-
PDF
- Split View
-
Views
-
Cite
Cite
Hayden Holmlund, Yasuhiro Yamauchi, Victor A Ruthig, Julie Cocquet, Monika A Ward, Return of the forgotten hero: the role of Y chromosome-encoded Zfy in male reproduction, Molecular Human Reproduction, Volume 29, Issue 8, August 2023, gaad025, https://doi.org/10.1093/molehr/gaad025
- Share Icon Share
Abstract
The Y-linked zinc finger gene ZFY is conserved across eutherians and is known to be a critical fertility factor in some species. The initial studies of the mouse homologues, Zfy1 and Zfy2, were performed using mice with spontaneous Y chromosome mutations and Zfy transgenes. These studies revealed that Zfy is involved in multiple processes during spermatogenesis, including removal of germ cells with unpaired chromosomes and control of meiotic sex chromosome inactivation during meiosis I, facilitating the progress of meiosis II, promoting spermiogenesis, and improving assisted reproduction outcomes. Zfy was also identified as a key gene in Y chromosome evolution, protecting this chromosome from extinction by serving as the executioner responsible for meiosis surveillance. Studies with targeted Zfy knock-outs revealed that mice lacking both homologues have severe spermatogenic defects and are infertile. Based on protein structure and in vitro assays, Zfy is expected to drive spermatogenesis as a transcriptional regulator. The combined evidence documents that the presence of at least one Zfy homologue is required for male fertility and that Zfy2 plays a more prominent role. This knowledge reinforces the importance of these factors for mouse spermatogenesis and informs our understanding of the human ZFY variants, which are homologous to the mouse Zfy1 and Zfy2.
Introduction
For many decades, it was thought that the Y chromosome is a gene desert, with Sry as the only valuable gene on this chromosome. Thanks to advances in genomics, we now know that the Y chromosome encodes many genes, some of which are thought to benefit male reproduction while others play roles of broadly expressed regulators of transcription, translation, and protein stability (Bellott et al., 2014). The exact function of many Y chromosome genes is still not well established. Here, we present the story of one mouse Y chromosome gene: zinc finger Y chromosome encoded (Zfy), which was once the center of attention as a potential candidate for the testis-determining factor. When that fame went to another Y gene, Sry, Zfy became quickly forgotten and it has taken more than two decades for it to re-emerge with newly ascribed roles in spermatogenesis.
Zfy as the testis-determining gene?
The Y chromosome was first identified as a sex-determining factor by the American geneticist Nettie Stevens, who observed that when mealworm sperm carrying a small ‘accessory chromosome’ fertilized eggs, only male offspring resulted (McClung, 1902; Stevens, 1905). This chromosome became known as the Y chromosome, and it was subsequently shown to be conserved across marsupials and eutherians, including humans (Painter, 1923, 1925). The importance of proper sex chromosome constitution was clinically demonstrated in the late 1950s. Patients with an XO karyotype were phenotypically female but displayed defects in development, puberty, and fertility, a disorder called Turner syndrome (Ford et al., 1959). Another developmental disorder, Klinefelter syndrome, was observed in individuals with an XXY karyotype, who were phenotypically male but lacked proper sexual development compared to that of XY males (Jacobs and Strong, 1959). Because XO and XX individuals develop as females while XY and XXY individuals develop as males, it was concluded that it is not the X chromosome dosage that decides about sex, but the presence of the Y chromosome. A search then began to identify the testis-determining gene (or genes) on the Y chromosome, called testis-determining factor (TDF) in humans (McKusick, 1975) and testis-determining gene on the Y (Tdy) in mice (Eicher et al., 1982).
The proposed TDF/Tdy was expected to exhibit several specific features (Koopman et al., 2016). It had to be (i) conserved across mammals, (ii) located on the Y chromosome within the smallest sex-determining region identified for humans and mice, (iii) responsible for producing a protein that acts autonomously and controls the activity of other genes, (iv) expressed in somatic cells of the testis at the time of sex determination, and (v) indispensable to male development and, when mutated, lead to a failure of testis growth, resulting in XY female development. Genetic analyses of sex reversed men and women have shown that the presence of a Y-derived 300 kb fragment in XX individuals was enough to induce maleness, while the deletion of 160 kb from the Y of XY individuals results in the development of females (Page et al., 1987). The two regions had a 140 kb overlap which was hypothesized to include the mysterious TDF. Encoded within this region was a newly discovered transcriptional unit that was predicted to encode distinct cysteine- and histidine-rich ‘finger’ regions with the capacity to bind to nucleic acid (Page et al., 1987). Comparison of this new sequence to those of known proteins confirmed that it encoded a putative transcription factor that may regulate the expression of other genes. This factor became known as the zinc finger gene Y-linked (ZFY) protein in humans. Two homologous gene sequences were also present in mice (Zfy1 and Zfy2) and were shown to be included within the sex reversal factor, Sxra (Evans et al., 1982; Singh and Jones, 1982) (Fig. 1). Based on its location on the Y chromosome and the potential to control the activity of other genes, ZFY/Zfy was proposed to be TDF/Tdy (Page et al., 1987; Page, 1988).
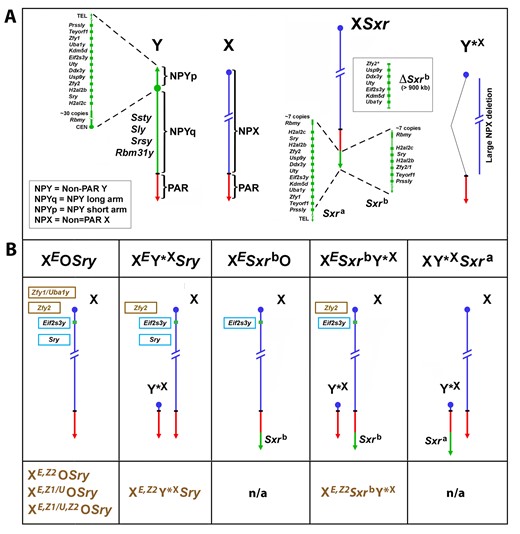
Mouse X and Y chromosomes, variant sex chromosomes, and mouse genotypes with Zfy transgenes. (A) The mouse Y chromosome contains ∼90 Mb of male-specific DNA and ∼0.7 Mb constituting the pseudoautosomal region (PAR) situated at the end of the long arm. The PAR is the region of homology with the X that mediates pairing and recombination between the X and Y in normal males. The remaining nonpairing male-specific part of Y (NPY) contains several genes and gene families. On the short arm (NPYp), there are single-copy genes: Prssly, Teyorf1, Uba1y, Smcy/Kdm5d, Eif2s3y, Uty, Dby/Ddx3y, Usp9y, Sry, duplicated gene Zfy (Zfy1 and Zfy2), duplicated gene H2al2y (H2al2b and H2al2c), and a multi-copy gene Rbmy. The nonpairing region of the long arm (NPYq), representing ∼90% of all NPY, contains mostly repetitive sequences and encodes multiple copies of 5 distinct genes that are expressed in spermatids: Ssty1, Ssty2, Sly, Srsy, and Rbm31y (Soh et al., 2014). Y*X is an X chromosome derivative encoding PAR, X centromere, and near centromeric region. Sxra is a sex reversal variant Tp(Y)1CtSxr-a encoding almost intact NPYp complement but with Rbmy gene family reduced. Sxrb is an Sxra derivative with a 1.3-Mb deletion with removal of the majority of the NPYp gene complement and creation of a Zfy2/1 fusion gene (Zfy2* in ΔSxrb refers to majority of the coding sequence of Zfy2 gene). The Sxra was originally detected on the X chromosome (XSxra) and the breeding scheme to maintain this mutation kept it on X. However, when XSxraY*X males were reproduced by ICSI (Yamauchi et al., 2009), the XSxra and Y*X association at metaphase I via the obligatory crossover within the PAR allowed for creation of both XSxra and Y*XSxra gametes. In consequence, Sxra can be carried on either X or Y*X. Since the Sxrb mutation arose from XSxra, Sxrb is always X-encoded (XSxrb). (B) The mice with Y chromosome deficiencies and transgenic additions; these mice were used to investigate the effects of Zfy loss and rescue in the context of loss of other Y chromosome genes. The X chromosome located Eif2s3y (E) and the autosomally located Sry transgenes are shown in the light blue frames. The Zfy2 transgene (Z2), shown in a brown frame, is located on the X chromosome in the Hrpt locus. The Zfy1 transgene (Z1/U), shown in a brown frame, encodes both Zfy1 and Uba1y as the transgenic line was produced with BAC containing both genes (Royo et al., 2010). The genotype designations without the Zfy1 and Zfy2 transgenes are shown above the diagrammatic representation of sex chromosomes, and those with the Zfy1 and Zfy2 transgenes are shown below them (brown font). Sxra and Sxrb gene content is shown in (A). n/a = mice with transgenic Zfy addition were not produced. The mice shown in (B) were used by the Burgoyne, Turner, and Ward Labs to indirectly ascribe spermatogenic roles to ZFY1 and ZFY (Royo et al., 2010; Vernet et al., 2011, 2012, 2014, 2016a,b; Decarpentrie et al., 2012; Yamauchi et al., 2014, 2015).
Upon further investigation, ZFY/Zfy was disqualified as a TDF/Tdy candidate as it did not meet all the qualities expected from the testis determinant. First, rather than being specifically expressed by fetal gonadal somatic cells at the time of sex determination, human ZFY was found to be ubiquitously expressed (Lau and Chan, 1989; Schneider-Gadicke et al., 1989), while in mice, there were conflicting reports regarding Zfy expression patterns. Initially, Zfy1 and Zfy2 transcripts were found to be confined to the postnatal testis, with no transcripts present in any fetal tissues (Mardon et al., 1989). Later, Zfy1 and Zfy2 transcripts were detected in embryonic tissues, including the developing gonads (Koopman et al., 1989; Nagamine et al., 1990; Zambrowicz et al., 1994). However, Zfy predominance in adult testicular germ cells suggested another role in male reproduction besides sex determination. Second, ZFY has a conserved X chromosome homologue, ZFX, which is also ubiquitously expressed and escapes X chromosome inactivation (Schneider-Gadicke et al., 1989). In mice, Zfy has both a ubiquitously expressed X homologue, Zfx and a testis-specific autosomal homologue, Zfa (Ashworth et al., 1990; Gubbay et al., 1990). Although it remains possible that ZFY/Zfy may play distinct biochemical functions from its X-linked and autosomal homologues, the existence of homologues indicated a general cellular function rather than responsibility for a specific developmental event, like sex determination. Third, although many eutherians have at least one copy of ZFY, the testis-determining Y chromosome in marsupials lack an equivalent homologue. Finally, genomic sequence analysis of four sex reversed XX male and intersex human patients failed to detect ZFY (Palmer et al., 1989). Together, these findings implied that another Y chromosome gene must be responsible for male sex determination. In the early nineties, sex-determining region Y, or Sry, was unequivocally shown to be the mammalian sex-determining gene (Gubbay et al., 1990; Sinclair et al., 1990; Koopman et al., 1991).
