTCGATGCCCGCG GTGAGCACCTTGC-3′). Escherichia coli XL1-Blue MRF’ Kan strain (Stratagene) was used for the routine propagation of all pBXcmT and pTRG recombinant plasmids. Bacterial one-hybrid assays were carried out as described previously (40). Positive growth co-transformants were selected on a selective screening medium plate containing 20 mM 3-AT (3-amino-1, 2,4-triazole), 16 μg/ml streptomycin, 15 μg/ml tetracyc-line, 34 μg/ml chloramphenicol and 30 μg/ml kanamycin. The plates were incubated at 30°C for 3–4 days. Co-transformants containing the pBX-Rv2031/pTRG -Rv3133 plasmids (40) served as positive control and co-transformants containing the empty vectors pBX and pTRG served as negative control.
Electrophoretic mobility shift assay (EMSA)
DNA fragments for electrophoretic mobility shift assay (EMSA) were amplified by PCR or synthesized by Invitrogen. A pair of primers (5′-CGAACACGCCTTTCCGGCCTGCGACGAGTTT-3′ and 5′-AGCATCTCGATGCCCGCGGTGAGCACCTTGC-3′) was used to amplify the upstream regulatory sequence of hpoR operon, designated as Ms5860p, by PCR from the genome of M. smegmatis mc2 155. For competition assay, the Ms5860p DNA fragment was re-amplifed with the fluorescein Isothiocyanate (FITC)-labeled reverse primer. The DNA-binding assays were performed according to a previously described procedure (41)with several changes. The reactions (20 μl) for measuring the mobility shift contained DNA fragments and various amounts of protein diluted in a buffer containing 50 mM Tris–HCl, pH 7.5, 10 mM MgCl2, 1 mM DTT and 50 mM NaCl. The mixtures were co-incubated at room temperature for 30 min and then directly subjected to 5% native Polyacrylamide Gel Electrophoresis containing 0.5 × Tris-borate-ethylenediaminetetraacetic acid (EDTA) buffer. Electrophoresis was performed at 150 V. Images were acquired by Typhoon Scanner (GE Healthcare).
Footprinting assay
For footprinting assay, the Ms5860p DNA fragment was re-amplifed with the FITC-labeled reverse primer. The purified DNA fragment was added to the reaction mixture (containing various amounts of HpoR) at room temperature for 30 min as in EMSA. All of the mixtures were treated with DNaseI (1 unit, Fermentas) (Fermentas China Co., Ltd, Shenzhen, China) at 37°C for 2 min 30 s as described previously (42). The results were analyzed with Applied Biosystems 37030XL DNA analyzer (manufactured by Tsingke Company, Wuhan, China).
Chromatin immunoprecipitation assay
The chromatin immunoprecipitation (ChIP) was performed as described previously (43). Mycobacterium smegmatis cells were grown to mid-logarithmic phase in 7H9 medium, fixed with 1% formaldehyde and stopped with 0.125 M glycine. Then the cross-linked cells were harvested and resuspended in 1 ml of TBSTT (TBS, 0.2% Triton X-100, 0.05% Tween-20). The sample was sonicated on ice. The supernatant extract was collected by centrifugation. The special antibodies or preimmune serum were added into the sample extracts under rotation for 3 h at 4°C. The complexes were immunoprecipitated with 20 μl 50% proteinA-agarose for 1 h at 4°C. The immunocomplexes were recovered by centrifugation and resuspended in 100 μl TE (20 mM Tris–HCl, pH 7.8, 10 mM EDTA, 0.5% SDS). Then the cross-linking was reversed for 6 h at 65°C. The DNA samples of the input and ChIP were purified and analyzed by PCR. A pair of primers (5′-GATCGTCAGGTCCCGCAACTCCT CGA CATGC-3′ and 5′-GCTGGACACATGTCCAAGTTAGCCGAGCGCG-3′) was used for PCR analysis.
Quantitative PCRs analysis
Quantitative PCRs (qPCRs) were performed using a 25 μL reaction mixture with the following ingredients: 10 μl 2 × SYBR qPCR Mix kit (Aidlab, China), 200 nM concentration of Ms5860p-specific primer pairs (5′-GATCGTCAGGTCCCGCAACTCCT CGA CATGC-3′ and 5′-GCTGGACACATGTCCAAGTTAGCCGAGCGCG-3′), and 2 μl volume of the immunoprecipitated and purified DNA samples from the ChIP assay. Each reaction was performed in triplicate. Amplification and detection of the Ms5860p were performed with CFX96 instrument (BIO-RAD) using the following protocol: 95°C for 5 min, followed by 40 cycles of 95°C for 15 s, 60°C for 15 s and 72°C for 45 s, melt curve, 55–99°C, 0.5°C/10 s, 25°C for 5 min. The data were analyzed with Bio-Rad CFX Manager Version 2.1. Amplification specificity was assessed using melting curve analysis. The relative quantity of Ms5860p in ChIP was normalized to the quantity of Ms5860p in input. The degree of change in relative quantity of Ms5860p was calculated using the 2−△△Ct method. For statistical analysis, two-tailed Student’s t-tests were performed.
β-galactosidase activity assays
β-galactosidase activity experiments were performed in M. smegmatis by constructing operon-lacZ fusions based on the expression vector of pMV261 (44). Target and control promoters were cloned into pMV261 backbone and then the reporter gene lacZ was cloned behind the promoters. The plasmids were transformed into the hpoR-knockout strain and the wild-type M. smegmatis strain, respectively, to obtain their corresponding recombinant reporter strains. All recombinant strains were grown in 7H9 medium at 37°C until log phage. For the HpoR de-repression assays under oxidative stress, the M. smegmatis strains were grown overnight at 37°C in 7H9 medium until log phase, then cells were continued to culture with addition of 10 mM H2O2 for 4 h. The bacterial cells were harvested and washed using phosphate-buffered saline (PBS). β-Galactosidase measurements were performed as described previously (45).
Isolation and detection of c-di-GMP in M. smegmatis
Isolation of c-di-GMP in M. smegmatis was performed according to the procedures previously (46) with modifications. Mycobacterium smegmatis cells were grown overnight at 37°C in 7H9 medium until log phase. Cells were continued to culture with addition of 10 mM H2O2 for 4 h. Then the cells were harvested and washed using PBS. To extract intracellular c-di-GMP, the samples were incubated for 20 min on ice with 0.6 M HClO4 and centrifuged at 7000 rpm for 10 min. The supernatant was neutralized to pH 6.0 with 5 M K2CO3 and was recentrifuged to remove the precipitated KClO4. The resultant supernatant was condensed to 0.5 ml. The lyophilized samples were resuspended and injected into C-18 columns for High Performance Liquid Chromatography (HPLC) analysis. The relationship of standard curve between c-di-GMP concentration and peak area was acquired through a series of concentration gradient standard c-di-GMP. The sample peak area, derived from Msm/WT and Msm/WT (10 mM H2O2), was put into the standard curve equation and the c-di-GMP concentration of sample could be calculated. Each sample peak area was determined independently for three times.
