-
PDF
- Split View
-
Views
-
Cite
Cite
Zhaoheng Zhang, Jiayue Qu, Min Lu, Xinyu Zhao, Yang Xu, Li Wang, Zhongjia Liu, Yingying Shi, Chaotian Liu, Yipu Li, Chao Wang, Mingliang Xu, Zhangjie Nan, Qingqin Cao, Jinbao Pan, Wende Liu, Xinrui Li, Qingpeng Sun, Weixiang Wang, The maize transcription factor CCT regulates drought tolerance by interacting with Fra a 1, E3 ligase WIPF2, and auxin response factor Aux/IAA8, Journal of Experimental Botany, Volume 75, Issue 1, 1 January 2024, Pages 103–122, https://doi.org/10.1093/jxb/erad372
- Share Icon Share
Abstract
Plants are commonly exposed to abiotic stressors, which can affect their growth, productivity, and quality. Previously, the maize transcription factor ZmCCT was shown to be involved in the photoperiod response, delayed flowering, and quantitative resistance to Gibberella stalk rot. In this study, we demonstrate that ZmCCT can regulate plant responses to drought. ZmCCT physically interacted with ZmFra a 1, ZmWIPF2, and ZmAux/IAA8, which localized to the cell membrane, cytoplasm, and nucleus, respectively, both in vitro and in vivo in a yeast two-hybrid screen in response to abiotic stress. Notably, ZmCCT recruits ZmWIPF2 to the nucleus, which has strong E3 self-ubiquitination activity dependent on its RING-H2 finger domain in vitro. When treated with higher indole-3-acetic acid/abscisic acid ratios, the height and root length of Y331-ΔTE maize plants increased. Y331-ΔTE plants exhibited increased responses to exogenously applied auxin or ABA compared to Y331 plants, indicating that ZmCCT may be a negative regulator of ABA signalling in maize. In vivo, ZmCCT promoted indole-3-acetic acid biosynthesis in ZmCCT-overexpressing Arabidopsis. RNA-sequencing and DNA affinity purification-sequencing analyses showed that ZmCCT can regulate the expression of ZmRD17, ZmAFP3, ZmPP2C, and ZmARR16 under drought. Our findings provide a detailed overview of the molecular mechanism controlling ZmCCT functions and highlight that ZmCCT has multiple roles in promoting abiotic stress tolerance.
Introduction
Drought and soil salinity are the major adverse environmental conditions encountered by plants, and drought in particular is one of most detrimental abiotic stressors in plant production (Gong et al., 2020; Ding et al., 2023). Under drought stress, abscisic acid (ABA) is known to stimulate short-term responses, such as the closure of stomata to maintain the plant’s water balance, and long-term growth responses through the regulation of stress-responsive genes (Zhang et al., 1987). For example, Arabidopsis DET1-DDB1-ASSO-CIATED1 (DDA1) is a substrate receptor for CULLIN4-RING E3 ubiquitin ligase (CRL4)-COP10-DET1-DDB1 (CDD) complexes, which facilitate the proteasomal degradation of PYL8 (Irigoyen et al., 2014). The homeodomain transcription factor HB33 is repressed by auxin response factor2 (ARF2), which may modulate the crosstalk between the ABA and auxin signalling pathways (Wang et al., 2011). ABA signalling regulates many developmental stress responses, including those to drought, cold, or high salinity, and some important APETALA2/ethylene response factor (AP2/ERF) families and auxins are also involved in drought responses (Stockinger et al., 1997; Finkelstein et al., 1998; Liu et al., 1998).
Auxins are phytohormones, which are widely acknowledged as regulators of plant development. However, several recent studies have also highlighted their roles in stress responses. Auxins associate with ethylene to regulate root development and architecture, which are key aspects of drought and salinity tolerance (Kohli et al., 2013; Wu et al., 2017; Yang and Qin, 2022). Two types of auxin signalling are involved in the regulation of auxin responses. One of these, the auxin response factor (ARF) family, functions by binding to auxin response elements (AuxREs) on the promoters of auxin response genes, to activate or repress gene responses to auxin and recruit a second family of transcription factors, Aux/IAA repressors, which confer an auxin response to the genes. ARFs bind specifically to TGTCTC AuxREs in the promoters of their target genes, which dimerize with ARF activators (Luo et al., 2018).
Auxins associate with ethylene to regulate root development and architecture, which are key aspects of drought and salinity tolerance (Kohli et al., 2013; Wu et al., 2017; Yang and Qin, 2022). Aux/IAA proteins harbour four conserved characteristic domains, I, II, III, and IV. Domain I contains an LxLxL motif and domain II contains a GWPPV motif; these are the core binding site for TIR/AFB proteins and auxin, respectively (Luo et al., 2018). Several studies have shown that auxin treatment stimulates the interaction between SCFTIR1 and auxin/IAA proteins and promotes their ubiquitination for degradation (Gray et al., 2001; Shani et al., 2017). It has been demonstrated that Aux/IAA proteins physically interact with Class A ARFs in the absence of auxin (Luo et al., 2018; Wang et al., 2021).