Although the lack of a function in sex determination was not indicative of a lack of reproductive function overall, the ZFY gene family disappeared from public interest, and it has taken almost two decades for it to re-emerge with new ascribed roles.
Mice carrying sex chromosome variants and Y-derived transgenes were used as tools in early studies of Zfy function
For many years, progress with assigning spermatogenic functions to Y-specific genes was hindered because conventional approaches for mapping of functions, like positional cloning and classic gene targeting, were not successful. Due to the highly repetitive sequences of the male-specific region of the mouse Y chromosome (MSY; also called nonpairing Y, NPY), the complete sequence and gene composition of the Y chromosome have not been obtained until recently (Soh et al., 2014) (Fig. 1A). Most of what has been known about Y chromosome gene function in the years preceding development of genome editing strategies was derived from deletion mapping and the analysis of deletion associated phenotypes, as well as from a transgene rescue approach, in which missing genes were added back to deletion males. The initial findings, indicative of the spermatogenic function of Zfy, were also all acquired with the use of mouse models with natural mutations, sex chromosome variants, and Y chromosome derived transgenes. The key sex chromosome variants and models used in these studies are shown in Fig. 1B and Table 1. Sex reversal factor, Sxra (Fig. 1A) is believed to have arisen from a duplication of the Y chromosome short arm (Yp), transposition to the Y chromosome pseudoautosomal region (PAR), and translocation to the PAR region of the X chromosome (Cattanach et al., 1971; Evans et al., 1982; McLaren et al., 1988; Roberts et al., 1988). It represents an almost intact Y chromosome short arm (Yp) that encodes most of its genes, including the sex determinant Sry, the spermatogonial proliferation factor Eif2s3y, and the zinc finger Y genes Zfy1 and Zfy2. A spontaneous mutation of Sxra, that removed ∼900 kb of interstitial sequence encoding six genes (Usp9y, Ddx3y, Uty, Eif2s3y, Kdm5d, and Uba1y) and created the Zfy2/1 fusion gene, resulted in a sex reversal factor variant, Sxrb (Burgoyne et al., 1986; Sutcliffe and Burgoyne, 1989) (Fig. 1A). Our prior work has shown that two Y chromosome genes encoded within Sxra, Sry, and Eif2s3y, when added as transgenes to mice with a single X chromosome (XEif2s3yOSry), represent a minimum Y chromosome contribution compatible with spermatogenesis progressing to haploid round spermatids (Yamauchi et al., 2014). The presence of either Sxra, or Sxrb or the Sry transgene, warrants development of testis. The presence of Eif2s3y, either encoded within Sxra, or added as a transgene to XOSry or XSxrbO mice, allows for the initiation and progression of spermatogenesis. These three models differ regarding the presence of Zfy: mice with just Sry and Ei2s3y transgenes have no Zfy, mice carrying Sxra have both Zfy1 and Zfy2, and mice carrying Sxrb have the Zfy2/1 fusion gene. The Zfy2/1 fusion gene is driven by a strong Zfy2-specific promoter and is expected to encode a protein sequence almost identical to the protein encoded by endogenous Zfy1 (Vernet et al., 2011; Decarpentrie et al., 2012) (Fig. 1 and Table 1).
Genotype . | Y chromosome contribution* (Zfy contribution) . | Meiotic pairing partner for X . | Phenotype . | Offspring obtained . | References# . |
---|---|---|---|---|---|
XY | Intact (Zfy1 and Zfy2) | Y |
| Normal mating | N/A |
XOSry | Sry tg | None |
| None | Mazeyrat et al. (2001) |
XSxrbO | Sxrb (Zfy2/1 fusion gene) | None |
| None | Vernet et al. (2011, 2012) |
XSxraO | Sxra (Zfy1 and Zfy2) | None |
| N/A | Vernet et al. (2011, 2012) |
XSxraY *X | Sxra (Zfy1 and Zfy2) | None |
| ROSI | Vernet et al. (2011, 2012) |
XY *XSxra | Sxra (Zfy1 and Zfy2) | None |
| ROSI and ICSI | Yamauchi et al. (2009) |
XESxrbO | Sxrb (Zfy2/1 fusion gene) | None |
| ROSI | Vernet et al. (2011, 2016b) and Yamauchi et al. (2014, 2015) |
XESxrbY*X | Sxrb (Zfy2/1 fusion gene) | Y*X |
| ROSI | Vernet et al. (2014, 2016b) and Yamauchi et al. (2014, 2015) |
XEOSry | Sry tg, Eif2s3y tg | None |
| ROSI | Vernet et al. (2011, 2012, 2016b), Yamauchi et al. (2014, 2015), and Ruthig et al. (2017) |
XEY*XSry | Sry tg, Eif2s3y tg | Y*X |
| ROSI | Vernet et al. (2014, 2016b) and Yamauchi et al. (2014, 2015) |
XE,Z2OSry | Sry tg, Eif2s3y tg, Zfy2 tg | None |
| ROSI | Vernet et al. (2011) and this study |
XE,Z1/UOSry | Sry tg, Eif2s3y tg, Zfy1/Uba1y tg | None |
| None | Vernet et al. (2011) and this study |
XE,Z1/U,Z2OSry | Sry tg, Eif2s3y tg, Zfy1/Uba1y tg, Zfy2 tg | None |
| ROSI (poor efficiency) | This study |
XE,Z2Y*XSry | Sry tg, Eif2s3y tg, Zfy2 tg | Y*X |
| ROSI and ICSI | Vernet et al. (2014, 2016b) and Yamauchi et al. (2015) |
XE,Z1/UY*XSry | Sry tg, Eif2s3y tg, Zfy1/Uba1y tg | Y*X |
| Not tested | Vernet et al. (2014, 2016b) |
XE,Z1/U,Z2 Y*XSry | Sry tg, Eif2s3y tg, Zfy1/Uba1y tg, Zfy2 tg | Y*X |
| Not tested | Vernet et al. (2014) |
XE,Z2SxrbY*X | Sxrb, Zfy2 tg | Y*X |
| ROSI | Yamauchi et al. (2015) |
XY Zfy1 KO | Intact (Zfy2) but lacking Zfy1 | X |
| Normal mating | Yamauchi et al. (2022) and Nakasuji et al. (2017) |
XY Zfy2 KO | Intact (Zfy1) but lacking Zfy2 | X |
| Normal mating | Yamauchi et al. (2022) and Nakasuji et al. (2017) |
XY Zfy DKO | Intact but lacking Zfy1 and Zfy2 | X |
| ROSI and ICSI | Yamauchi et al. (2022) and Nakasuji et al. (2017) |
Genotype . | Y chromosome contribution* (Zfy contribution) . | Meiotic pairing partner for X . | Phenotype . | Offspring obtained . | References# . |
---|---|---|---|---|---|
XY | Intact (Zfy1 and Zfy2) | Y |
| Normal mating | N/A |
XOSry | Sry tg | None |
| None | Mazeyrat et al. (2001) |
XSxrbO | Sxrb (Zfy2/1 fusion gene) | None |
| None | Vernet et al. (2011, 2012) |
XSxraO | Sxra (Zfy1 and Zfy2) | None |
| N/A | Vernet et al. (2011, 2012) |
XSxraY *X | Sxra (Zfy1 and Zfy2) | None |
| ROSI | Vernet et al. (2011, 2012) |
XY *XSxra | Sxra (Zfy1 and Zfy2) | None |
| ROSI and ICSI | Yamauchi et al. (2009) |
XESxrbO | Sxrb (Zfy2/1 fusion gene) | None |
| ROSI | Vernet et al. (2011, 2016b) and Yamauchi et al. (2014, 2015) |
XESxrbY*X | Sxrb (Zfy2/1 fusion gene) | Y*X |
| ROSI | Vernet et al. (2014, 2016b) and Yamauchi et al. (2014, 2015) |
XEOSry | Sry tg, Eif2s3y tg | None |
| ROSI | Vernet et al. (2011, 2012, 2016b), Yamauchi et al. (2014, 2015), and Ruthig et al. (2017) |
XEY*XSry | Sry tg, Eif2s3y tg | Y*X |
| ROSI | Vernet et al. (2014, 2016b) and Yamauchi et al. (2014, 2015) |
XE,Z2OSry | Sry tg, Eif2s3y tg, Zfy2 tg | None |
| ROSI | Vernet et al. (2011) and this study |
XE,Z1/UOSry | Sry tg, Eif2s3y tg, Zfy1/Uba1y tg | None |
| None | Vernet et al. (2011) and this study |
XE,Z1/U,Z2OSry | Sry tg, Eif2s3y tg, Zfy1/Uba1y tg, Zfy2 tg | None |
| ROSI (poor efficiency) | This study |
XE,Z2Y*XSry | Sry tg, Eif2s3y tg, Zfy2 tg | Y*X |
| ROSI and ICSI | Vernet et al. (2014, 2016b) and Yamauchi et al. (2015) |
XE,Z1/UY*XSry | Sry tg, Eif2s3y tg, Zfy1/Uba1y tg | Y*X |
| Not tested | Vernet et al. (2014, 2016b) |
XE,Z1/U,Z2 Y*XSry | Sry tg, Eif2s3y tg, Zfy1/Uba1y tg, Zfy2 tg | Y*X |
| Not tested | Vernet et al. (2014) |
XE,Z2SxrbY*X | Sxrb, Zfy2 tg | Y*X |
| ROSI | Yamauchi et al. (2015) |
XY Zfy1 KO | Intact (Zfy2) but lacking Zfy1 | X |
| Normal mating | Yamauchi et al. (2022) and Nakasuji et al. (2017) |
XY Zfy2 KO | Intact (Zfy1) but lacking Zfy2 | X |
| Normal mating | Yamauchi et al. (2022) and Nakasuji et al. (2017) |
XY Zfy DKO | Intact but lacking Zfy1 and Zfy2 | X |
| ROSI and ICSI | Yamauchi et al. (2022) and Nakasuji et al. (2017) |
See Fig. 1 for gene content of Y, Sxra, and Sxrb. See text for more details regarding specific genotypes. Abbreviations used: tg, transgene; E, Eif2s3y transgene; Z2, Zfy2 transgene; Z1/U, Zfy1/Uba1y transgene; KO, knock-out; DKO, double knock-out; ROSI: round spermatid injection; ICSI: intracytoplasmic sperm injection. Only X chromosome-encoded Zfy transgenes are included (autosomally encoded Zfy transgenes lead to pachytene arrest preventing spermatogenesis progression).