Cross-linking assays for nucleotide-HpoR interaction
The cross-linking assay was performed according to previously published procedures (16). In brief, 15 μM proteins were incubated for 10 min on ice in diguanylate cyclase reaction buffer (50 mM Tris–HCl, pH 7.5, 50 mM NaCl, 5 mM MgCl2) together with 5 μM radioactive-labeled c-di-GMP or c-di-AMP. And the competing cold ligands c-di-GMP, c-di-AMP and GTP were added at the same time with radioactive-labeled c-di-GMP. Following ultraviolet irradiation for 30 min, reaction samples were directly subjected to a 12% w/v sodium dodecyl sulphate-polyacrylamide gel electrophoresis (SDS-PAGE). Electrophoresis was performed at 150 V and at room temperature until bromophenol blue reached the bottom part of the gel. Radioactive gels were exposed to a storage phosphor screen (GE Healthcare) overnight. Images were obtained using Typhoon Scanner (GE healthcare).
Isothermal titration calorimetry (ITC) analysis
ITC assays were carried out on a Nano ITC Low Volume isothermal calorimeter (TA Instruments, New Castle, DE, USA) according to a previously described procedure (16). The HpoR protein was dialyzed in the buffer (20 mM Tris-base, 100 mM NaCl, 5 mM MgCl2, pH 7.5). All buffers were degassed before use. Both HpoR and the solution containing c-di-GMP were added into the sample cell (190 μl) and the syringe (50 μl), respectively. There were 25 injections per experiment, and the stirring rate was 250 rpm. Data were recorded automatically and subsequently analyzed using the NanoAnalyze Software provided by the manufacturer. In control experiment, the c-di-GMP solution was titrated into the buffer in sample cell to obtain the heat of dilution. The value of the heat of dilution was then subtracted from the experimental curve in the final analysis. All the titration curves were fitted to the independent-site binding model.
Surface plasmon resonance (SPR) analysis
Surface plasmon resonance (SPR) analysis was carried out in a Biacore 3000 instrument (GE Healthcare) with Streptavidin chip (SA) sensor chips. The assays were performed at 25°C. For the effect of c-di-GMP on DNA-binding activity of HpoR, Biotin-labeled Ms5860p was immobilized onto SA chips. The different concentrations of c-di-GMP (0.5–32 μM) were incubated with a fixed concentration of HpoR (130 nM) for 30 min at 4°C, then the complex was passed over the chips. An overlay plot was produced using BIAevaluation 3.1 software to depict the effect of c-di-GMP on DNA-binding activity of HpoR.
Construction of the gene deletion mutant M. smegmatis and M. bovis strains and Southern blot analysis
A pMind-derived (47) suicide plasmid carrying a hygromycin resistance gene was constructed and a sacB-lacZ gene was inserted to confer sensitivity to sucrose as a negative selection marker. Knockout of the hpoR was performed as described previously (41). The primers for genes knockout were shown in Supplementary Table S2. Deletion of the gene was confirmed by Southern blot analysis as described previously (48). The probes consisted of a 300-bp fragment of the downstream region of the gene amplified using appropriate primers.
RESULTS
c-di-GMP levels affect the H2O2 resistance of M. smegmatis
Our studies originated from a determination of the level change of c-di-GMP in the mycobacterium upon oxidative stress. HPLC analysis showed that the content of the intracellular c-di-GMP of M. smegmatis significantly improved (7.419 ± 0.57 μM) under the stress of 10 mM H2O2 (Figure 1A, lower panel, and 1B) when compared with that without stress (4.575 ± 0.32 μM) (Figure 1A, middle panel, and 1B), indicating that H2O2 stress can induce c-di-GMP accumulation in M. smegmatis.
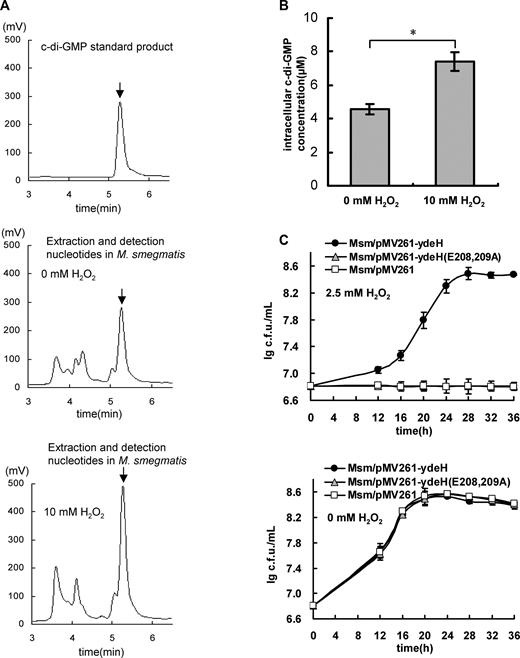
High level of c-di-GMP enhances the antioxidant ability of Mycobacterium smegmatis. (A) Assays for the concentration of the intracellular c-di-GMP in M. smegmatis upon H2O2 stress. Mycobacterial nucleotides were extracted. The samples were separated by reverse-phase HPLC and assayed in buffer A on C-18 columns. The purified c-di-GMP is used as positive control (the upper panel) and the characteristic peak for c-di-GMP is indicated by arrows. The peak for the sample derived from the Msm/WT is shown in the middle panel and the sample from the Msm/WT stressed by 10 mM H2O2 shown in the lower panel. (B) Determination of the concentration of intracelluar c-di-GMP in M. smegmatis. An asterisk (*) in figure represents significant difference(P ≤ 0.05, two-tailed Student’s t-test) between two groups. Error bars represent the variant range of the data derived from three biological replicates. (C) Assays for the effect of c-di-GMP levels on the growth of M. smegmatis. The mycobacterial strains, Msm/pMV261-ydeH, Msm/pMV261-ydeH (E208, 209A) and Msm/pMV261, were grown in 7H9 medium (the lower panel) or supplemented with 2.5 mM H2O2 (the upper panel) and the growth curves were determined. Error bars represent the variant range of the data derived from three biological replicates.
We further investigated the relationship of a high level of the intracellular c-di-GMP with the H2O2 resistance of the mycobacterium. Using a previously reported strategy (26), we overexpressed an E. coli-derived diguanylate cyclase YdeH (49), which has a high activity of c-di-GMP synthesis in M. smegmatis, and constructed a mycobacterial strain (Msm/pMV261-ydeH) with a high level of c-di-GMP (26). Strikingly, the Msm/pMV261-ydeH strain grew well under H2O2 stress (Figure 1C, upper panel). By contrast, the control strain (Msm/pMV261-ydeH (E208,209A)) expressing the mutant gene ydeH (E208, 209A), which lost the diguanylate cyclase activity, or the wild-type strain with an empty pMV261 vector was sensitive to 2.5 mM H2O2 (Figure 1C, upper panel). Essentially, no obvious growth difference between these two mycobacterial strains was observed if no H2O2 stress (Figure 1C, lower panel).