The first Aux/IAA genes, PS-IAA4/5 and PS-IAA6, were isolated from pea in 1993 (Theologis et al., 1985; Oeller et al., 1993), and this gene family has now been identified in 30 plant species by genome-wide analysis. In Arabidopsis, most Aux/IAA proteins display similar phenotypes for processes such as embryo development, lateral root initiation and elongation, hypocotyl growth, tropism, and flower organ development (Liscum and Reed, 2002; Overvoorde et al., 2005). These Aux/IAA proteins can interact with auxin signalling components, transcription factors, and signalling proteins.
Maize is one of the world’s most important crops, and its production is significantly affected by salt and drought stress (Mao et al., 2015; Wang et al., 2021). A growing number of studies have found that tolerance response genes play important roles in maize plant growth. ZmNAC111 enhances drought tolerance via RNA-directed DNA methylation and H3K9me2 (Mao et al., 2015). ZmbZIP72 can be induced by high salt and drought stress, and transgenic Arabidopsis can show enhanced resistance to stress (Ying et al., 2012). In addition, ZmbZIP71 and ZmbZIP81 are both induced by exogenous drought stress and are part of the stress response pathway (Liu and Wang, 2011; Wang et al., 2014). Lu et al. (2012) found that, in maize, ZmSNAC1 participates in the self-regulatory mechanisms that occur in response to drought. Our recent study inferred that ZmNAP, ZmNAC19, ZmNAC4, ZmJUB1, and ZmNAC87 respond to drought and ABA treatments (Ding et al., 2023). Ma et al. (2018) found that transgenic maize overexpressing ZmbZIP4 has a stronger root system (e.g. a longer primary root), resulting in enhanced drought tolerance. There are 34 Aux/IAA genes in maize, and some members of this family have been characterized in detail (Matthes et al., 2019). A previous study reported that BIF1 and BIF4 encode Aux/IAA proteins, which dynamically regulate the expression of BARREN STALK1 (BA1), a basic helix-loop-helix (bHLH) transcriptional regulator necessary for axillary meristem formation that shows a striking boundary expression pattern (Galli et al., 2015). The maize Aux/IAA protein RUM1 (ROOTLESS WITH UNDETECTABLE MERISTEM 1) is a key regulator of lateral and seminal root formation (Zhang et al., 2016). Ramos Báez et al. (2020) used the nuclear Auxin Response Circuit reconstructed in yeast (Saccharomyces cerevisiae) system to functionally annotate maize auxin signalling components, and found that auxin/indole-3-acetic acid (IAA) repressor proteins were degraded in response to auxin, with rates that depended on both receptor and repressor identities, and maize auxin/indole-3-acetic acids were able to repress AUXIN RESPONSE FACTOR transcriptional activity. Most studies on Aux/IAAs have focused on Arabidopsis, whereas the information on the post-translational regulation of Aux/IAA proteins in response to changes in abiotic stresses such as drought, salt stress, and nutrient use efficiency remains limited in maize.
Previous studies have revealed that ZmCCT, a homologue of the rice photoperiod response regulator gene Ghd7, functions as a flowering repressor in maize (Xue et al., 2008; Hung et al., 2012; Yang et al., 2013; Ku et al., 2016; Tang et al., 2022; Gou et al., 2023). ZmCCT is the causal gene at the qRfg1 locus, and a polymorphic CACTA-like transposable element (TE1) ~2.4 kb upstream of ZmCCT is the genetic determinant of allelic variation (Hung et al., 2012; Yang et al., 2013). The CACTA-like transposon represses ZmCCT expression to reduce photoperiod sensitivity (Yang et al., 2013). Our previous study also demonstrated that the non-TE1 ZmCCT allele is a stalk rot quantitative disease resistance gene, which was promptly induced by a rapid but transient change in histone methylation, leading to disease resistance when challenged with Fusarium graminearum (Wang et al., 2017). This chromatin-based regulatory mechanism enables ZmCCT expression to be regulated in a more precise and timely manner in its defence responses against F. graminearum infection (Wang et al., 2017; Tang et al., 2022). ZmCCT protein contains a conserved CCT (CO, COL, and TOC1) domain at its C-terminus, which is widely involved in the regulation of flowering and plays an important role in plant growth and development (Jin et al., 2018; Tong et al., 2022). Some CCT domain genes, such as ZmCOL3, ZmCCT9, ZMM4, ZmMADS69, ZmCCA1, ZmCCA1a, and Zea mays CENTRORADIALIS 8 (ZCN8), play key roles in flowering time regulation (Danilevskaya et al., 2008; Huang et al., 2018; Jin et al., 2018; Liang et al., 2019). ZmCOL3 can repress flowering time by activating the expression of ZmCCT (Jin et al., 2018). A recent study also showed that ZmCCT has a dual function in regulating maize flowering time and stress responses under long-day conditions (Su et al., 2021). Although ZmCCT has been cloned, the mechanism of regulation of the signalling pathways in cells in response to both biotic and abiotic stresses remains unclear.