Only selected, most relevant references describing phenotypic characterization of these mice are shown.
Genotype . | Y chromosome contribution* (Zfy contribution) . | Meiotic pairing partner for X . | Phenotype . | Offspring obtained . | References# . |
---|---|---|---|---|---|
XY | Intact (Zfy1 and Zfy2) | Y |
| Normal mating | N/A |
XOSry | Sry tg | None |
| None | Mazeyrat et al. (2001) |
XSxrbO | Sxrb (Zfy2/1 fusion gene) | None |
| None | Vernet et al. (2011, 2012) |
XSxraO | Sxra (Zfy1 and Zfy2) | None |
| N/A | Vernet et al. (2011, 2012) |
XSxraY *X | Sxra (Zfy1 and Zfy2) | None |
| ROSI | Vernet et al. (2011, 2012) |
XY *XSxra | Sxra (Zfy1 and Zfy2) | None |
| ROSI and ICSI | Yamauchi et al. (2009) |
XESxrbO | Sxrb (Zfy2/1 fusion gene) | None |
| ROSI | Vernet et al. (2011, 2016b) and Yamauchi et al. (2014, 2015) |
XESxrbY*X | Sxrb (Zfy2/1 fusion gene) | Y*X |
| ROSI | Vernet et al. (2014, 2016b) and Yamauchi et al. (2014, 2015) |
XEOSry | Sry tg, Eif2s3y tg | None |
| ROSI | Vernet et al. (2011, 2012, 2016b), Yamauchi et al. (2014, 2015), and Ruthig et al. (2017) |
XEY*XSry | Sry tg, Eif2s3y tg | Y*X |
| ROSI | Vernet et al. (2014, 2016b) and Yamauchi et al. (2014, 2015) |
XE,Z2OSry | Sry tg, Eif2s3y tg, Zfy2 tg | None |
| ROSI | Vernet et al. (2011) and this study |
XE,Z1/UOSry | Sry tg, Eif2s3y tg, Zfy1/Uba1y tg | None |
| None | Vernet et al. (2011) and this study |
XE,Z1/U,Z2OSry | Sry tg, Eif2s3y tg, Zfy1/Uba1y tg, Zfy2 tg | None |
| ROSI (poor efficiency) | This study |
XE,Z2Y*XSry | Sry tg, Eif2s3y tg, Zfy2 tg | Y*X |
| ROSI and ICSI | Vernet et al. (2014, 2016b) and Yamauchi et al. (2015) |
XE,Z1/UY*XSry | Sry tg, Eif2s3y tg, Zfy1/Uba1y tg | Y*X |
| Not tested | Vernet et al. (2014, 2016b) |
XE,Z1/U,Z2 Y*XSry | Sry tg, Eif2s3y tg, Zfy1/Uba1y tg, Zfy2 tg | Y*X |
| Not tested | Vernet et al. (2014) |
XE,Z2SxrbY*X | Sxrb, Zfy2 tg | Y*X |
| ROSI | Yamauchi et al. (2015) |
XY Zfy1 KO | Intact (Zfy2) but lacking Zfy1 | X |
| Normal mating | Yamauchi et al. (2022) and Nakasuji et al. (2017) |
XY Zfy2 KO | Intact (Zfy1) but lacking Zfy2 | X |
| Normal mating | Yamauchi et al. (2022) and Nakasuji et al. (2017) |
XY Zfy DKO | Intact but lacking Zfy1 and Zfy2 | X |
| ROSI and ICSI | Yamauchi et al. (2022) and Nakasuji et al. (2017) |
Genotype . | Y chromosome contribution* (Zfy contribution) . | Meiotic pairing partner for X . | Phenotype . | Offspring obtained . | References# . |
---|---|---|---|---|---|
XY | Intact (Zfy1 and Zfy2) | Y |
| Normal mating | N/A |
XOSry | Sry tg | None |
| None | Mazeyrat et al. (2001) |
XSxrbO | Sxrb (Zfy2/1 fusion gene) | None |
| None | Vernet et al. (2011, 2012) |
XSxraO | Sxra (Zfy1 and Zfy2) | None |
| N/A | Vernet et al. (2011, 2012) |
XSxraY *X | Sxra (Zfy1 and Zfy2) | None |
| ROSI | Vernet et al. (2011, 2012) |
XY *XSxra | Sxra (Zfy1 and Zfy2) | None |
| ROSI and ICSI | Yamauchi et al. (2009) |
XESxrbO | Sxrb (Zfy2/1 fusion gene) | None |
| ROSI | Vernet et al. (2011, 2016b) and Yamauchi et al. (2014, 2015) |
XESxrbY*X | Sxrb (Zfy2/1 fusion gene) | Y*X |
| ROSI | Vernet et al. (2014, 2016b) and Yamauchi et al. (2014, 2015) |
XEOSry | Sry tg, Eif2s3y tg | None |
| ROSI | Vernet et al. (2011, 2012, 2016b), Yamauchi et al. (2014, 2015), and Ruthig et al. (2017) |
XEY*XSry | Sry tg, Eif2s3y tg | Y*X |
| ROSI | Vernet et al. (2014, 2016b) and Yamauchi et al. (2014, 2015) |
XE,Z2OSry | Sry tg, Eif2s3y tg, Zfy2 tg | None |
| ROSI | Vernet et al. (2011) and this study |
XE,Z1/UOSry | Sry tg, Eif2s3y tg, Zfy1/Uba1y tg | None |
| None | Vernet et al. (2011) and this study |
XE,Z1/U,Z2OSry | Sry tg, Eif2s3y tg, Zfy1/Uba1y tg, Zfy2 tg | None |
| ROSI (poor efficiency) | This study |
XE,Z2Y*XSry | Sry tg, Eif2s3y tg, Zfy2 tg | Y*X |
| ROSI and ICSI | Vernet et al. (2014, 2016b) and Yamauchi et al. (2015) |
XE,Z1/UY*XSry | Sry tg, Eif2s3y tg, Zfy1/Uba1y tg | Y*X |
| Not tested | Vernet et al. (2014, 2016b) |
XE,Z1/U,Z2 Y*XSry | Sry tg, Eif2s3y tg, Zfy1/Uba1y tg, Zfy2 tg | Y*X |
| Not tested | Vernet et al. (2014) |
XE,Z2SxrbY*X | Sxrb, Zfy2 tg | Y*X |
| ROSI | Yamauchi et al. (2015) |
XY Zfy1 KO | Intact (Zfy2) but lacking Zfy1 | X |
| Normal mating | Yamauchi et al. (2022) and Nakasuji et al. (2017) |
XY Zfy2 KO | Intact (Zfy1) but lacking Zfy2 | X |
| Normal mating | Yamauchi et al. (2022) and Nakasuji et al. (2017) |
XY Zfy DKO | Intact but lacking Zfy1 and Zfy2 | X |
| ROSI and ICSI | Yamauchi et al. (2022) and Nakasuji et al. (2017) |
See Fig. 1 for gene content of Y, Sxra, and Sxrb. See text for more details regarding specific genotypes. Abbreviations used: tg, transgene; E, Eif2s3y transgene; Z2, Zfy2 transgene; Z1/U, Zfy1/Uba1y transgene; KO, knock-out; DKO, double knock-out; ROSI: round spermatid injection; ICSI: intracytoplasmic sperm injection. Only X chromosome-encoded Zfy transgenes are included (autosomally encoded Zfy transgenes lead to pachytene arrest preventing spermatogenesis progression).
Only selected, most relevant references describing phenotypic characterization of these mice are shown.
In normal males, X–Y synapsis is mediated by the homologous pairing regions (PARs) of the X and Y chromosomes. Sex reversed males lack the Y chromosome and instead have Sxra (or Sxrb) attached distal to the PAR of one of its X chromosomes (XXSxra or XXSxrb) (Fig. 1A) or carry an autosomally encoded Sry (XXSry). The presence of two X chromosomes in a male mouse results in sterility, as germ cells are prevented from progressing beyond prospermatogonia (Cattanach et al., 1971; Burgoyne et al., 1986; Sutcliffe and Burgoyne, 1989). Thus, males with a single X chromosome are preferred for investigation of spermatogenesis in the Sxr carriers (XSxraO or XSxrbO) and Sry transgenics (XOSry), because in these males, spermatogenesis can be initiated with progress through meiosis (Fig. 1 and Table 1). However, X chromosome univalency is not benign for spermatogenesis progression, as it triggers meiotic arrest and apoptosis. The negative effects of X chromosome univalency can be prevented by providing a meiotic pairing partner satisfying PAR synapsis: Y*X chromosome variant (Fig. 1A) (Odorisio et al., 1998). Y*X is an X chromosome with a deletion that removes essentially all coding sequences that are expected to interfere with spermatogenesis (like in XX) while retaining PAR that allows for effective pairing with an X (Burgoyne et al., 1992). In our hands, a successful pairing of X and Y*X was observed in 85% of pachytene spermatocytes in males with Sry and Eif2s3y transgenes in the XY*X context (Yamauchi et al., 2014). Finally, several Zfy1 and Zfy2 transgenic mouse lines were developed by the Burgoyne Lab, some with the Zfy transgenes expressed from autosomes (to the detriment of spermatogenesis), and some with the transgenes expressed from the X chromosome (compatible with spermatogenesis) (Royo et al., 2010).
In sum, all pregenome editing studies of Zfy gene function were performed with mice carrying sex chromosome variants (Sxra and Sxrb) and/or minimal Y-derived transgenes (Sry, Eif2s3y), in the context of either XO or XY*X sex chromosomes, and with and without Zfy transgenes (Fig. 1 and Table 1).