H2O2 stress can induce c-di-GMP accumulation in M. smegmatis and the intracellular c-di-GMP can enhance mycobacterial H2O2 resistance, implying that the oxidative stress would induce the mycobacterial H2O2 resistance. To test this hypothesis, we compared the antioxidant growth ability of the pre-stressed mycobacteria by 10 mM H2O2 with non-stressed bacteria. As shown in Supplementary Figure S1A, the bacterial counts of the pre-stressed Msm/WT were found to be significantly higher than those of non-stressed Msm/WT at all three time points (16, 22 or 28 h) when comparing their growths on the plates containing 3 mM H2O2, indicating that the oxidative stress could induce the mycobacterial H2O2 resistance. This is consistent with our data shown above (Figure 1A–C). Consistently, when overexpressing an E. coli-derived phosphodiesterases YhjH (50), which has a high activity of c-di-GMP degradation and can lead to a lower level of c-di-GMP in bacteria, the recombinant mycobacterial strain (Msm/pMV261-yhjH) obtained an obvious worse growth than the Msm/pMV261 strain under similar H2O2 stressful conditions (Supplementary Figure S1B). These results strongly suggest that oxidative stress can induce c-di-GMP accumulation and enhances the mycobacterial H2O2 resistance.
Taken together, H2O2 stress can induce c-di-GMP accumulation in M. smegmatis, and high level of the intracellular c-di-GMP can remarkably enhance mycobacterial H2O2 resistance.
Expression of the hpoR operon contributes to the mycobacterial H2O2 resistance
To pursue the signaling pathway that c-di-GMP regulates the resistance, we compared the differential gene expression between Msm/pMV261-ydeH and Msm/pMV261-ydeH (E208,209A) strain through transcriptomic assays. As shown in Figure 2A, when comparing the gene expression difference between these two strains by RNA sequencing, a large group of genes was found to be upregulated or downregulated in the Msm/pMV261-ydeH strain. This indicates that c-di-GMP could induce a global expression change of the mycobacterial genes (Supplementary Table S3). Interestingly, a redox gene cluster Ms5855-5865, designated as the hpoR operon (hydrogen peroxide resistance), was integrally induced and its expression was upregulated in the Msm/pMV261-ydeH strain (Figure 2B and Supplementary Table S3).
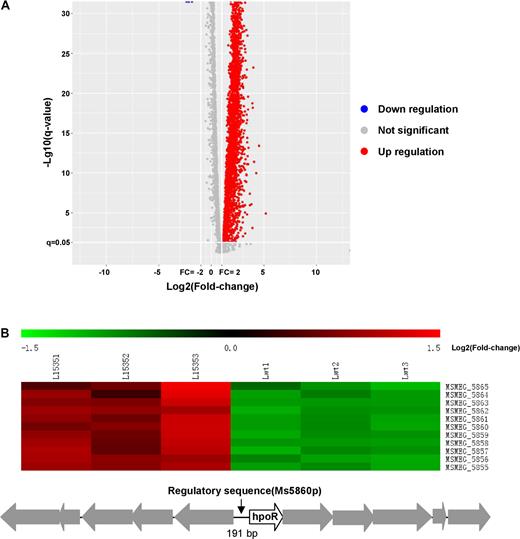
Transcriptomic assays for the effect of c-di-GMP on the gene expression of Mycobacterium smegmatis. (A) The volcanic diagram of gene expression difference between Msm/pMV261-ydeH and Msm/pMV261-ydeH (E208, 209A) strains determined by RNA Sequencing assays. The significantly upregulated genes were shown by red spots. Downregulated and not significant change genes were shown by blue and gray spots, respectively. (B) The heat map of the c-di-GMP-triggered differential expression profile for the hpoR operon (Ms5855-Ms5865 gene cluster). L15351, L15352 and L15353 represent three biological replicates of differentially expressed hpoR operon genes in Msm/pMV261-ydeH strain, respectively. Lwt1, Lwt2 and Lwt3 represent three biological replicates of the those genes in Msm/pMV261-ydeH (E208,209A) strain, respectively. Lower panel shows the schematic of the hpoR operon and its regulatory region.
We constructed a Msm/Ms5860p::hyg strain in which the native upstream regulatory sequence (191 bp) of the hpoR operon was replaced by hygromycin gene (hyg) to investigate whether the regulation of expression of the hpoR operon correlates with the mycobacterial H2O2 resistance. As shown in Figure 3A, the bacterial counts of the wild-type were found to be significantly lower than those of mutant strains at all three time points when comparing the growth of two mycobacterial strains stressed by 3 mM H2O2 for 16, 20 or 24 h, indicating that the Ms5860p could play an important role for the mycobacterial H2O2 resistance. No growth difference between two strains was observed if they grew in the absence of H2O2 stress (Figure 3B). This result indicates that the regulation of the hpoR operon contributes to the mycobacterial ability against oxidative stress.
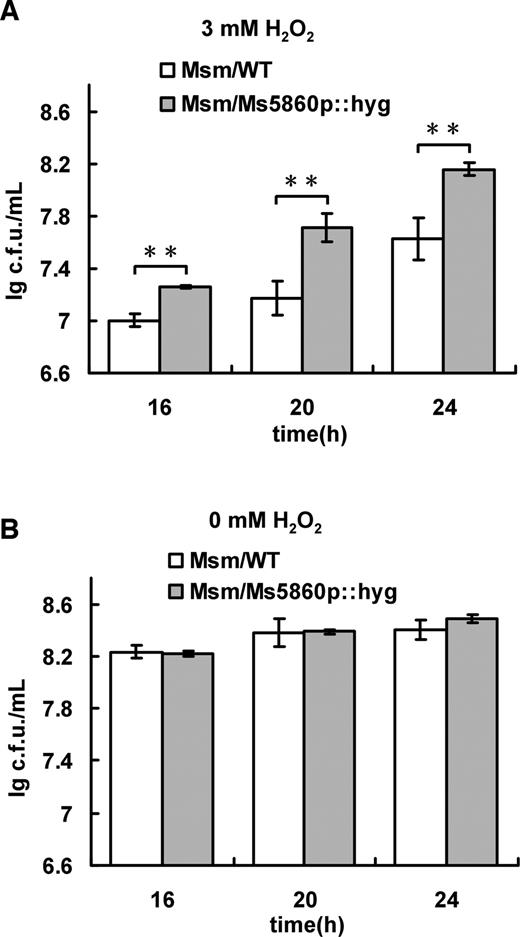
Assays for the effect of H2O2 on the growth of Mycobacterium smegmatis. Both Msm/WT and Msm/Ms5860p::hyg were grown in 7H9 medium (B) and the medium supplemented with 3 mM H2O2 (A), and bacterial counts were determined. All error bars in the figure represent the variant range of the data derived from three biological replicates. The P-values of the relative expression data were calculated by unpaired two-tailed Student’s t-test using GraphPad Prism 5. An asterisk (*) represents significant difference between two groups.
Taken together, c-di-GMP can trigger the expression of the hpoR operon, which plays a critical role for the mycobacterial H2O2 resistance.
HpoR specifically binds with the upstream regulatory sequence of the hpoR operon
We used the regulation of the hpoR operon as an example to further investigate the signaling pathway through which c-di-GMP potentially modulates the defense against oxidative stress in M. smegmatis. Most of the hpoR operon genes encode redox-related enzymes. Ms5860 is a putative regulatory protein, designated as HpoR in the present study, which controls the expression of the hpoR operon. To test this hypothesis, we used a bacterial one-hybrid assay to detect whether HpoR can specifically bind the upstream sequence of the operon. As shown in Figure 4A, the reporter strain containing HpoR and the target DNA (Ms5860p) grew well, but the self-activation control strain containing either HpoR or the DNA alone did not grow on the screening media. This result indicates that HpoR specifically interacts with the upstream sequence of the hpoR operon, Ms5860p.