In this study, we demonstrate the drought-tolerant property of the non-TE1 ZmCCT allele compared with the TE1 allele. When ZmCCT was overexpressed in maize and Arabidopsis, the plants exhibited obvious stress when exposed to drought. Moreover, ZmCCT was also shown to physically and directly associate with ZmWIPF2, ZmAux/IAA8, and ZmFra a1. ZmCCT can recruit cytoplasm-localized ZmWIPF2, via ubiquitination activity, to the nucleus when co-transfected into cells and regulate downstream stress-induced auxin and drought-related targeted gene expression. The results improve our understanding of how plants sense drought and how the signal is translated to the nucleus to initiate the transcription of target genes in response.
Materials and methods
Plant materials
Y331 and Y331-ΔTE are a pair of maize near-isogenic lines as previously described (Wang et al., 2017; Tong et al., 2022). Y331-ΔTE and Y331 share ~99.9% identity in their genetic background, with Y331 being based on a GoldenGate 3KSNP (Illumina, USA). Y331 and 83B28H1 are susceptible near-isogenic lines, and Y331-∆TE and 83B28H5 are stalk rot-resistant near-isogenic lines (Wang et al., 2017; Tong et al., 2022). Y331 and 83B28H1contain a transposable element (TE) insertion in the promoter, whereas Y331-ΔTE and 83B28H5 lack a TE insertion (non-TE ZmCCT) (Tong et al., 2022).
Construction of expression vectors and generation of transgenic plants
The full-length coding sequence of ZmCCT was amplified by PCR using specific primers (listed in Supplementary Table S1) from B73 and inserted into the binary vector pCAMBIA3301 driven by the maize CaMV35S promoter using a ClonExpressII One Step Cloning Kit (Vazyme, China) to construct the enhanced ZmCCT expression vector, p35S::ZmCCT-OE. The recombinant vector was transformed into the KN5585 maize inbred line via shoot-tip transformation using Agrobacterium tumefaciens strain EHA105, and transformed into Arabidopsis thaliana via floral-dip transformation using Agrobacterium strain GV3101. Positive transformants were selected using glufosinate ammonium (Sigma, USA) and identified by PCR using specific primers (Supplementary Table S1). For β-glucuronidase (GUS) staining, Arabidopsis seedlings at 10 d after germination were selected and transferred to GUS staining solution according to a previously described method (Ye et al., 2019)
Maize and Arabidopsis drought tolerance assays
Two pairs of maize near-isogenic lines, Y331 and Y331-ΔTE, and 83B28H1 and 83B28H5, were used in the drought tolerance experiment. The seedling substrate was mixed with water and put into a pot with a diameter of 36 cm to ensure the same quality of the substrate used in each pot. Two kinds of near-isogenic plant materials were planted in the same pot. Three parallel replications were planted in each treatment, and five plants were planted in each replicate. When the plants had reached the three-leaf stage, they were watered thoroughly, after that they were not watered again (i.e. the drought treatment began); a normally watered control group was provided with an appropriate amount of water every day. ZmCCT-overexpressing maize plants were normally cultured until the V10 stag, and were then subjected to drought; 14 d after the start of the drought treatment, the plants were re-watered and their ability to grow and recover was observed.
The survival rate (SR), leaf relative water content, water loss rate (WLR), photosynthesis rate (PS), transpiration rate (TR), stomatal conductance (SC), chlorophyll content, shoot dry weight (SDW), shoot fresh weight (SFW), root dry weight (RDW), root fresh weight (RFW), and root length (RL) were used as indicators of drought resistance. The PS, TR, and SC, of the T5 transgenic and wild-type (WT) plants were measured on fully expanded leaves of seedlings at the three-leaf stage using a CIRAS-3 Portable Photosynthesis System (PP SYSTEMS, USA). Photosynthetic performance was recorded before and after the drought treatment. Statistical analysis was based on data obtained from five seedlings for each plant line and the experiment was repeated twice. For the Arabidopsis drought tolerance assays, 7-day-old plants grown under a 16 h light/8 h dark photoperiod at constant 25°C (photosynthetically active radiation, 350 μmol m–2 s–1) were transferred into pots containing 250 g of soil. Five-week-old plants growing under favourable water conditions were exposed to drought stress. Water was withheld from the plants for 12-14 d. Watering was then resumed to allow the plants to recover. Two days later, the number of surviving plants was recorded. At least 15 plants of each line were compared with WT plants in each test, and statistical data were based on data obtained from three independent experiments (Ding et al., 2023).