Genomic structure and expression of ZFY/Zfy
The initial findings regarding ZFY/Zfy expression originate from the time when it was considered a candidate for TDF/TDY. Human ZFY was found to have two transcript variants: a 3 kb variant specific to the testis and a longer 5.7 kb variant present in somatic tissues (Lau and Chan, 1989). Homologous 3 kb ZFY transcript variants were also discovered in the adult testis of several other mammal species, suggesting that ZFY may have a conserved function in spermatogenesis. A similar expression pattern was observed for ZFX, with a shorter 5 kb variant specific to the testis and longer 6.7 and 8 kb variants present in somatic tissues (Lau and Chan, 1989). In mice, Zfy1 and Zfy2, as well as the autosomal homologue Zfa, were found to be expressed primarily in the adult testis (Mardon et al., 1989; Ashworth et al., 1990). Additionally, Zfy transcripts were detected in embryonic stem cells, blastocysts and fetal gonadal cells (Zfy1 only), as well as fetal mouse tissues (Zfy1 and Zfy2). The X-linked homologue Zfx, on the other hand, was discovered to be ubiquitously expressed (Lau and Chan, 1989).
The expression of ZFY/Zfy was revisited in 2012 (Decarpentrie et al., 2012). Using both wild-type mice and models with varying Zfy contributions (Sxra and Sxrb carriers), Zfy transcription was characterized in depth, revealing several important features. First, Zfy1 and Zfy2 were shown to have unique expression patterns. Transcripts of both homologues were found to be expressed in spermatocytes during the diplotene and zygotene stage of meiotic prophase I, then silenced during the pachytene phase with the onset of Meiotic Sex Chromosome Inactivation (MSCI), and expressed again postmeiotically, in spermatids (Fig. 2). Second, Zfy2 expression was found to predominate over Zfy1 expression, especially in spermatids (Fig. 2). Before MSCI, both Zfy1 and Zfy2 expression was shown to be driven by their homologous Zfy promoters. However, postmeiotically, Zfy2 expression is driven by a stronger spermatid-specific promoter (Fig. 3A). Third, Zfy was shown to undergo alternative splicing to create transcript variants. Two Zfy1 transcript variants were observed: a short one which has exon 4 removed by alternative splicing, and a long one, which retains exon 4 (Fig. 3A). The short Zfy1 transcript was shown to predominate in spermatocytes, whereas the long Zfy1 transcript predominates in spermatids. The short Zfy2 transcript variant was present only at low levels compared to the long Zfy2. Fourth, the presence of the testis-specific ZFY transcript was confirmed in both human spermatocytes and round spermatids. This transcript variant was reported to have exon 3 (homologous to exon 4 in mice) removed via alternative splicing (Fig. 3A) and is therefore the equivalent of the mouse short Zfy transcripts. The short Zfy transcript variant was also identified in sheep (Zhang et al., 2018), providing evidence that Zfy splicing may be more widely conserved.
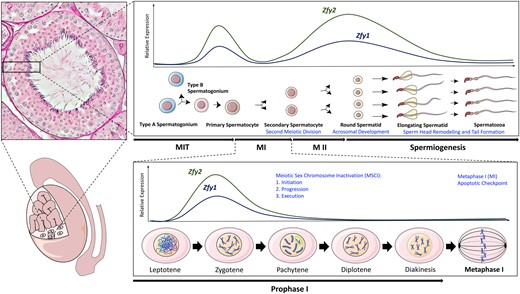
The role of mouse Zfy in spermatogenesis. Schematic diagram illustrating/showing the roles ascribed to Zfy during spermatogenesis, based on previous transgene-rescue studies. Illustrations of mouse testis, male germ cell development, and meiosis I are shown, along with an animated image of a seminiferous tubule cross-section. The roles of Zfy are shown in blue text font, and Zfy expression in relation to mitosis, meiosis, and spermiogenesis is represented by lines (Zfy1 shown with dark blue, Zfy2 shown with dark green). Relative expression levels shown are representative of published RNA-seq data (Gan et al., 2013; Green et al., 2018). The paternal and maternal chromosomes are shown in purple and blue, respectively, during prophase I and metaphase I. The Meiotic Sex Chromosome Inactivation (MSCI) sex body in pachytene spermatocytes is shown by a green oval. MSCI initiation, progression, and execution, refers to the triple role of Zfy in MSCI: first initiating MSCI in pachytene spermatocytes, then monitoring MSCI progression throughout meiosis I, and then finally executing cells that display MSCI failure, as previously described (Royo et al., 2010; Vernet et al., 2016a). MIT, mitosis; MI, meiosis I; MII, meiosis II.
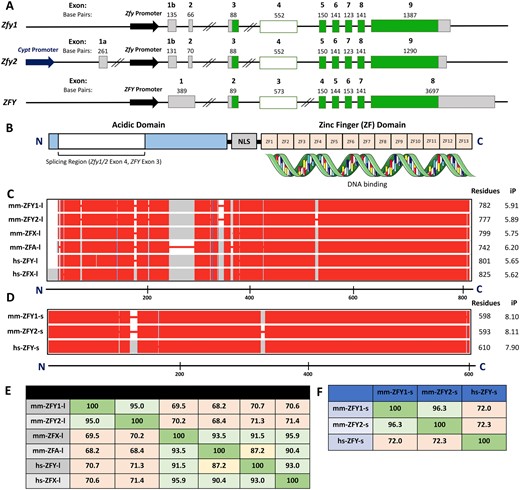
ZFY/Zfy genomic sequence and protein structure. (A) Genomic sequence of coding and noncoding exons for mouse Zfy1 (transcript ID ENSMUST00000065545.6) and Zfy2 (transcript ID ENSMUST00000115891.2 with exon 1b from ENSMUST00000187148.7) and human ZFY (transcript ID ENST00000155093.8). Coding exons are shown in dark green, noncoding exons are shown in gray. Zfy1 and Zfy2 exon 4 and ZFY exon 3, which are differentially spliced, are shown in shaded green. Sequences are not drawn to scale. Double line breaks represent large gaps (>10 kb) in genomic sequence. (B) Protein structure of mouse and human ZFY. The acidic domain is shown in light blue and the 13 zinc finger domains (ZF1–13) are shown in orange. The region of ZFY encoded by human ZFY exon 3 and mouse Zfy1 and Zfy2 exon 4 is shown in shaded blue. The nuclear localization sequence (NLS) is shown in gray. N and C terminal ends are designated by N and C, respectively. (C) Protein sequence alignments for the long variants of mouse ZFY1, ZFY2, ZFA, and ZFX, as well as human ZFY and ZFX. (D) Protein sequence alignments for the short variants of mouse ZFY1 and ZFY2 and human testis-specific ZFY. The mouse ZFY protein sequences are those predicted based on the exon 4 splicing patterns previously observed (Decarpentrie et al., 2012). In (C) and (D), the conserved sequences are shown in red, while sequences not conserved across all isoforms are shown in gray. The absence of protein sequence is illustrated by red lines. The scale bar shown below represents protein length in amino acid residues. N- and C-terminal ends are designated by N and C, respectively. Protein length in amino acid residues, as well as isoelectric point (iP) are shown to the right of the alignments. (E) Table illustrating protein sequence percent similarity between long variants for human and mouse isoforms. (F) Table illustrating protein sequence percent similarity between short variants for human and mouse isoforms.
The human (hs) and mouse (mm) ZFY protein sequences encode an N-terminal acidic domain (shown to be the transactivating domain in vitro) and a C-terminal zinc-finger domain with the capacity to bind to DNA (Fig. 3B) (Mardon et al., 1990; Vernet et al., 2014). The zinc finger array is responsible for DNA binding while the activation domain is expected to be largely unstructured and to attract other proteins, such as the Mediator complex (Frietze and Farnham, 2011; Sanborn et al., 2021). The short variants (mm-ZFY1-s, mm-ZFY2-s, and hs-ZFY-s) contain a truncated N-terminal acidic domain while the long variants (mm-ZFY1-l, mm-ZFY2-l, and hs-ZFY-l) encode the complete acidic domain (Fig. 3B).
The C-terminal zinc finger domain is strongly conserved across all ZFY variants and their homologues, whereas there is some protein sequence variation in the N-terminal acidic domain (Fig. 3C and D). The mm-ZFY1-l and mm-ZFY2-l protein sequences are 95% similar and share at least 70% homology with hs-ZFY-l, hs-ZFX-l, mm-ZFX-l, and the predicted mm-ZFA-l protein sequence (Fig. 3E) (note that NCBI currently lists Zfa as a pseudogene; gene id 22639, updated 26 September 2022). The mm-ZFY1-s and mm-ZFY2-s sequences also share ∼95% protein similarity to each other and ∼70% similarity to hs-ZFY-s (Fig. 3F).
The acidic domain of ZFY1, ZFY2, and ZFX has been shown to activate transcription in yeast when fused to the DNA-binding domain of the Gal4 transcriptional activator (Mardon et al., 1990; Vernet et al., 2014). The same assay was used to investigate the functional differences between the short and long Zfy and ZFY isoforms (Decarpentrie et al., 2012). The long acidic domains encoded by mm-ZFY1-l, mm-ZFY2-l, and hs-ZFY-l activated β-galactosidase production, but the short acidic domains of mm-ZFY1-s, mm-ZFY2-s, and hs-ZFY-s did not. Thus, these isoforms have the capacity to bind but not transactivate, and may therefore serve as competitive inhibitors for full length ZFYs.
Role of Zfy in meiosis I: MSCI
One of the first studies that revisited the function of mouse Zfy regarded MSCI (Royo et al., 2010). The mammalian X and Y chromosomes share little homology and are therefore mostly nonsynapsed. The asynapsis triggers meiotic sex chromosome inactivation, termed MSCI (Turner, 2007). To test whether MSCI is essential for normal male meiotic progression, two unique mouse models with one X and two Y chromosomes were used. These two models are genetically identical and have the same sex chromosome set but in one of them, the two Y chromosomes are synapsed (XYY), while in the other (XYY), the two Ys remain distinct (nonsynapsed), because one of them is attached distal to X PAR and therefore one of the two PARs is not at the chromosome extremity prompting pairing. Two synapsed Ys in XYY escape MSCI and express the genes they encode during pachytene, resulting in pachytene stage spermatogenesis arrest. Two nonsynapsed Ys in XYY retain MSCI and spermatogenesis progresses normally in these mice. When the Y chromosome short arm derived transgenes are expressed from autosomes, they also escape MSCI. Seven of these autosomally encoded transgenes (Uba1y, Kdm5d, Eif2s3y, Uty, Ddx3y, Usp9y, and Rbmy) were shown to be neutral when expressed during the pachytene, and two (Zfy1 and Zfy2) resulted in the pachytene stage arrest phenotype mirroring that observed with the XYY model. While expression of Zfy1 and Zfy2 transgenes from their autosomal location led to escape from MSCI, infertility, and pachytene arrest, when the Zfy transgenes were purposefully placed on the X chromosome, MSCI took place and spermatogenesis progressed normally. Thus, mis-expression of Zfy genes during pachytene was proved to cause the pachytene stage arrest (Royo et al., 2010).