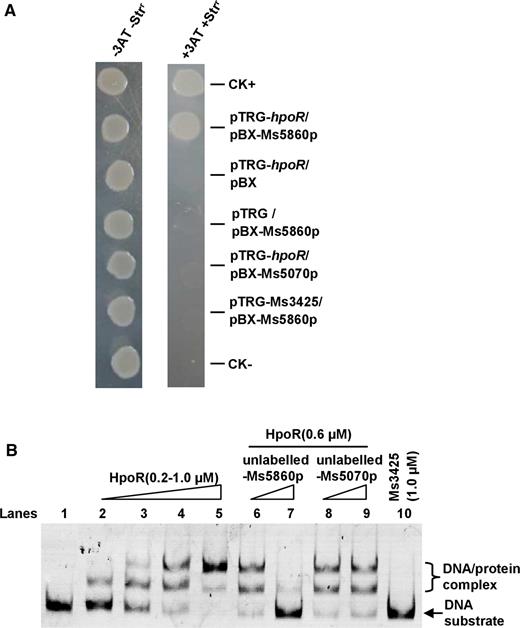
Assays for the interaction between HpoR and Ms5860p. (A) Bacterial one-hybrid assays for the interaction between HpoR and Ms5860p. Co-transformants containing the pBX-Ms5860p/pTRG-hpoR plasmids grew well on the screening medium. The positive control co-transformants containing the pBX-Rv2031/pTRG-Rv3133 grew well on the screening medium, but the negative control strain containing pBX /pTRG plasmids did not. (B) EMSA assays. Specific DNA binding activity of HpoR on Ms5860p promoter DNA was observed when Ms5860p DNA substrate was co-incubated with increasing amounts of HpoR (lanes 2–5). The unlabeled Ms5860p (lanes 6–7) could compete with the labeled Ms5860p to bind with the HpoR protein, but Ms5070p could not (lanes 8–9). Ms3425 was used as a negative control protein (lane 10).
An EMSA was performed for further confirmation, as shown in Figure 4B. A stepwise increase in the amount of shifted DNA was clearly observed with increasing amounts of the HpoR protein (0.2–1 μM) in the reactions (lanes 2–5). Addition of unlabeled Ms5860p at 30-fold (Figure 4B, lane 7) excesses to reaction mixtures competitively inhibited the binding of FITC-labeled Ms5860p to HpoR. In contrast, an unlabeled Ms5070 promoter DNA, used as a negative control DNA, did not compete for binding of HpoR with labeled Ms5860p (Figure 4B, lane 9). In addition, no DNA-binding activity could be observed for the negative protein Ms3425 under the same experimental conditions (Figure 4B, lane 10).
Therefore, our results showed that HpoR could specifically bind with the upstream regulatory sequence, Ms5860p, of the hpoR operon.
HpoR recognizes a palindrome sequence motif
A DNase I footprinting assay was used to further map the potential motif within the Ms5860p fragment recognized by HpoR. As shown in Figure 5A, when the DNA substrate Ms5860p was exposed to DNase I, two regions containing CGCTGGACACATGTCCAAGT and AGCCAGACAGCTGTCCAGGA were clearly protected by HpoR if an increasing amounts of the HpoR protein (0.5–3 μM) was added into the reactions. Strikingly, both binding regions contained a similar core sequence motif (G/AGACANNTGTCC), in which the inverted repeats were almost intact. A palindromic motif formed by each inverted repeat and separated from each other by 2 nt was found in the core sequence. This suggests that HpoR recognizes a palindrome sequence motif. Further EMSAs confirmed that a 12-bp motif (G/AGACANNTGTCC) in these regions was required for recognition by HpoR. Three DNA substrates, designated as Ms5860p1, Ms5860p2 and Ms5860p3, were produced to characterize the importance of the palindromic motif for the recognition by HpoR. As shown in Figure 5B, HpoR could not bind with the Ms5860p3 fragments in which the two inverted repeats were mutated. In contrast, the protein was able to bind to an Ms5860p1 or Ms5860p2 substrate in which retained an intact inverted repeat.
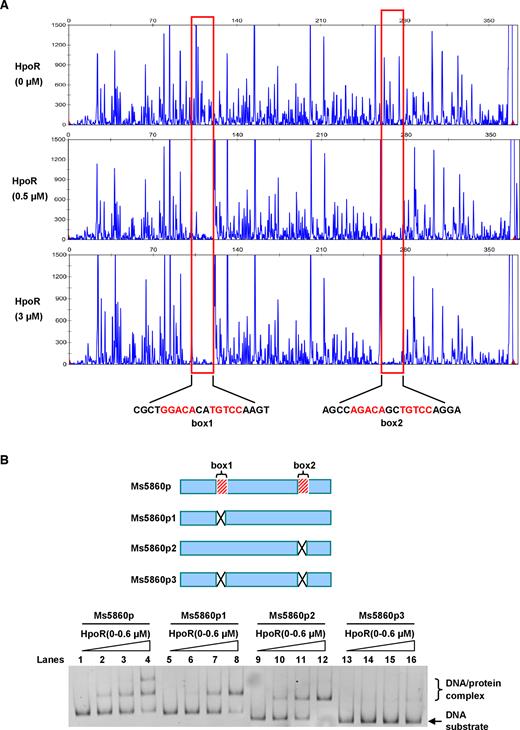
Assays for mapping the motif sequence recognized by HpoR. (A) FITC labeled primer-based DNaseI footprinting experiments. Protection of the Ms5860p promoter DNA against DNaseI digestion by increasing amounts of HpoR (0, 0.5 and 3.0 μM) was evaluated. The sequences of the protected regions on the non-coding strand are marked in the figures. (B) EMSA assays for the importance of two motifs for the binding of HpoR. Two DNA-binding motifs, box1 and box2, locating on the genome in Mycobacterium smegmatis are indicated (upper panel). Three mutant DNA substrates derived from the Ms5860p promoter DNA, designated as p1(the box1 deletion), p2(the box2 deletion) and p3(both the box1 and box2 deletion), were synthesized and labeled. Each of these different DNA substrates was co-incubated with 0–0.6 μM HpoR and loaded on the gel for analysis (lower panel).
Taken together, our findings indicate that HpoR recognizes a 12-bp motif palindrome sequence motif (G/AGACANNTGTCC) situated at the upstream regulatory region of the hpoR operon.