Exogenous application of naphthylacetic acid and abscisic acid
Y331 and Y331-ΔTE seedlings were used to measure the effects of exogenous naphthylacetic acid (NAA)/ABA on seedling root growth. Seedlings were grown to the V1 stage, then moved to 1/2 Hoagland solution for 24 h; an air pump was used to introduce air, which was maintained at 28 °C (day)/24 °C (night), with a day/night ratio of 16 h/8 h. The next day, the solution was changed to Hoagland solution, and the solution was subsequently changed every 2 d. The treatments were carried out on seedlings at the three-leaf stage: seedlings were treated with exogenous hormones at concentrations of 0 (control), 1, 3, and 5 µM.
Yeast two-hybrid library screen and interaction assay
The coding sequence of the target gene was cloned into the vector pGBKT7, and the plasmid library of pGADT7 was screened. The two vectors were respectively equipped with LUE2 and TRP1 genes, and the strains containing the two vectors were plated on leucine- and tryptophan-deficient medium. The two vectors were co-transformed into AH109 yeast receptor cells. The mated yeast cells were selected on SD-Leu-Trp-His-Ade plates. Bait cDNAs were cloned into the pGBKT7 vector, and prey cDNAs were cloned into the pGADT7 vector. Both prey and bait constructs were transformed into the yeast strain AH109 and plated on SD-Leu-Trp medium. Protein–protein interactions were determined by the growth of yeast on SD-Leu-Trp-His-Ade medium.
Luciferase complementation assay
The coding sequences of the interacting protein gene and ZmCCT were cloned into the 35S::IP-cLUC vector and the 35S::ZmCCT-nLUC vector. The vectors were transformed into Agrobacterium strain EHA105, and the concentration of the bacterial solution was adjusted before co-infiltration of Nicotiana benthamiana leaves. Luciferase complementation assays were performed 48 h later. Luciferase (LUC) activity was determined by using a Tanon (5200 Multi) imaging system.
In vivo co-immunoprecipitation assay
The coding sequences of the genes ZmFra a 1, ZmWIPF2, and ZmAux/IAA8 were cloned into 35S::ZmAux/IAA8-HA and 35S::ZmCCT-GFP vectors and expressed in the Escherichia coli system. Total proteins were extracted and incubated with GFP-Trap Agarose (Chromotek, USA) for at least 2 h at 4 °C. After immunoprecipitation, the proteins were detected by western blotting.
Protein purification from E. coli and E3 ubiquitin ligase activity assay
Full-length cDNAs of ZmWIPF2 were expressed as His-tagged proteins in the Rosetta (DE3) strain of E. coli. The protein was affinity purified with ProteinIso Ni-NTA Resin (TransGen Biotech, China). In vitro ubiquitination reactions were performed by adding 1 µg of His-ZmWIPF2, 40 ng of wheat (Triticum aestivum) E1, 100 ng of A. thaliana E2 (UBC10), 1 µg of ubiquitin, and 1.5 µl of 20× reaction buffer (1 M Tris–HCl, pH 7.5, 40 mM ATP, 100 mM MgCl2, 40 mM DTT, 600 mM creatine phosphate, and 1 mg m–1 creatine phosphokinase). The reaction was incubated at 30 °C for 1.5 h in a 30 µl reaction volume before being stopped with the SDS sample loading buffer and then heated to 100 °C for 5 min. Samples were visualized by western blotting.
Protein regulation assay in N. benthamiana
Briefly, 30 h after the desired constructs were co-transfected into N. benthamiana leaves, 100 μM MG132 was added to the N. benthamiana leaves, and 18 h later the leaves were harvested for protein extraction. Total protein was extracted with denaturation buffer [50 mM Tris–HCl, pH 7.5, 150 mM NaCl, 0.5% (v/v) NP40, 4 M urea, and 1 mM phenylmethylsulfonyl fluoride]. Protein abundance was detected by immunoblotting and normalized to N. benthamiana actin levels in each sample. Sample were visualized by western blotting.
Subcellular localization
For analysis of subcellular localization, the coding sequence of ZmCCT was cloned into the vector (35S::ZmCCT-GFP); the coding sequence of ZmAux/IAA8, ZmFra a 1, or ZmWIPF2 was cloned into the vector (35S::IP-mCherry). Plasmids were introduced into Agrobacterium strain EHA105 for transient infiltration into N. benthamiana leaves. Fluorescence was imaged using a Zeiss LSM880 confocal microscope at 48 h after infiltration of the leaves, which were placed in a nucleus-staining solution (1 mM DAPI) for 3 h before observation. All were images captured with the following parameters: excitation wavelengths: green fluorescent protein (GFP) 488 nm, mCherry580 nm, DAPI 360 nm, chloroplast 640 nm; emission wavelengths: GFP 507 nm, mCherry 610 nm, DAPI 460 nm, chloroplast 675 nm).