Several years later, another report pointed to the role of Zfy in MSCI (Vernet et al., 2016a). Microarray analysis was performed on whole testis from males with a single X chromosome, an autosomally encoded Sry transgene, and an X chromosome encoded Eif2s3y transgene, either without (XEOSry) or with an X chromosome encoded Zfy2 transgene (XE,Z2OSry) (Fig. 1B and Table 1). The testes were collected from males at 17.5 days postpartum (dpp), at which point, the most advanced germ cells present are late pachytene/early diplotene spermatocytes. A broad upregulation of X chromosome transcripts and a downregulation of pachytene spermatocyte-specific, autosomally encoded transcripts were observed in XEOSry when compared to XE,Z2OSry males and when compared to existing data obtained with testes from 17 dpp wild-type XY males (Vernet et al., 2016a). The upregulation of X chromosome genes in testes from XEOSry males indicated a possibility of MSCI failure. The MSCI leakage/failure was further confirmed by showing that the upregulation of X genes is not due to a shift in spermatogonia/spermatocyte ratio and by demonstrating with RNA–FISH that an X-linked Scml2 gene, known to be silenced during MSCI, remained expressed in pachytene spermatocytes. An increase in spermatocyte apoptosis, known to result from MSCI leakage, was also observed in testis sections from 15 dpp XEOSry males.
The MSCI failure was also detected in older XEOSry males, at 30–31 dpp, and in young adult males, but some postmeiotic cells were observed. Together, these findings suggested that (i) MSCI failure was not limited to the first wave of spermatogenesis and (ii) some spermatocytes progressed through meiosis I (MI) despite unsuccessful MSCI. Surprisingly, no increase in apoptotic cells was noted in older XEOSry males compared to XE,Z2OSry, despite the confirmed MSCI failure for this age. RNA–FISH analysis was used to show that the presence of either Zfy2 (in XE,Z2OSry males) or Zfy1 (in XE,Z1/UOSry males) restored MSCI in older males, with no difference in MSCI efficiency observed between the two genotypes.
Together, these MSCI-centered studies (Royo et al., 2010; Vernet et al., 2016a) demonstrated that Zfy genes play a triple role at the MSCI checkpoint (Fig. 2). First, they are required for efficient MSCI initiation. Second, thanks to their Y chromosome location, they serve as the sensors of MSCI progress: i.e. success or failure. Third, they act as the ‘executioners’, causing pachytene stage spermatogenesis arrest when they are misexpressed due to MSCI failure. Interestingly, both Zfy1 and Zfy2 homologues were found to be similarly involved in MSCI regulation, even though other studies have shown that Zfy2 is the more potent homologue (Vernet et al., 2011, 2014; Decarpentrie et al., 2012).
Role of Zfy in Y chromosome evolution
The studies reporting on Zfy function in MSCI and as being an MSCI executioner (Royo et al., 2010) led to an interesting theory regarding Zfy in Y chromosome evolution (Waters and Ruiz-Herrera, 2020; Ruiz-Herrera and Waters, 2022).
The therian X and Y chromosomes are believed to have arisen from an autosomal pair over 165 million years ago, shortly after the divergence of monotremes from the eutherian and marsupial lineages (Warren et al., 2008; Waters and Ruiz-Herrera, 2020). Compared to the X chromosome, the Y contains only a reduced number of protein-coding genes. The Y chromosome is speculated to be rapidly losing genes, with the potential to vanish within 10 million years at the current rate of gene loss (‘Wimpy Y Hypothesis’; Marshall Graves, 2000; Aitken and Marshall Graves, 2002). The Y chromosome has also been described as ‘fragile’ (‘Fragile Y Hypothesis’; Blackmon and Demuth, 2014; Waters and Ruiz-Herrera, 2020), explaining that due to shrinking PARs and a resulting increased risk of nondisjunction with the X, a strong selective pressure exists to transfer functions from the Y to autosomal chromosomes. This compensatory mechanism is wide-spread among mammals (Hughes et al., 2015).
Paradoxically, the Y chromosome is conserved across nearly all eutherians and marsupials, with only a few reported instances of Y-less mammals (Arakawa et al., 2002; Fredga, 1988; Matveevsky et al., 2017; Mulugeta et al., 2016). Meiosis is believed to be a key element to Y chromosome evolution, yet how the meiotic cellular program can influence Y chromosome survival is still unknown. According to a recently conceived ‘persistent Y’ hypothesis (Waters and Ruiz-Herrera, 2020; Ruiz-Herrera and Waters, 2022), a strong evolutionary pressure for the Y chromosome to persist depends on the existence of meiotic executioner/s that must be: (i) encoded on a Y chromosome, (ii) essential for survival or fertility, and (iii) pachytene lethal when ectopically expressed during MSCI. As pachytene expression of executioner genes is lethal, the only scenario in which executioner gene function could be transferred from the Y is via transposition to the X, which is also subject to MSCI. Because the X chromosome comprises only a small fraction of the eutherian genome, transpositions from the X to the Y are relatively rare evolutionary events.
Once considered a contender for the testis-determining factor, Zfy is now believed to be a top candidate for an executioner gene in eutherians, with the strongest evidence coming from mice (Royo et al., 2010; Vernet et al., 2016a), but also horses (Ruiz et al., 2019; Bugno-Poniewierska and Raudsepp, 2021), pigs (Barasc et al., 2012), and rodents that had the lost Y chromosome with Zfy translocated to the X chromosome (Ellobius and Tokudaia) (Arakawa et al., 2002; Mulugeta et al., 2016). The ZFY sequence was annotated on the recently sequenced giant panda Y chromosome and a gene conversion was observed for the ZFY exon 7 (Han et al., 2018). Phylogenetic analysis revealed that this gene conversion happened independently in multiple mammalian lineages, indicating a putative mechanism to maintain the function of this particular gene on the mammalian Y chromosome (Han et al., 2018), providing additional evidence supportive of important roles played by ZFY. Thus, through its critical regulatory function during MSCI, Zfy could play a crucial role in conservation of the Y chromosome throughout eutherians.
Role of Zfy in meiosis I: first meiotic metaphase
In addition to regulating MSCI in pachytene spermatocytes, Zfy was also discovered to have functions during the first meiotic metaphase of MI. During mammalian male meiosis, there is an efficient apoptotic elimination of cells with unpaired (univalent) chromosomes at M1 (Burgoyne et al., 2009). This phenomenon has been studied using mouse models lacking the Y chromosome and with a univalent X chromosome.
Earlier studies documented that in XSxraO males, there is an efficient apoptotic elimination of MI spermatocytes (Cattanach et al., 1971; Kot and Handel, 1990; Sutcliffe et al., 1991; Burgoyne et al., 1992), while in XESxrbO, this apoptotic response is not immediately evident (Mazeyrat et al., 2001). Three models, XSxraO, XESxrbO, and XEOSry (Fig. 1 and Table 1), were therefore directly compared in regard to spermatogenesis progression and germ cell apoptosis (Vernet et al., 2011). In wild-type XY males, the two meiotic divisions are completed during the stage of XII of the spermatogenic epithelium (Russell, 1990). In XSxraO, most of the MI spermatocytes at 30 dpp are arrested and apoptotic, and meiosis is never completed. In XESxrbO and XEOSry males, most spermatocytes complete the first meiotic division but instead of progressing through the second division, they arrest, forming interphasic secondary spermatocytes. These interphasic secondary spermatocytes accumulate in the subsequent tubule stages, and cells of the typical round spermatids size and nuclear morphology were not observed. The histological assessment was verified by immunohistochemistry on spread spermatogenic cells: haploid cells lacking SYCP3 staining were not observed (Vernet et al., 2011). However, we have subsequently shown that haploid spermatids are produced by XESxrbO and XEOSry males, but are rare and constitute <15% of cells with spermatid-like morphology; these haploid spermatids were functional in assisted fertilization and live offspring from XESxrbO and XEOSry males could be obtained after round spermatid injection (ROSI) (Yamauchi et al., 2014).
The completion of the first meiotic division by spermatocytes in XESxrbO and XEOSry could not have happened if these cells had been eliminated by apoptosis at MI, as in XSxraO. When apoptosis was quantified with TUNEL assay on 30 dpp testis sections, a marked decrease in percentage of tubules with apoptotic MI spermatocytes was observed with XESxrbO and XEOSry males, when compared to XSxraO. Thus, the apoptotic response to X chromosome univalence was delayed or reduced in these males. This suggested that a Y chromosome gene present in XSxraO and not in XESxrbO and XEOSry, is involved in regulation of elimination of MI cells with a univalent X chromosome. To identify this gene, the BAC transgenes derived from ΔSxrb (Mazeyrat et al., 2001; Royo et al., 2010) were individually added to XEOSry. The autosomally encoded Uba1y, Ddx3y, Kdm5d, Uty, and Usp9y transgenes were not able to rescue the apoptotic response and prevent the meiotic progression to secondary spermatocytes. The autosomally encoded Zfy1 and Zfy2 transgenes caused the pachytene stage arrest and infertility due to a complete elimination of midpachytene spermatocytes (Royo et al., 2010), thus their effect on subsequent stages of meiosis could not be assessed. When the Zfy1 and the Zfy2 transgenes expressed from X chromosome were considered in the context of XEOSry, Zfy2, but not Zfy1, was able to restore the apoptotic response, with the number of secondary spermatocytes decreasing in parallel to the increase of apoptotic MI spermatocytes (Vernet et al., 2011).
Thus, Zfy2 was identified as the sole NPYp gene that acts to remove cells with unpaired chromosomes at the first meiotic division (Fig. 2).