HpoR negatively regulates the expression of the hpoR operon
We next constructed a series of lacZ alone or promoter-lacZ co-expression plasmids and a hpoR-deleted mutant strain (Supplementary Figure S2A and B) to examine the regulatory effect of HpoR on the gene expression by β-galactosidase activity assays. As shown in Figure 6A, the strong promoter hsp60 strikingly promoted the expression of lacZ in both wild-type and hpoR-deleted M. smegmatis strains compared with the non-promoter lacZ plasmid, indicating that the report system worked well. Strikingly, the expression of lacZ was significantly upregulated in the hpoR -deleted mutant M. smegmatis strains compared with the wild-type strains. In comparison, the expression of lacZ was remarkably downregulated in the hpoR-overexpression strains. However, there was no significant difference between the wild-type and mutant or complementary strains observed in the expression of lacZ when a negative control, Ms5860p3 in which the palindrome sequence motif has been mutated, was used as promoter. Taken together, these indicate that HpoR acts as an inhibitor and negatively regulates hpoR operon expression. This conclusion was also be confirmed by our further transcriptomic assays (Supplementary Figure S3).
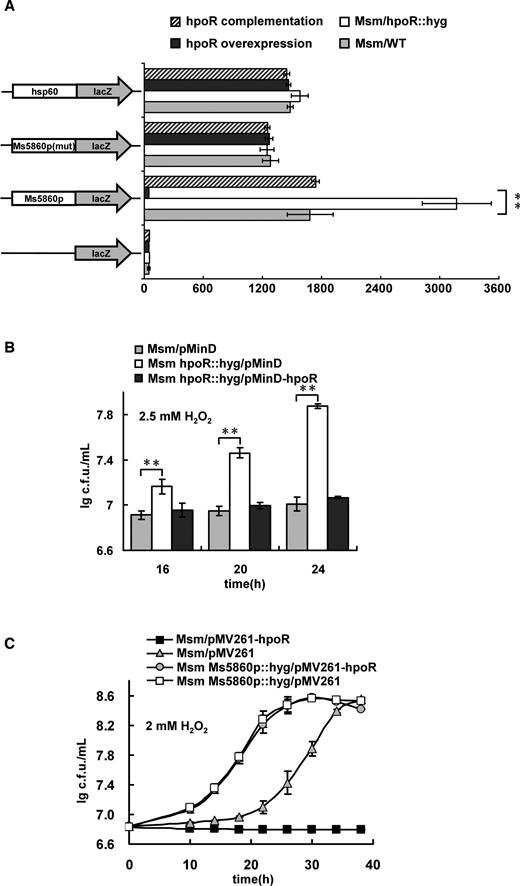
Assays for the negative regulations of HpoR on the hpoR operon genes and on the H2O2 resistance of Mycobacterium smegmatis. (A) β-galactosidase activity assays. The effect of HpoR on the gene expression was assayed by constructing Ms5860p-lacZ plasmid. The activity of β-galactosidase was further examined in the wild-type M. smegmatis strain (Msm/WT), hpoR knock out strain (Msm/hpoR::hyg), hpoR overexpression strain (Msm/pMV261-hpoR) and hpoR complementation strain (Msm hpoR::hyg/pMV361-hpoR). The data were presented as Miller units (right panel). Left column: schematic representation of each clone used to generate recombinant strains. For statistical analysis, two-tailed Student’s t-tests were performed using a P-value of ≤ 0.01. Null promoter-lacZ, hsp60-lacZ and Ms5860p mutant (Ms5860p3) were used as controls. The values presented were the averages of three independent biological experiments. The P-values of the results (≤0.01) are indicated by two asterisks (**) on top of the column in the figure. (B) HpoR negatively regulates the H2O2 resistance of M. smegamatis. These mycobacterial strains Msm/pMinD, Msm hpoR::hyg/pMinD and Msm hpoR::hyg/pMinD-hpoR were grown in 7H9 medium supplemented with 2.5 mM H2O2. Two asterisks (**) in figure represents significant difference (P ≤ 0.01, two-tailed Student’s t-test) between two groups. Error bars represent the variant range of the data derived from three biological replicates. (C) The mycobacterial strains Msm/pMV261-hpoR, Msm/pMV261, Msm Ms5860p::hyg/pMV261-hpoR and Msm Ms5860p::hyg/pMV261 were grown in 7H9 medium supplemented with 2 mM H2O2. Representative growth curves are shown. Error bars represent the variant range of the data derived from three biological replicates. Overexpressing hpoR in Msm/pMV261 strain inhibited the mycobacterial growth under H2O2 stress. But stimulation, not inhibition, was observed for the mycobacterial growth if overexpressing hpoR in the Msm Ms5860p::hyg/pMV261-hpoR strain, in which the upstream regulatory sequence (Ms5860p) of the hpoR operon has been knocked-out.
HpoR negatively regulates mycobacterial H2O2 resistance
HpoR inhibited the expression of the hpoR operon, which is correlated with mycobacterial H2O2 resistance (Figures 3A and 6A), suggesting that HpoR could regulate mycobacterial growth under H2O2 stress. This assumption could be confirmed as the hpoR-deleted mutant strain grew well than that of the wild-type strain under 2.5 mM H2O2 stress (Figure 6B). And when expressing hpoR gene in the hpoR-deleted M. smegmatis strain, the complementary strain re-obtained a similar growth compared with the wild-type strain (Figure 6B). Strikingly, the growth inhibition by hpoR-overexpression was found to depend on the regulatory sequence Ms5860p of the hpoR operon, as indicated that the inhibition could not be observed if hpoR was overexpressed in the Msm/Ms5860p::hyg strain (Figure 6C). More interestingly, when comparing the growths of Ms5860p::hyg/pMV261-HpoR and Ms5860p::hyg/pMV261 strains under the H2O2 stress, no significant growth difference between these two recombinant strains could be observed under our experimental conditions (Figure 6C), indicating that the expression of HpoR in the mutant lacking the Ms5860 promoter does not produce an additional protection for the mutant strain.
Therefore, HpoR negatively regulates mycobacterial H2O2 resistance and its regulation depends on the upstream regulatory sequence of the hpoR operon.
Effect of c-di-GMP on the mycobacterial H2O2 resistance depends on the promoter sequence of the hpoR operon
We further confirmed that the effect of c-di-GMP on the mycobacterial resistance to H2O2 depended on the regulatory sequence Ms5860p of the hpoR operon. As shown in Supplementary Figure S4, consistent with the observation shown in Figure 6C, the Msm/Ms5860p::hyg strain demonstrated a resistance to H2O2 stress as it lacks a functional Ms5860p promoter, which results in the de-repression of HpoR on the hpoR operon. However, when overexpressing the wild-type ydeH or its mutant gene in the promoter mutant strain, no significant growth difference under the H2O2 stress could be observed for these two mycobacterial strains. This is strikingly different from the observation shown in Figure 1C, in which Msm/pMV261-ydeH, a strain derived from YdeH overexpression in the wild-type M. smegmatis, obtained an obviously better growth than the control strain (Msm/pMV261-ydeH (E208,209A)) under a similar H2O2 stress.