Western blot analysis
Proteins were extracted from maize leaves with extraction buffer (50 mM Tris–HCl, pH 7.5, 0.5 M sucrose, 1 mM MgCl2, 10 mM EDTA, pH 8.0, 5 mM DTT, and protease inhibitor cocktail). Samples were transferred onto polyvinylidene fluoride membranes at 100 V for 1.5 h, blocked with 5% non-fat blocking-grade milk (Bio-Rad, USA) and incubated with primary antibodies, including anti-Ubiquitin (1:5000, Biomol, USA), anti-His (1:5000, Transgen, China), anti-Actin (for plant, 1:5000, Sigma, USA), anti-GFP (1:5000, EASYBIO, China), and anti-haemagglutinin (HA) (1:5000, Abcam, UK). Primary antibodies were derived from mouse. The membranes were then incubated with horseradish peroxidase-conjugated secondary antibodies derived from goat (1:1000, EASYBIO, China) and analysed with Immobilon Western HRP Substrate (Sigma, USA).
RNA-sequencing analysis
For maize RNA-sequencing (RNA-seq) analysis, pooled leaf tissues from three maize seedlings at the three-leaf stage were collected from Y331 and Y331-ΔTE plants before or after drought treatment. Total RNA from the maize leaf samples was extracted using TRIzol reagent (Invitrogen, USA). The total amount and integrity of RNA were assessed using the RNA Nano 6000 Assay Kit of the Bioanalyzer 2100 system (Agilent Technologies, USA).
Total RNA was used as the input material for RNA sample preparations. Briefly, mRNA was purified by using poly(T) oligo-attached magnetic beads. First-strand cDNA was synthesized using random hexamer primers and M-MuLV Reverse Transcriptase. The library fragments were purified with an AMPure XP system (Beckman Coulter, USA). Then, the PCR product was purified by using AMPure XP beads, and the library was sequenced by using the Illumina NovaSeq 6000.
Quantitative reverse transcription PCR
Partial total RNA in RNA-seq was used for reverse transcription. First-strand cDNA was synthesized from 1 μg of total RNA with a PrimeScript RT Reagent Kit (TaKaRa, Japan). For quantitative reverse transcription (qRT)–PCR, reactions were conducted on a QuantStudio 5 instrument (Applied Biosystems, USA) with TB Green Premix Ex Taq II (TaKaRa, Japan). Primers were designed with Primer Premier5 software. GAPDH was used as a reference gene. Relative gene expression was analysed by the 2-ΔΔCT method (Livak and Schmittgen, 2001). Each expression analysis was carried for three times. All primer sequences are listed in Supplementary Table S1.
DNA affinity purification-sequencing and electrophoretic mobility shift assay
The ZmAux/IAA8 coding sequence was inserted into the pFN19K (HaloTag) T7 SP6 Flexi expression vector (Promega). The Halo-ZmCCT or Halo-ZmAux/IAA8 fusion protein was expressed using the TNT SP6 Coupled Wheat Germ Extract System (Promega, USA) and then purified with Magne HaloTag Beads (Promega, USA). Genomic DNA (gDNA; 5 μg in 130 μl TE buffer) was fragmented to an average of 200 bp using a Covaris M220 (Woburn, USA) according to the manufacturer’s recommended settings. The fragmented gDNA was then purified using MICH DNA Clean Beads (Bluescape, China) at a DNA-to-beads ratio of 0.7–1.1. The beads were incubated with the gDNA for 5 min at room temperature and then placed on a magnet (Bluescape, China) to immobilize the beads. The supernatant was then removed. The beads were washed twice with 200 μl of 80% ethanol and allowed to dry. Once dry, they were resuspended in 22 μl resuspension buffer, incubated at room temperature for 5 min, and placed on the magnet. The DNA-containing supernatant was then transferred to a new tube. Libraries were constructed using the MICH TLX DNA-seq Kit (Bluescape, China) according to the manufacturer’s instructions. After incubating the beads with bound proteins with DNA fragments at room temperature for 1 h, they were washed three times with wash buffer to remove unbound DNA fragments. The HaloTag beads were resuspended in 30 μl elution buffer and then heated at 98 °C for 10 min to denature the protein and release the bound DNA fragments into the solution.
Biotin-labelled probes for ZmAux/IAA8 were synthesized by Bluescape (Hebei, China). The electrophoretic mobility shift assay (EMSA) was done with a LightShift Chemiluminescent EMSA kit (Thermo Scientific, USA). The primers used are listed in Supplementary Table S1. The control reactions used Biotin-EBNA Control DNA and EBNA protein.
Bioinformatics analysis
RNA-seq data of 150 bp paired-end raw reads were trimmed and quality filtered by using trim_galore, and mapped to the maize transcriptome (NCBI B73-REFERENCE NAM-5.0) using Salmon software. Differentially expressed genes (DEGs) were called using the R package DESeq2 with an s-value threshold of 0.005 (Love et al., 2014). Gene Ontology (GO) analysis was performed using the R package clusterProfiler (Yu et al., 2012). DNA affinity purification-sequencing (DAP-seq) data of ZmAux/IAA8 were pre-processed in a similar way to the RNA-seq reads, and aligned to maize genome using Bowtie2 software (Langmead and Salzberg, 2012). DAP-seq peaks were called using MACS2 software with a q-value threshold of 1E-8 (Zhang et al., 2008).