Role of Zfy in meiosis II
In 2014, in a collaborative work with Burgoyne’s group, we investigated the role of the Zfy genes in meiosis II (MII) (Vernet et al., 2014). The XO models with varying Zfy contribution (XEOSry, XESxrbO, XSxraO; Fig. 1) are not optimal to study MII because of the apoptotic response to the univalent X chromosome during MI (Burgoyne et al., 2009). Thus, we investigated the XEY*XSry, XESxrbY*X, and XSxraY*X males which, in addition to a single X chromosome, also carried the sex chromosome variant Y*X (Fig. 1C). The presence of Y*X allowed the sex bivalent to form in >70% of pachytene spermatocytes in these models. We first quantified the incidence of true haploid round spermatids, distinguishing them from similarly looking diploid interphasic spermatocytes which entered spermiogenesis without completing meiosis, and related it to our previous data on ploidy frequency of XESxrbO and XEOSry males (Vernet et al., 2012). These analyses revealed that XEOSry and XEY*XSry males had similar incidence of haploid round spermatids. However, the XESxrbY*X produced haploid round spermatids much more efficiently than the XESxrbO males, and spermatid production in XY*XSxra males was comparable to that in XY males. Together, this indicated that MII is not potentiated solely by the PAR–PAR synapsis, but that there is a genetic information encoded within Sxra, that is partially retained in Sxrb, that promotes MII in the context of a sex bivalent. Among protein-coding genes within Sxrb, only the Zfy2/1 fusion gene is expressed both pre- and postmeiotically. The Zfy1 and Zfy2 transgenes were thus individually and in combination added to XEY*XSry males. The addition of the Zfy1 transgene significantly increased the number of haploid spermatids (from 17.4% to 47.2%) while the addition of Zfy2 or both homologues almost completely restored haploid spermatid production (78.2% and 87.6% for Zfy2 and Zfy1 +Zfy2, respectively). Interestingly, overexpression of the X homologue Zfx also increased haploid spermatid production (from 15.0% to 79.9%). Thus, the presence of the sex bivalent, Zfy2 and, to a lesser extent, Zfy1 and Zfx, aids progression through MII (Fig. 2).
Zfy genes are subject to MSCI and are thus expected to be silenced at the beginning of the pachytene stage and reactivated postmeiotically. Using a combination of DNA–FISH and RNA–FISH, we demonstrated that Zfy1, Zfy2, and Zfx are all expressed in secondary spermatocytes. An interphase between meiotic divisions is male specific and our data provided the first direct evidence that it may serve to allow MII critical X and Y gene reactivation following sex chromosome silencing in meiotic prophase. The changes observed during MII, however, may also be a consequence of an earlier cellular insult. An early spermatogenesis defect appearing due to deficiency or lack of Zfy, such as disrupted MSCI, could lead to some aberrations within cells that would persist due to a lack of their clearing by apoptosis, which would then have consequences for MI and MII progression. There is still much to learn about how Zfy impose their important roles during spermatogenesis, and whether the multiple functions of are separable or whether some of the later events are part of the same initial cellular defect.
Role of Zfy in spermiogenesis
The three models with varying NPYp content (XSxraO, XESxrbO, and XEOSry; Fig. 1) were used to demonstrate that NPYp (MSYp) encode genes necessary for spermiogenesis (Vernet et al., 2012, 2016b).
Despite the strong apoptotic response to the univalent X chromosome during metaphase I, a small number of XSxraO spermatocytes progress through meiosis and undergo spermiogenesis either as diploid or haploid spermatids, although sperm development is not complete due to the absence of the Y chromosome long arm (Levy and Burgoyne, 1986; Kot and Handel, 1990; Sutcliffe et al., 1991; Cocquet et al., 2009). The XESxrbO and XEOSry males were also suspected to produce spermatids able to undergo some degree of morphogenesis. This was because prior data have shown that interphasic spermatocytes can enter spermiogenesis (Vernet et al., 2011), expression of the spermatid-specific Zfy2 promoter can be seen in XESxrbO males (Decarpentrie et al., 2012), and spermatid elongation takes place in older XEOSry males (Mazeyrat et al., 2001).
To determine the extent to which NPYp genes, including Zfy1 and Zfy2, contribute to spermiogenesis, spermatid development was assessed in XY, XSxraO, XESxrbO, and XEOSry males at 41–45 dpp, at which time spermiogenesis occurs normally in XY males (Vernet et al., 2012). Both diploid and haploid spermatids were observed in the three XO models, with XSxraO producing significantly more haploid cells compared to XEOSry and XESxrbO (44.1% vs 11.4% and 5.2%, respectively) but fewer than XY males (99.1%). The spermatids from all genotypes underwent some acrosome development, with XO models displaying defects in acrosome structure. Spermatid nuclei elongation and condensation was only observed in XSxraO and XESxrbO, and not in XEOSry males. Also, the spermatids from XESxrbO males displayed delayed head morphogenesis when compared to XSxraO and XY males, and spermatids undergo elongation regardless of their ploidy status. Sperm tail development was frequent in XSxraO, somewhat less common in XESxrbO males, and never observed in XEOSry.
Together, these findings supported the idea that Sxra and, to a lesser extent, Sxrb encode genetic information essential for promoting spermiogenesis.
The NPYp contribution to spermiogenesis was also assessed using males with the same Y chromosome gene content but carrying the meiotic pairing partner Y*X (Fig. 1) and therefore not subjected to the meiotic arrest due to the X chromosome univalency (Yamauchi et al., 2015; Vernet et al., 2016b). The addition of Y*X did not enhance spermatid elongation or sperm morphogenesis. XEY*XSry and XEOSry males displayed similar arrest at the round spermatid stage, with no sperm head or tail development observed, while XESxrbY*X had similar delayed spermatid elongation, nuclear condensation and acrosome relocation as that observed with XESxrbO (Vernet et al., 2016b). When compared to XY, XESxrbY*X males had 2.5 times fewer round spermatids but almost 14 times fewer elongating/elongated spermatids, indicating that not all round spermatids undergo elongation (Yamauchi et al., 2015).
When the Zfy1 and Zfy2 transgenes were separately introduced to the XEY*XSry males to assess their role in sperm morphogenesis, spermatid elongation, and tail development was observed with addition of Zfy2 but not Zfy1 (Vernet et al., 2016b). However, the number of elongating/elongated spermatids remained significantly lower (8.5 times fewer) than in XY males (Yamauchi et al., 2015). Thus, Zfy2, but not Zfy1, partially restores spermiogenesis of XEY*XSry. In agreement with this notion, more elongated and condensed spermatids were observed in XE,Z2Y*XSry than in XESxrbY*X males, which carry the Zfy2/1 fusion gene (Yamauchi et al., 2015). However, the addition of Zfy2 to XEY*XSry males did not reinstate spermiogenesis progression comparable to that seen in XY*XSxra males, which have both Zfy1 and Zfy2. XE,Z2Y*XSry males had more compromised sperm morphogenesis with signs of necrosis, acrosome invagination, incomplete elongation, and formation of cytoplasmic vacuoles. Thus, the presence of Zfy2 is enough to overcome Step 7 spermatid arrest and initiate elongation but it is qualitatively and quantitatively impaired compared to that seen with the complete Zfy contribution or contribution of other NPYp genes. Very few or no sperm were observed in the epididymis of XE,Z2Y*XSry males and, instead, sloughed round spermatids were frequently noted (Yamauchi et al., 2015; Vernet et al., 2016b). The headshape of both epididymal and testicular sperm was always abnormal, and more severely abnormal than in XY*XSxra males (Yamauchi et al., 2015).
Together, the data supported that the presence of Zfy2 prompted round spermatids to initiate and undergo sperm head morphogenesis and complete tail development (Fig. 2). The Zfy2 in XE,Z2Y*XSry males made this transition much more effectively than Zfy2/1 in XESxrbY*X but did not reach the level observed in males with a complete Zfy contribution or contribution of other NPYp genes provided by Sxra.
Role of Zfy in assisted reproduction in mice with limited Y chromosome complements
Reproduction requires two gametes, sperm and oocyte, which are very different in what they provide for the union. During naturally occurring fertilization, sperm must deliver to the oocyte a complete haploid genome, properly packed chromatin with intact DNA, and a proper epigenome (i.e. chromatin organization, DNA methylation, and noncoding RNAs). Sperm also must be present in sufficient numbers, have proper motility and morphology, and be able to undergo several functional transformations taking place in the female reproductive tract. Many of these qualities are also required in conventional IVF. With the advent of a method called ICSI, however, the requirements of what a male gamete must bring became simplified. Instead of allowing sperm to struggle to deliver its DNA and other components to the oocyte through the expanse of female reproductive tract, the sperm head can simply be injected via ICSI to achieve fertilization. This eliminates the need for most of the essential sperm features. What is left is the bare minimum: the sperm haploid genetic material and epigenome. And the latter does not have to be perfectly normal to achieve fertilization, development, and live birth. It is also possible to obtain successful fertilization and live offspring after injection of haploid round spermatids using a similar technique, ROSI.
In 2013, we demonstrated that live offspring can be obtained after ROSI with germ cells from males with only two Y chromosome genes: Sry and Eif2s3y (XEOSry and XEY*XSry; Fig. 1) (Yamauchi et al., 2014). However, the efficiency of ROSI measured as a proportion of offspring from embryos transferred, was much lower for these males than for XY males with a full Y chromosome complement. When ROSI was performed with round spermatids from males carrying the Sxrb (XESxrbO and XESxrbY*X; Fig. 1), the efficiency increased by over 2-fold but remained considerably lower than that of XY males. This indicated that among the genes encoded within Sxrb, there is one or more that facilitates spermatid function in ROSI.
Our next goal was to identify the Sxrb-encoded gene responsible for this function. Sxrb encodes Rbmy, H2al2y (H2al2b and H2al2c), Sry, Zfy2/1, Teyorf1, and Prssly (Fig. 1A). Expression and translation of mouse Sry occur very briefly during fetal development (Gubbay et al., 1990; Koopman et al., 1991) and in adult testes, Sry transcripts are thought to be aberrant and not translatable (Capel et al., 1993; Jeske et al., 1995). The presence of the RBMY protein is questionable beyond zygotene (Burgoyne and Mitchell, 2007) while H2al2y (H2al2b and H2al2c) is expressed late during spermiogenesis (Ferguson et al., 2009). Thus, based on expression pattern and known function Sry, Rbmy, and H2al2y (H2al2b and H2al2c) were not considered as viable candidates. At the time when the experiments were done, Prssly and Teyorf1 had not yet been discovered; the existence of these genes was only shown when the mouse Y chromosome sequence was published (Soh et al., 2014), and we have since shown that both genes are dispensable for fertility in mice (Holmlund et al., 2022). We therefore focused our attention on Zfy1 and Zfy2, which appear in Sxrb as a Zfy2/1 fusion gene. We added the Zfy2 transgene to males carrying Eif2s3y and Sry (XE,Z2OSry and XE,Z2Y*XSry males; Fig. 1 and Table 1) and observed an increase in the ROSI efficiency above that noted in mice with Sxrb (27% and 43% (Yamauchi et al., 2015) vs 20% and 16% (Yamauchi et al., 2014)). Spermatogenesis in XE,Z2Y*XSry males was progressing well with good spermatid elongation up to Steps 15–16. Testicular sperm were severely morphologically abnormal, but this was expected in males lacking NPYq. When testicular sperm were used in ICSI, successful fertilization was achieved and live offspring (23%) were born (Yamauchi et al., 2015). Interestingly, some but not all XESxrbY*X males occasionally produced few testicular sperm. These sperm, however, could not support embryonic and fetal development after ICSI (Yamauchi et al., 2015).