Therefore, our data suggest that the regulatory effect of c-di-GMP on the mycobacterial H2O2 resistance significantly depends on the upstream regulatory sequence of the hpoR operon.
c-di-GMP specifically interacts with the HpoR protein
Some regulators such as OxyS (51) have been reported to directly sense H2O2 and regulated bacterial antioxidant activity. Unfortunately, we showed that HpoR did not sense H2O2 directly and its DNA-binding activity was not affected by H2O2 (Supplementary Figure S5). Alternatively, both c-di-GMP and HpoR could significantly affect expression of the hpoR operon genes which is required for mycobacterial H2O2 resistance, suggesting that c-di-GMP signaling would be directly delivered to the HpoR protein. A cross-linking assay confirmed this hypothesis. As shown in Figure 7A, a strong autoradiograph signal corresponding to HpoR bound to radioactively labeled c-di-GMP on a PAGE (Figure 7A, lane 2), indicating that HpoR can bind with c-di-GMP. Addition of unlabeled c-di-GMP at 10-, 30- and 60-fold excess to the reaction mixtures competitively inhibited the binding of HpoR to [32P]-c-di-GMP (Figure 7A, lanes 3–5), indicating specificity of HpoR binding to c-di-GMP. A negative control nucleotide, either GTP or c-di-AMP, did not alter the binding of HpoR to c-di-GMP even at a 60-fold higher concentration (300 μM) (Figure 7A, lanes 6 and 7). HpoR only weakly bind with c-di-AMP molecules under the same conditions (Figure 7A, lane 8). In addition, a previously reported receptor protein LCN2 binds c-di-GMP well (Figure 7A, lane 1), but an unrelated protein, Rv2764c, did not bind with c-di-GMP, as no corresponding signal was observed on the gel (Figure 7A, lane 9). These results indicate that HpoR could specifically recognize c-di-GMP.
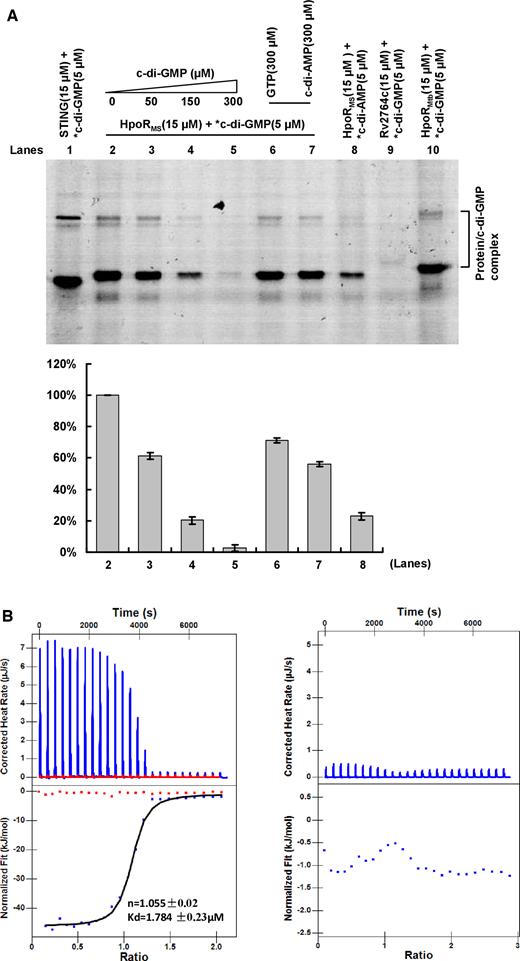
Assays for the specific interaction between HpoR and c-di-GMP. (A) UV cross-linking assay. STING was used as a positive control (lane 1). A competitive experiment was carried out by addition of unlabeled and increasing amounts of c-di-GMP to the reaction mixtures containing 15 μM HpoR and 5 μM labeled c-di-GMP (lanes 2–5). GTP and c-di-AMP were used as negative control molecule (lanes 6–8). Rv2764c was used as a negative control protein (lane 9). The ortholog protein HpoRMtb in Mycobacterium tuberculosis was also detected (lane 10). After irradiating by UV for 30 min, reaction samples were assayed on a 12% (w/v) SDS-PAGE. The radioactive gel was exposed to a storage phosphor screen (GE Healthcare). Radioactive-labeled nucleotides are indicated by an asterisk (*). The *c-di-GMP–HpoR complex was quantified in the lower panel. (B) ITC assays for the specific interaction between HpoR and c-di-GMP. Original titration data and integrated heat measurements are shown in the upper and lower plots, respectively. The solid line in the bottom panel represents the best fit to a one-site binding model of the interaction of HpoR with c-di-GMP (the left panel). c-di-AMP was used as a negative control. No interaction between HpoR and c-di-AMP was observed (the right panel).
The specific interaction between HpoR and c-di-GMP was also confirmed by a further isothermal titration calorimetry (ITC) assay. Figure 7B (left panel) shows the raw data for titration of c-di-GMP against HpoR and indicates that the interaction is exothermic. The integrated heat measurements were shown in the lower panel of the figure. The binding stoichiometry between c-di-GMP and HpoR was 1:1 (n = 1.055 ± 0.02). The binding affinity of the interaction (Kd) was 1.784 ± 0.23 μM, which is comparable to the reported Kd values of several other transcriptional factor receptors (23,26). In contrast, no specific binding with HpoR was observed when a negative control molecule, c-di-AMP, was conducted for a similar assay under the same conditions (Figure 7B, right panel).
Taken together, our results showed that HpoR could specifically interact with c-di-GMP and HpoR is a novel c-di-GMP receptor transcription factor.
c-di-GMP mediates the DNA-binding activity of HpoR both in vitro and in vivo in M. smegmatis
A direct interaction between c-di-GMP and the regulator HpoR exists, suggesting that the second messenger could affect the DNA-binding activity of HpoR. An EMSA was used to test this hypothesis. As shown in Figure 8A, a corresponding increase in the amounts of shifted DNA substrates was clearly observed as an increasing amounts of c-di-GMP (9–270 μM) was added into the reactions (Figure 8A, lanes 3–7). This indicated that low concentration of c-di-GMP stimulates the DNA-binding activity of HpoR in a concentration-dependent manner. Interestingly, the amounts of shifted DNA substrates deceased as the amount of c-di-GMP added into the reactions was increased from 450 to 900 μM (Figure 8A, lanes 8–11). This indicates that c-di-GMP inhibits the DNA-binding activity of HpoR at its high concentrations.
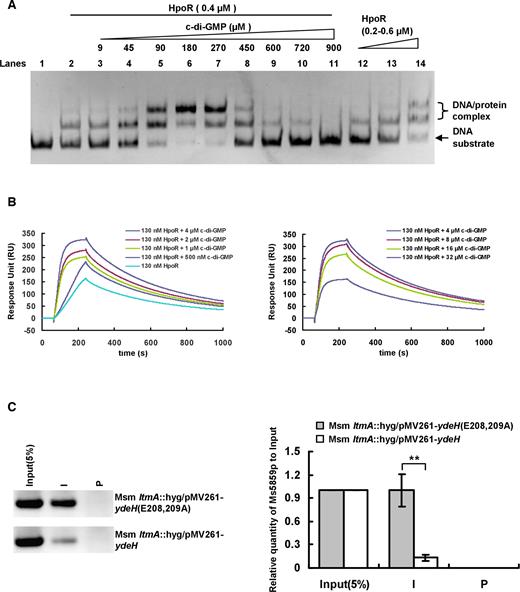
High level of c-di-GMP de-represses the negative regulation of HpoR in vitro and in vivo in Mycobacterium smegmatis. (A) EMSA assays for the effect of c-di-GMP on DNA-binding activity of HpoR in vitro. The concentration of HpoR was immobilized and the increasing amounts of c-di-GMP (9–900 μM) (lanes 3–11) were added into the reactions and incubated for 30 min. Then the DNA substrate was added into the mixture and incubated for 30 min. (B) SPR assays for the regulatory effect of c-di-GMP on DNA-binding activity of HpoR. The biotin-labeled Ms5860p was immobilized on the surface of SA chip and different concentrations of c-di-GMP were incubated with a fixed concentration of HpoR (130 nM) was passed over the chip. (C) ChIP assays for the effect of c-di-GMP on the intracellular DNA-binding activity of HpoR in M. smegmatis. The Input (5%) indicated that the supernatant of disrupted cells was diluted to 5% and used as the template for PCR. ChIP using preimmune (P) or immune (I) sera raised against HpoR. These experiments were quantified using qPCR (the right panel). The P-values of the relative expression data were calculated by two-tailed Student’s t-test using GraphPad Prism 5. Two asterisks (**) in figure represent significant difference (P ≤ 0.01) between two groups.