Structure analyses in silico
Model structures of ZmWIPF2 and ZmAux/IAA8 proteins were predicted using AlphaFold2 (Jumper et al., 2021). The model ZmWIPF2 presents three identifiable domains, an N-terminal zinc finger domain (amino acids 85–121), a single α-helix structure (amino acids 163–181), and a RING domain (amino acids 195–259). The model ZmAux/IAA8 shows an identifiable (PB1) domain in its C-terminus (amino acids 112–220). The pLDDT metric value of most identifiable domains in ZmWIPF2 and ZmAux/IAA8 is ≥85% indicative of reliability a priori compared with the experiment (Jumper et al., 2021).
Phylogenetic tree construction
The full-length amino acid sequences of 94 IAA transcription factor-encoding genes identified in maize, rice, and Arabidopsis were aligned using the MEGA version 7 program with default parameters. A phylogenetic tree was constructed based on this alignment result using the neighbour-joining method in MEGA version 7 with the following parameters: Poisson correction, pairwise deletion, uniform rates, and bootstrap (1000 replicates).
Statistical analysis
Datasets were analysed using Prism 6 software (GraphPad Software). Data are presented as means ±SD or means ±SE. Statistical significant differences were determined using Student’ s t-test.
Results
ZmCCT-dependent drought tolerance under drought stress
Our previous work showed that ZmCCT mediates both photoperiod sensitivity and stalk rot resistance (Yang et al., 2013; Wang et al., 2017). To explore whether ZmCCT plays an important role in drought stress, we evaluated the drought tolerance phenotypes of the Y331/Y331-ΔTE and 83B28H1/H5 haplotype inbred lines. Water was withheld from plants grown in pots for approximately 20 d, after which the Y331 and 83B28H1 lines started to display more severe signs of leaf curling and wilting compared with the ZmCCT-isogenic lines (Fig. 1A). After re-watering for a 2 d recovery period, the Y331-ΔTE inbred lines and 83B28H5 haplotype lines had higher survival rates (33.03% and 27.50%, respectively) than the Y331 and 83B28H1 lines (23.00% and 12.5%, respectively) (Fig. 1A, B). The relative water content (RWC) and water loss rate (WLR) of detached leaves were measured as additional indicators of drought tolerance. The detached leaves from Y331-ΔTE and 83B28H5 plants had a higher RWC and a lower WLR compared with the leaves of Y331 and 83B28H1 (Fig. 1C, D).
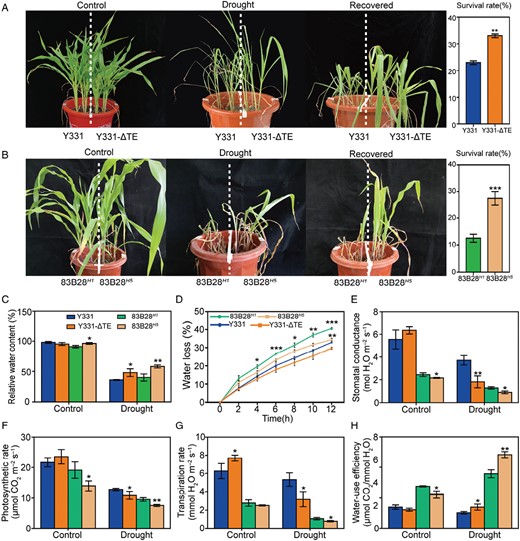
Tolerance of two pairs of maize near-isogenic lines to drought. (A, B) Phenotypes of Y331/Y331-ΔTE and 83B28H1/83B28H5, two pairs of maize near-isogenic lines, in response to drought. Photographs were taken under well-watered conditions and after a drought treatment followed by re-watering 2 d afterwards. The mean percentage survival and SE were calculated from three independent experiments. (C) Leaf relative water content of the control and drought-treated plants. (D) Water loss rate from second leaves. Leaves were detached, kept at 24 °C, and weighed every hour for 12 h. (E–G) Comparison of the photosynthetic performance of two pairs of maize near-isogenic lines during drought stress: (E) stomatal conductance, (F) photosynthesis rate, (G) transpiration rate, and (H) water-use efficiency. Data are means ±SD of at least three biological replicates. Significant differences were determined using unpaired Student’s t-tests and are indicated with asterisks (*P<0.05, **P<0.01, ***P<0.001).