Together, these data demonstrate that Zfy2 is the gene that increases the functional prowess of round spermatids in ROSI and allows them to undergo transformation into functional sperm in ICSI.
Limitations of studies with transgenic and mutant mice
Previous work involving mice with Y chromosome mutations and transgene rescue strategies produced convincing evidence that Zfy1 and Zfy2 play important roles during spermatogenesis. However, all of these studies share the same significant limitation: the function of Zfy was always evaluated in the context of other Y chromosome deficiencies, and often with mice with only few Y chromosome genes left. Some of these deficiencies, for example loss of NPYq, are known to have profound consequences for spermatogenesis (Yamauchi et al., 2009, 2010, 2012; Riel et al., 2019). Thus, it was difficult to decipher whether Zfy genes were solely responsible for the observed mouse phenotypes and how much was contributed by the lack of other Y chromosome genes. To test Zfy function directly, gene specific knock-out (KO) was needed. This has become possible only after genome editing strategies were shown to be applicable for Y chromosome genes (Kato et al., 2013; Wang et al., 2013).
CRISPR/Cas9 KO of Zfy
Genome editing was first used to produce Zfy KO mice in 2017 (Nakasuji et al., 2017). Three KO models, Zfy1 KO, Zfy2 KO, and Zfy double KO (DKO) were created by disrupting the conserved Zfy exon 4, inducing a frameshift mutation in the mm-ZFY1 and mm-ZFY2 peptide sequences (Fig. 3A and B). Initial fecundity testing revealed that the Zfy1 KO mice were fertile, the Zfy2 KO mice were sub-fertile, and the Zfy DKO mice were infertile. Sperm analyses confirmed that sperm from Zfy DKO males displayed defects in morphology, motility, capacitation, acrosome reaction, and oocyte activation. Attempts to reproduce the Zfy DKO mice via IVF and ICSI were unsuccessful; the DKO sperm were unable to fertilize oocyte in IVF while ICSI yielded embryos with chromosomal abnormalities and poor embryo development in vitro. When transferred to pseudopregnant recipients, the transplanted embryos failed to develop into pups (Nakasuji et al., 2017).
Preliminary transcriptomics and proteomics analyses were performed with Zfy DKO males. Microarray analysis on whole testis from a single Zfy DKO male suggested that Zfy could regulate a group of reproduction-related genes. Mass spectrometry analysis of sperm from a single DKO male revealed that of the 3191 proteins detected, nearly half (1476) were significantly downregulated in DKO sperm when compared to sperm from wild-type males. Of the 62 downregulated proteins with annotated roles in reproduction, four had roles matching the phenotypical abnormalities of Zfy DKO mice: PLCZ1, involved in oocyte activation (Saunders et al., 2002); HTT, required for proper sperm morphology (Yan et al., 2016); and PRSS21 and PLCD4, which are important for the acrosome reaction (Fukami et al., 2001; Yamashita et al., 2008). Downregulation of these proteins in Zfy DKO testis was confirmed by western blot analysis.
The inability to reproduce the Zfy DKO mice via ART posed limitations. All analyses were run with founder ‘F0’ males. These mice could display a phenotype different from an established Zfy DKO colony because they might have been mosaic mutants. Additionally, the KO strategy used to produce the first Zfy KO mice (Nakasuji et al., 2017) did not warrant a complete KO because the disrupted region of Zfy, exon 4, is spliced out in the short Zfy transcript variants (Decarpentrie et al., 2012). Consequently, mm-ZFY1-s, and to a much lesser extent, mm-ZFY2-s, could have been retained in these DKO mice, primarily during zygotene, when the mm-ZFY1-s expression is predominant. This could have resulted in different phenotypes than those caused by a total loss of all isoforms. These limitations could have also affected the transcriptomics and proteomics analyses, which were each performed with only a single Zfy DKO founder male.
We recently revisited the study of Zfy KO mice, aiming to (i) remove the genomic sequences encoding for all ZFY variants and (ii) reproduce these mice with ART to maintain colonies for future transcriptomics and proteomics studies (Yamauchi et al., 2022). Complete removal of each Zfy homologue was verified by genomic sequencing, and the absence of Zfy transcripts was confirmed by RT-PCR. As previously observed, Zfy1 KO mice were fertile, Zfy2 KO mice were sub-fertile, and Zfy DKO mice were completely infertile. In both studies (Nakasuji et al., 2017; Yamauchi et al., 2022), sperm from Zfy2 KO and Zfy DKO males, but not from Zfy1 KO males, were morphologically abnormal. Sperm from Zfy DKO were also shown to display defects in mitochondria, axoneme, and outer dense fibers. However, while we observed a decreased testis size and a drastic decrease in sperm number, with some DKO males lacking epididymal sperm entirely, a previous study reported that the gross appearance of testes and epididymides and sperm number were not affected (Nakasuji et al., 2017). Also, while in our hands ICSI and ROSI with haploid gametes from the DKO males were successful and efficient, Nakasuji et al. (2017) reported that sperm from their DKO males were impaired in their ability to activate oocytes after ICSI and that ICSI-derived embryos had poor developmental potential. These striking differences in sperm production efficiency and ART outcome may be because the DKO mice produced before were on a different genetic background, or because the KO was incomplete and selective in that it removed just the long ZFY isoforms, or due to the fact that only single founder DKO males were examined.
Together, the phenotypic characterization of Zfy KO and DKO mice supports that the presence of at least one Zfy homologue is required for male fertility, that loss of both homologues leads to complete infertility and disturbances in spermatogenesis and sperm function, and that out of the two homologues, Zfy2 is more important for spermatogenesis and sperm function.
Biochemical function of human ZFY and ZFX
A recent study investigated the function of the ubiquitously expressed human ZFY and ZFX, and the closely related ZNF711 genes. In human embryonic kidney (HEK293T) cells, which are of female origin and therefore lack ZFY, three lines were created with CRISPR/Cas9 targeting: ZFX KO, ZNF711 KO, and ZFX/ZNF711 DKO (Ni et al., 2020). Cell proliferation was reduced in single KO lines and severely inhibited in the DKO line. RNA sequencing confirmed significantly altered transcriptomes for all three lines, with DKO cells displaying the most significant changes. Gene ontology (GO) analysis of the differentially expressed genes (DEGs) in the DKO cells suggested that loss of ZFX and ZNF711 was associated with changes in a broad range of cellular functions. Upregulated DEGs were grouped into gene families encoding proteins such as histones, cadherins, and zinc finger transcription factors, whereas downregulated DEGs were grouped into those expressing kinases, ATPases, peptidases, chaperone proteins, and oxidoreductases. Differential gene expression analysis of downregulated DEGs identified ‘cyclins and cell cycle regulation’ as one of the potential pathways in which ZFX and ZNF711 could be involved, consistent with the low proliferation rates observed in the ZFX/ZNF711 DKO cell lines.
Chromatin immunoprecipitation followed by sequencing (ChIP-seq) and its derivative that uses exonuclease (ChIP-exo) were performed for hs-ZFX and hs-ZFN711 in HEK293T cells and for hs-ZFY-l, hs-ZFX, and hs-ZNF711 using male prostate (22Rv1) cells. All three isoforms exhibited nearly identical genome-wide-binding profiles, binding mainly to CpG island (CGI) promoter regions, which typically drive the expression of ubiquitously expressed housekeeping genes (Saxonov et al., 2006). The majority of genomic-binding sites were observed to be downstream of the transcription start site. A strong correlation existed between downregulated DEGs in ZFX/ZNF711 DKO cells and the promoter regions bound by ZFX family genes, whereas no such correlation existed for upregulated DEGs. Thus, hs-ZFX and hs-ZNF711 may act as transcriptional activators for the downregulated DEGs in DKO cells and indirectly regulate the upregulated DEGs. Both hs-ZFY and hs-ZFX proteins have 13 homologous C2H2 DNA-binding zinc finger domains (ZF1–13), and hs-ZFN711 contains all but two domains (ZF3 and ZF7). To determine which were essential for transcriptional activity, hs-ZFX variants encoding varying ZF deletions were transfected to HEK293T cells, and genomic-binding patterns were investigated by ChIP-seq. ZF1–10 were dispensable for hs-ZFX transcriptional activity, with mutants containing only ZF11–13 exhibiting similar genomic binding patterns to those of wild-type hs-ZFX.
This report provides strong evidence that the conserved family of zinc finger genes act as transcriptional factors. The biochemical functions of the testis-specific ZFY isoforms, mm-ZFY1-l, mm-ZFY1-s, mm-ZFY2-l, mm-ZFY2-s, and hs-ZFY-s, remain elusive due to lack of reliable antibodies. However, the DNA-binding ZF domains are conserved across all ZFY/X homologues and transcript variants (Mardon et al., 1990), and it was previously confirmed that the ZF12 and ZF13 domains of mm-ZFY2 recognize specific nucleotide motifs in vitro (Grants et al., 2010). Thus, there is a strong possibility that both long and short ZFY/X proteins exhibit at least some of the same binding patterns in mice as they do in humans. However, their functional interactions between coregulators and genomic-binding sites may differ depending on whether they retain the spliced region of the N-terminal acidic domain.