In the experiment shown in Figure 1, we have found that the content of the intracellular c-di-GMP of M. smegmatis could rise to ∼8 μM under the H2O2 stress, suggesting that this physiological concentration of c-di-GMP would regulate the DNA-binding activity of HpoR in M. smegmatis. To address this issue, we conducted a further SPR assay to examine the dynamic interaction between HpoR and DNA in their low concentrations and re-determined the regulatory effect of c-di-GMP on the interaction. As shown in Figure 8B, when 130 nM HpoR was passed over the DNA substrate immobilized SA chip, a response of ∼150 RU could be observed. When an increasing amount of c-di-GMP (0.5–4.0 μM) was further added together with 130 nM HpoR and the mixture was passed over the chip, a corresponding increase in response was clearly observed (Figure 8B, left panel). Most interestingly, the response deceased as the added amount of c-di-GMP was increased from 8 μM to 32 μM (Figure 8B, right panel), indicating that 8–32 μM c-di-GMP can inhibit the DNA-binding activity of HpoR. This concentration range is very close to the determined content of the intracellular c-di-GMP in M. smegmatis (Figure 1). Therefore, our results suggest that HpoR possesses a unique DNA-binding characteristic in responding to the c-di-GMP signal.
We utilized ChIP assays to further assess the effect of high level of c-di-GMP on the DNA-binding activity of HpoR in the cell of M. smegmatis. LtmA has been characterized as the first c-di-GMP receptor regulator in mycobacteria (26). To suppress the interference of LtmA, we expressed ydeH in the ltmA-deleted M. smegmatis strain to construct a high level of the c-di-GMP strain, and expressed ydeH(E208,209A) to construct a control strain. As shown in Figure 8C, HpoR could precipitate ∼7-fold higher of the upstream regulatory DNA fragments of the hpoR operon from the ydeH(E208,209A)-overexpressing strain than in the ydeH-overexpression strain (Figure 8C). This result implies that high level of c-di-GMP can remarkably inhibit the intracellular DNA-binding activity of HpoR in M. smegmatis, which is consistent with our conclusion obtained from the in vitro experiments above. Furthermore, we could clearly confirm that H2O2 stress obviously decreased the DNA-binding activity of HpoR in the cell of M. smegmatis (Supplementary Figure S6) since the H2O2 inducing c-di-GMP accumulation in M. smegmatis (Figure 1A, middle panel, and 1B). This is also consistent with our data shown above (Figure 8C).
Taken together, c-di-GMP modulates the DNA-binding ability of HpoR both in vitro and in vivo in M. smegmatis.
H2O2 stress induces the de-repression of HpoR on the hpoR operon expression
H2O2 stress induces c-di-GMP accumulation in M. smegmatis (Figure 1). High level of the c-di-GMP inhibits the DNA-binding activity of HpoR and HpoR negatively regulates the expression of the hpoR operon (Figures 6 and 8). These data imply that H2O2 stress can de-repress the negative regulation of HpoR on the hpoR operon expression. We further utilized a similar β-galactosidase activity experiment as above to examine this assumption. As shown in Supplementary Figure S7A and B, upon the induction of 5–10 mM H2O2, the expression of lacZ under Ms5860p was significantly upregulated in M. smegmatis compared with that in the absence of the stress induction. In comparison, there was no significant difference between the stress induction and no stress observed for the expression of lacZ when a negative control, Ms5860p3, was used as promoter. These indicate that H2O2 stress can de-repress the expression of the hpoR operon in an Ms5860p-dependent way. This is consistent with our data shown above (Figures 1, 6 and 8).
The hpoR operon and its regulation on the H2O2 resistance are conserved in mycobacteria
The hpoR operon contributes to the resistance of M. smegmatis to H2O2, while HpoR, a c-di-GMP-responsive regulator, negatively affects the role of the hpoR operon. Interestingly, the genomes of both the operon (Figure 9A) and HpoR (Figure 9B) are highly conserved among several important mycobacterial species, including M. tuberculosis and Mycobacterium bovis Bacillus Calmette-Guerin Vaccine (BCG) which is the most widely used vaccine against TB. The amino acid sequences of Mtb HpoR and BCG HpoR, encoded by Rv0767c and BCG0819c, respectively, are identical (Figure 9B), and the upstream sequences of the two operons also contain the conserved motif (GGACANNTGTCC) for the recognition of HpoR (Supplementary Figure S8A). Furthermore, Mtb HpoR (Rv0767c) showed a good binding ability for c-di-GMP (Figure 7A, lane 10). Interestingly, a high level of c-di-GMP could improve the H2O2 resistance of M. bovis BCG (Figure 9C). To further determine if the functions of the operon and HpoR are conserved, we produced a hpoR-deleted M. bovis BCG strain (Supplementary Figure S8B and C). As shown in Figure 9D, the mutant strain demonstrated good H2O2 resistance compared with the wild-type M. bovis BCG strain. This result indicates that M. bovis BCG HpoR negatively regulates the H2O2 resistance, which is similar to the result obtained from M. smegmatis.
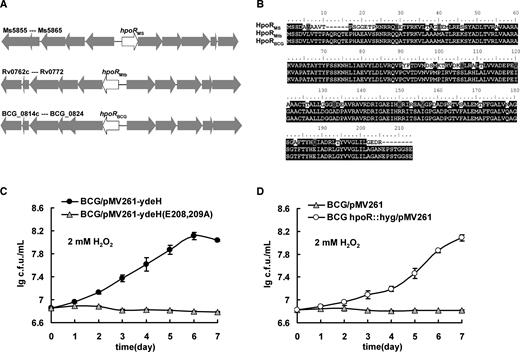
Assays for the conservation of HpoR and its regulation on the antioxidant activity in Mycobacterium bovis. (A) Schematic representation of the conservation for the hpoR operon in the genomes of Mycobacterium smegmatis, Mycobacterium tuberculosis and M. bovis. (B) The amino acid sequence alignment of HpoR. (C) Assays for the stimulating effect of high level of c-di-GMP on the growth of M. bovis under H2O2 stress. Error bars represent the variant range of the data derived from three biological replicates. (D) Assays for the regulation of HpoR on the growth of M. bovis under H2O2 stress. Error bars represent the variant range of the data derived from three biological replicates.