Next, the PS, TR, and SC over the 20 d period of drought were compared between the Y331, Y331-ΔTE, 83B28H1, and 83B28H5 inbred lines. Under drought conditions, the physiological parameters were comparable between the Y331/Y331-ΔTE and the 83B28H1/83B28H5 haplotype lines (Fig. 1E–G). After 20 d of drought treatment, the TR of the Y331-ΔTE and 83B28H5 lines initially decreased, which resulted in a greater water-use efficiency (WUE, calculated as PS in relation to TR) (Fig. 1F–H). The PS was also significantly decreased in the stress-tolerant lines Y331-ΔTE and 83B28H5 compared with Y331 and 83B28H1, respectively (Fig. 1F). Thus, the WUE of the Y331-ΔTE and 83B28H5 lines was greater than that of the Y331 and 83B28H1 lines, respectively (Fig. 1E–H).
Furthermore, the chlorophyll content (measured as SPAD value), RL, RFW, SFW, SDW, and RDW were used to assess the pleiotropic effects of drought tolerance. Both Y331-ΔTE and 83B28H5 seedlings with non-TE1 ZmCCT showed better growth potential under drought treatment compared with the Y331 and 83B28H1 seedlings, respectively (Supplementary Fig. S1). The RL, SPAD, and SDW of the two near-isogenic lines showed the most significant decline, with the RL of Y331 and Y331-ΔTE decreasing by 34.9% and 29.7% (Supplementary Fig. S1B), the SPAD value decreasing by 73.0% and 69.7% (Supplementary Fig. S1A), and the SDW decreasing by 53.3% and 52.7%, respectively (Supplementary Fig. S1E). These data suggest that plants expressing ZmCCT exhibited a better WUE under water deficit conditions than plants lacking functional ZmCCT, thus indicating that ZmCCT enhanced drought tolerance in the maize seedlings.
ZmCCT overexpression enhances seedling drought tolerance in maize and Arabidopsis
Transgenic plants were generated using the maize KN5585 line and Arabidopsis, with the full-length coding sequence of ZmCCT, and confirmed by PCR (Supplementary Fig. S2). Seven T0OE-ZmCCT transgenic plants were obtained from three independent transgenic experiments. For transgenic maize, the phenotypes of two independent 35S::ZmCCT lines were analysed (Fig. 2A). In comparison with WT plants transformed with the empty vector, the T5 transgenic lines (OE-ZmCCT) had significantly higher physiological parameters of SR and leaf RWC (Fig. 2B, C). Furthermore, the OE-ZmCCT transgenic plants exhibited significantly lower rates of WLR, TR, SC, and PS compared with the WT (Fig. 2D–G). These results highlight the consistent functions of ZmCCT in mediating the response to drought in young maize plants.
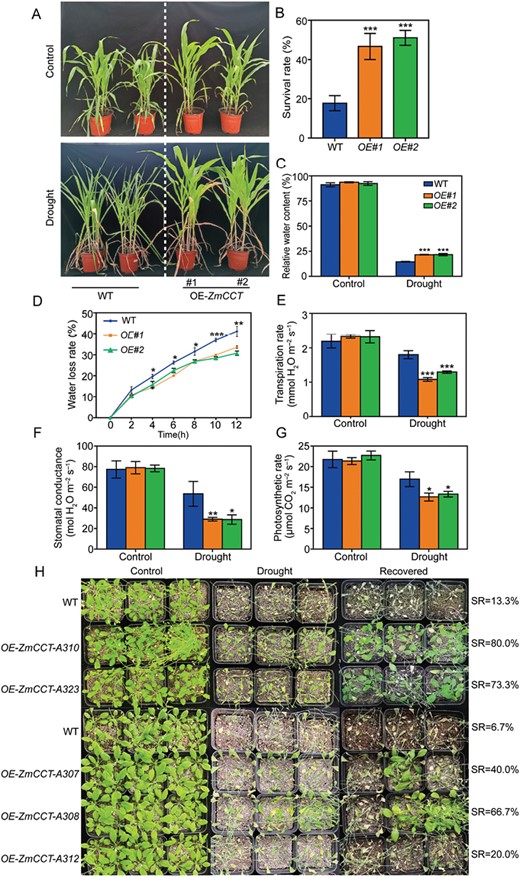
Tolerance of the maize and Arabidopsis ZmCCT-overexpressing lines to drought. (A) Phenotypes of the maize ZmCCT-overexpressing lines in response to drought. Photographs were taken under well-watered conditions and after drought treatment. (B) Statistical analysis of the survival rates after drought treatment and recovery. The mean percentage survival and SE were calculated from three independent experiments. (C) Leaf relative water contents of control and drought-treated plants. (D) Water loss rate from second leaves. Leaves were detached, kept at 24 °C, and weighed every 2 hours for 12 h. (E–G) Comparison of the photosynthetic performance of the maize ZmCCT-overexpressing lines during drought treatment: (E) transpiration rate, (F) stomatal conductance, and (G) photosynthesis rate. (H) Drought tolerance evaluation of ZmCCT-overexpressing Arabidopsis plants and survival rates after drought treatment and recovery. Data are the means ±SD of at least three biological replicates. Significant differences were determined using unpaired Student’s t-tests and are indicated with asterisks (*P<0.05, **P<0.01, ***P<0.001).