Functional differences between the two Zfy homologues
We have always been interested in the functional differences between the two mouse Zfy homologues. Most of the studies, which we referred to earlier in this review, indicated that out of the two, Zfy2 plays a more prominent role in spermatogenesis. While the presence of the Sxrb-encoded Zfy2/1 fusion gene (i.e. Zfy1 coding region driven by Zfy2 promoter; Decarpentrie et al., 2012; Fig. 1 and Table 1) had beneficial effects on spermatogenesis progression and spermatid function when compared with males carrying Sry and Eif2s3y as the sole Y genes, it did not reach the level observed in males carrying Sxra with intact copies of Zfy1 and Zfy2 (Vernet et al., 2011; Yamauchi et al., 2014, 2015). The investigations of mice carrying the individual Zfy transgenes, Zfy1/U and Zfy2 as well as known differences in expression level, pointed to Zfy2 having a stronger influence. Zfy2, and not Zfy1, was shown to (i) reinstate apoptotic response at meiosis I (Vernet et al., 2011), (ii) be involved in the initiation of MSCI (Vernet et al., 2016a), (iii) enable transformation of round spermatids into sperm during spermiogenesis (Yamauchi et al., 2015; Vernet et al., 2016b); and (iv) enable formation of sperm functional in ICSI (Yamauchi et al., 2015). Both Zfy1 and Zfy2 were shown to be involved in execution of cells with MSCI failure (Royo et al., 2010). Also, both Zfy1 and Zfy2 (and Zfx) were shown to play a role in meiosis II by facilitating the second meiotic division, with Zfy2 being much more powerful (Vernet et al., 2014). Finally, the phenotypic characterization of Zfy1 KO and Zfy2 KO mice has shown that while the loss of Zfy1 is entirely benign for fertility and spermatogenesis, mice lacking Zfy2 displayed minor fertility impairment and had increased incidence of sperm headshape defects (Nakasuji et al., 2017; Yamauchi et al., 2022). Loss of Zfy1 led to Zfy2 overexpression, which translated to global levels of Zfy1/2 transcripts in Zfy1 KO higher than in Zfy2 KO and comparable to those of wild-type males. Therefore, the differences in the phenotype of both KO models are likely due to quantitative differences, although qualitative differences in Zfy expression and protein isoforms function may also contribute (Yamauchi et al., 2022). Future analyses will clarify whether loss of Zfy1, Zfy2, or both, leads to deregulation of X and Y chromosome encoded genes.
Another insight into the roles of Zfy1 and Zfy2 comes from our new data documenting spermatogenesis progression and assisted reproduction trials with XEOSry males carrying Zfy transgenes (Figure 4). All Zfy transgenic males (XE,Z2OSry, XE,Z1/UOSry, and XEZ1/U,Z2OSry; Fig. 1 and Table 1) had round spermatids identifiable in testis sections which indicated that spermatogenesis was progressing through meiosis (Fig. 4A); yet spermatids did not elongate and arrested at Steps 7–8 of development. When the numbers of round spermatids were quantified on testis sections, all males had significantly fewer cells than XY but no significant differences between the three Zfy transgenics, or between Zfy transgenics and XEOSry males, were noted. However, while live offspring could be obtained after ROSI with the majority of the XE,Z2OSry and of the XEOSry males, round spermatids could not be found in testicular cell suspension for any of the XE,Z1/UOSry males, and for three out of five of XEZ1/U,Z2OSry males (Fig. 4B). Live testicular cell suspension is reflective of the entire germ cell content of each testis, while in the analysis of testis sections, only a few selected tubules are included in scoring. It is thus possible that the randomly selected tubules were not representative of the entire round spermatid population in the testis, the total number of which was below the threshold allowing for identification in cell suspension prior to ROSI.
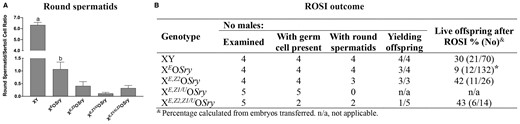
The consequences of Zfy transgenic addition to XEOSry males on spermatogenesis and ART outcome. XE,Z1/UOSry, XE,Z2OSry, and XE,Z1/U,Z2OSry males were examined to assess spermatogenesis progression and spermatid function in ROSI. The XY and XEOSry data are shown as a reference control; these data were published previously (Yamauchi et al., 2014, 2015). (A) PAS/HA-stained testis sections were examined to assess spermatogenesis progression. Three males were examined per genotype; for each male, three randomly selected tubules were examined per stage (XII–I, II–IV, V–VI, VII–VIII, IX–X, and XI), and the numbers of round spermatids and Sertoli cells were counted. The data are expressed as the round spermatid/Sertoli cell ratio. Statistical significance (t-test, P < 0.05): adifferent than all others; bdifferent than XE,Z1/UOSry. (B) Testes from Zfy transgenic males were used to obtain live testicular cell suspension and round spermatids, if found, were used for ROSI. *Different than all other (Fisher’s exact test).
The combined histology and ROSI data offer insights into the putative functional differences between ZFY1 and ZFY2 proteins. The higher success of ROSI with XE,Z2OSry when compared to XEOSry could be interpreted as round spermatids being ‘healthier’ in the presence of Zfy2, even though these cells appeared much less frequently. Because the predominant isoform encoded by Zfy2, mm-ZFY2-l, includes complete transactivating and zinc finger domains and is predicted to be a transcription factor, mm-ZFY2-l could improve spermatid development and function by interacting with transcriptional coregulators and binding to the promoter regions of other reproductive-related genes, thus regulating the expression of meiotic and spermatogenic-related genes. In XEOSry, which lack both Zfy1 and Zfy2, X-encoded Zfx, that maintains the complete transactivating and zinc finger domains, can play a compensatory role for Zfy2 function.
The ‘detrimental’ effect of Zfy1 is less clear, especially considering the phenotype of Zfy KO mice. Since Zfy2 KO males have a modest spermiogenic phenotype when compared to Zfy DKO males, and Zfy1 is overexpressed in Zfy2 KO males, Zfy1 is promoting spermatogenesis and fertility in this context. There seem to be three possibilities explaining the unusual findings obtained with the XE,Z1/UOSry and XEZ1/U,Z2OSry transgenics. First, it is possible that the presence of the Uba1y gene in the Zfy1/Uba1y BAC transgene may play a role in the observed deleterious effect. Second, it cannot be dismissed that the location of the BAC transgene on the X chromosome after random integration may cause some insertional effects. The third possible explanation considers the possible functional differences between Zfy homologues and transcript variants. The transcripts produced by the endogenous Zfy1 (and presumably by the Zfy1/U transgene) during zygotene primarily lack exon 4, whereas postmeiotic Zfy1 transcripts retain exon 4 (Fig. 3A and B). Consequently, in premeiotic cells, the predominant isoform encoded by Zfy1, mm-ZFY1-s, lacks a portion of the transactivating domain while retaining the entire zinc finger region, and thus may bind competitively to the same genomic regions as other ZF-related proteins but fail to provide the same transactivating activity. If true, mm-ZFY1-s would have a dominant negative effect on other ZF-related proteins which are less abundant than mm-ZFY2-l. However, if the biochemical properties of mm-ZFY1-s are different from that of mmZFY1-l and mm-ZFY2-l, it could display a unique profile of protein-binding partners and genomic-binding patterns. Human short isoform (hs-ZFY-s) is testis-specific while the long isoform (hs-ZFY-l) is ubiquitous, suggesting that hs-ZFY-s plays a yet unclear role during spermatogenesis (Page et al., 1987; Decarpentrie et al., 2012). In mice, the production of stable short ZFY proteins remain to be experimentally confirmed. This has not yet been possible due to lack of a good specific antibody against mouse ZFY. Future experiments are needed to distinguish between the three explanations and to pinpoint on the function of ZFY homologues and their splicing variants.
Conclusions
Decades of transgene-rescue studies have shown that Zfy2, and, to a lesser extent, Zfy1, are essential for normal male reproduction. More recently, this assertion has been confirmed with direct targeting of Zfy, and in vitro studies have found that at least hs-ZFY and hs-ZFX can act as transcription factors. The current theory also suggests that Zfy not only plays a crucial role in male reproduction but also in the survival of the Y chromosome throughout eutherians. What remains to be determined is the mechanism by which Zfy1 and Zfy2 contribute to spermatogenesis, and what the functional differences between the homologues and isoforms are. It is also unknown what role human ZFY, especially hs-ZFY-s, plays in spermatogenesis.
Our new ART data suggest that presence of Zfy1 alone in the context of limited Y gene contribution (XE,Z1/UOSry and XEZ1/U,Z2OSry transgenics) may be detrimental to spermatogenesis and mouse fertility. Once validated in future experiments, these data should be of interest to human reproduction because the predominant isoform encoded by Zfy1 during zygotene, mm-ZFY1-s, is homologous to the testis-specific human variant (hs-ZFY-s), whereas the mouse isoforms present postmeiotically, mm-ZFY1-l and mm-ZFY2-l, are homologous to the ubiquitously expressed hs-ZFY. We have suggested that mm-ZFY1-s could bind competitively to other ZF homologues: a hypothesis that could apply to both mice and humans. Thus, the correct ratio of long to short ZFY isoforms may be needed for optimal ZFY function in meiosis I. Uncovering the functional differences between Zfy1 and Zfy2 can offer insight into how hs-ZFY-l and hs-ZFY-s contribute to reproduction in humans.
Due to the intricacies of the biochemical and molecular mechanisms behind meiosis and spermatogenesis, the only way to identify the function of ZFY will be to develop antibodies specific to the testis-specific isoforms (mm-ZFY1-l, mm-ZFY1-s, mm-ZFY2-l, mm-ZFY2-s, and hs-ZFY-s) and to perform antibody-based assays to determine ZFY genomic-binding sites and protein coregulators. ZFY function can also be investigated if mouse models with mouse or human ZFY isoforms specifically tagged are produced. The existing ChIP-seq data on hs-ZFY and hs-ZFX provide some insight to ZFY function as a transcription factor. However, the limitation of these data is that they were not obtained with male germ cells, and ZFY may behave differently in vivo than in cultured cells.
In sum, we hope that we have presented sufficient evidence supporting that the Y chromosome Zfy/ZFY gene is indeed a ‘returning spermatogenesis hero’ and that this evidence will inspire future explorations of these important zinc finger proteins.
Data availability
The data underlying this article are available in the article.
Acknowledgements
We thank Frédéric Chalmel and the UrGenT team (Irset, Rennes) for their help with scRNA-Seq analyses.
Authors’ roles
Conceptualization: H.H. and M.A.W.; unpublished data acquisition: Y.Y. and V.R.; unpublished data analysis and interpretation: M.A.W.; original draft preparation: H.H. and M.A.W.; review and editing: H.H., J.C., and M.A.W.; supervision: M.A.W. and J.C.; funding acquisition: M.A.W. All authors have read and agreed to the published version of the manuscript.
Funding
This research was funded by National Institute of Health, grants HD072380 and HD106936 and Hawaii Community Foundation, grants 17CON-86294 and 14ADVC064546 to M.A.W. The Histology Core at the University of Hawaii is supported by an NIH U54MD007601 grant. H.H. is a recipient of 2022–2023 STEM Chateaubriand Fellowship.
Conflicts of interest
The authors declare no conflict of interest.
References
Author notes
Present address for Victor A Ruthig: Sexual Medicine Lab, Department of Urology, Weill Cornell Medicine, 1300 York Ave, New York, NY, USA.