Therefore, the hpoR operon and its regulation on the H2O2 resistance are conserved in several mycobacterial species, including M. tuberculosis and M. bovis BCG.
DISCUSSION
The regulatory network of c-di-GMP has been extensively studied in bacteria, but the whether or not the c-di-GMP signal is linked to the bacterial antioxidant regulation is unclear, and the c-di-GMP responsive transcription factors involved in the regulation of bacterial antioxidant ability also remain to be explored. In the present study, we characterized HpoR as a new c-di-GMP-responsive transcription factor, and found that HpoR participated in regulation of the H2O2 resistance of M. smegmatis. H2O2 stress induces c-di-GMP accumulation in M. smegmatis. c-di-GMP specifically binds with HpoR and high level of c-di-GMP neutralizes the inhibitory role of HpoR, which triggers expression of hpoR operon and enhances the mycobacterial H2O2 resistance. Our findings built a link between c-di-GMP signaling and mycobacterial adaptation to oxidative stress.
With the discovery of various receptors, the regulatory functions of c-di-GMP have become increasingly diverse (6,7,9,11). In recent years, c-di-GMP has been shown to modulate multiple cellular processes, including biofilm formation (5,6), bacterial motility (7,8), virulence (9,10), cell development (11–13) and host immune responses (14–16). In the present study, c-di-GMP was found to promote the mycobacteria to combat oxidative stress through its receptor transcription factor HpoR. The oxidative stress is an unavoidable by-product of aerobic processes and is a common extracellular and intracellular environment cue for bacteria (1,2). However, the mechanisms by which bacteria deliver the stress signal into increased expression of antioxidant enzymes and adaptively trigger antioxidant regulation remain obscure. In the present study, we provided evidences to show that H2O2 stress could boost intracellular c-di-GMP levels in M. smegmatis, although the exact mechanism remains unclear and c-di-GMP further delivered the stress signal to the regulator HpoR for triggering the expression of the hpoR operon that finally exerts the mycobacterial resistance to H2O2 stress. Generally, several typical detoxification enzymes such as catalase and superoxide dismutases tremendously contribute to bacterial antioxidant ability. Unexpectedly, the hpoR operon was found, in the present study, to play a critically important role for the c-di-GMP-triggered antioxidant ability of M. smegmatis, although most of genes in the hpoR operon encode hypothetical redox-related proteins, including oxidoreductase (Ms5857), short chain dehydrogenase (Ms5858 and Ms5862), aldehyde dehydrogenase NAD family protein (Ms5859) and cytochrome P450 (Ms5861, Ms5863 and Ms5864). This implies that there exists a unique mechanism and signaling pathway through which c-di-GMP triggers the bacterial antioxidant regulation in M. smegmatis. Our current data support a model, in which H2O2 stress can induce c-di-GMP accumulation in M. smegmatis. High levels of c-di-GMP de-repress the negative regulation of HpoR on the expression of hpoR operon, which enhances the mycobacterial H2O2 resistance. Therefore, our study found a link between c-di-GMP signaling and mycobacterial antioxidant regulation through the hpoR operon, which extended the second messenger's function to the bacterial defense against environmental stress.
In recent years, several c-di-GMP receptor transcription factors have been successfully characterized in different bacterial species (8,19,23–26). Upon responding to the c-di-GMP signal, these transcription factors positively or negatively affect gene expression as c-di-GMP can directly stimulate or inhibit their DNA-binding activities. For example, c-di-GMP stimulates the DNA-binding activity of LtmA and positively modulates the expression of lipid transport and metabolism genes in M. smegmatis (26). In comparison, c-di-GMP inhibits the DNA-binding activity of Clp, and negatively regulates expression of genes in Xanthomonas campestris (24). However, it seems more complicated for HpoR in responding to c-di-GMP. Different from these previously reported transcription factors, in the present study we found that the stimulation or inhibition of the DNA-binding activity of HpoR by c-di-GMP strongly depended on the concentration of the second messenger, and low concentration of c-di-GMP had an exactly contrary effect on the activity of HpoR to a high concentration of the signal molecule. In particular, we provided data to show that c-di-GMP could clearly regulate the interaction of HpoR with DNA in the range of c-di-GMP concentrations from 0.5 to 32 μM, which is very close to the determined content of the intracellular c-di-GMP in M. smegmatis (Figure 1). Low concentrations of c-di-GMP (0.5–4 μM) stimulated the DNA-binding activity of HpoR, whereas high concentrations (8–32 μM) inhibited the activity. Our findings suggest that, upon the H2O2 stress, HpoR can sense the c-di-GMP signal and plays a regulatory role under physiological conditions in M. smegmatis. The unique characteristic of the c-di-GMP-responsive regulator supports a model, in which HpoR can more easily trigger the mycobacterium to rapidly adapt to environmental changes. In the absence of a stressful induction, low concentration of c-di-GMP in bacterial cells stimulates the DNA-binding activity of HpoR, which thus reduce the unnecessarily additional expression of antioxidant enzymes. By contrast, responding to oxidative stress, a high level of c-di-GMP accumulates in the bacterial cells, which inhibits the DNA-binding activity of HpoR and triggers antioxidant regulation. Therefore, our current study characterized HpoR as a unique and novel c-di-GMP effective transcription factor, which plays an important role for the mycobacterial adaptation to oxidative stress.
Another interesting finding from this study is that both the hpoR operon and its regulation are conserved in several mycobacterial species, including the pathogenic M. tuberculosis and the vaccine strain M. bovis BCG. To survive within host cells, a pathogen or an active vaccine strain must resist various stresses, including oxidative stresses. In the present study, we characterized a novel antioxidant cluster, hpoR operon and a c-di-GMP-responsive regulator conserved both in M. tuberculosis and M. bovis BCG. As expected, when deleting the regulator HpoR to relieve the inhibition of hpoR operon expression, M. bovis BCG becomes more resistant to H2O2 stress than the wild-type strain (Figure 9D). Mycobacterium bovis BCG is the only active vaccine against TB and has been widely used for over 60 years. Enhancing the survival ability of the BCG strain in the host cell would help improve its protective effect and constancy. Our results provide a potential guide for further designing new active BCG vaccine strains in the future.
DATA AVAILABILITY
The RNA-seq data of M. smegmatis were deposited to the Gene Expression Omnibus (GEO) (GSE104978 and GSE108652).
SUPPLEMENTARY DATA
Supplementary Data are available at NAR Online.
ACKNOWLEDGEMENTS
Author’s contributions: Z.-G. H. designed and coordinated the study. W. L., M. L., L. H., J. Z.,Z. X. and J. C. conducted experiments. All authors contributed to the interpretations and conclusions presented. Z.-G. H., and W.L. interpreted results and wrote the manuscript.
FUNDING
National Key R&D Program of China [2017YFD0500300]; National Natural Science Foundation of China [31730005, 31401108, 31670075]; Fundamental Research Funds for the Central Universities [2662016PY090, 2662017PY096]; Chang Jiang Scholars Program (to Z.-G.H.). Funding for open access charge: National Key R&D Program of China [2017YFD0500300].
Conflict of interest statement. None declared.
Comments