In total, 29 independent transgenic Arabidopsis lines (OE-ZmCCT) were randomly selected for drought tolerance analysis. Five independent T3 transgenic lines (OE-ZmCCT-A307, OE-ZmCCT-A308, OE-ZmCCT-A312, OE-ZmCCT-A310, and OE-ZmCCT-A323) and WT plants were further selected for 14 d of drought treatment to verify the functions of ZmCCT in the plant response to drought (Supplementary Fig. S2C, D). A similar improved drought tolerance phenotype in transgenic lines A310 and A323 was observed (Fig. 2H). Under drought treatment, the transgenic lines displayed less severe wilting compared with the WT plants; after re-watering for a 2 d recovery period, 80.0%, 73.3%, 40.0%, 66.7%, and 20.0% of the T3 transgenic lines survived, whereas the SR of the WT sibling plants was only 13.3% and 6.7%, respectively (Fig. 2H). These results suggest that ZmCCT-overexpressing Arabidopsis plants are more tolerant to drought than WT plants.
ZmCCT physically interacts with ZmFra a 1, ZmWIPF2, and ZmAux/IAA8 in vivo and in vitro
To further identify the mechanism by which ZmCCT regulates stress tolerance in plants, a yeast two-hybrid library screening assay was conducted to identify the factors interacting with ZmCCT under abiotic stress conditions. The full-length coding sequence (amino acids 1–240, ZmCCT), N-terminal sequence (amino acids 1–117, ZmCCT-N), and C-terminal sequence (amino acids 118–240 aa, ZmCCT-C) were each inserted into the vector pGBKT7 (Fig. 3A, B) and used to transform yeast. The results showed that the full-length coding sequence and the ZmCCT-N exhibited self-activation, but ZmCCT-C did not (Supplementary Fig. S3). A maize water stress tolerance yeast library was used to identify ZmFra a 1 (Zm00001d043314), ZmWIPF2 (Zm00001d037650, Zm00001d031741, NCBI 103643126), and ZmAux/IAA8 (Zm00001d039624) (Fig. 3C, D).
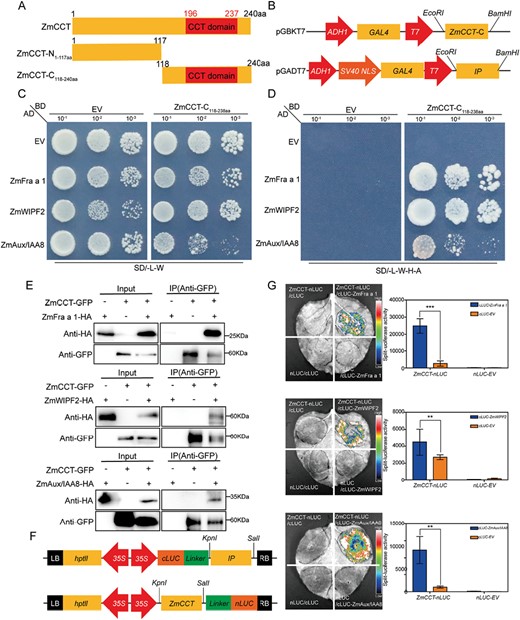
The ZmCCT protein interacts with ZmFra a 1, ZmWIPF2, and ZmAux/IAA8. (A) Diagram of the conserved CCT domain and full-length coding sequence truncation for the ZmCCT protein. Schematic diagrams indicate the various constructs encoding full-length or truncated ZmCCT. (B–D) ZmCCT interacts with ZmFra a 1, ZmWIPF2, and ZmAux/IAA8 in yeast. Equal amounts of yeast cells expressing the indicated bait (BD; pGBKT7) and prey (AD; pGADT7) constructs were plated on SD/-Leu-Trp and SD/-Leu-Trp-His-Ade medium. (E) Co-immunoprecipitation assay demonstrating the interactions between ZmCCT and ZmFra a 1, ZmWIPF2, and ZmAux/IAA8 in Nicotiana benthamiana. ZmCCT-GFP was co-expressed with ZmFra a 1-HA, ZmWIPF2-HA, and ZmAux/IAA8-HA in N. benthamiana. All constructs were driven by the CaMV 35S promoter. Total proteins were extracted, and ZmCCT-GFP was precipitated with anti-GFP agarose. ZmCCT and the ZmFra a 1, ZmWIPF2, and ZmAux/IAA8 proteins were separated by immunoblot and detected with anti-GFP and anti-HA antibodies, respectively. (F) Schematic diagrams of the vector constructs used for luciferase complementation assay (LCA). (G) LCA showing the physical interactions between ZmCCT and ZmFra a 1, ZmWIPF2, and ZmAux/IAA8. Leaf disks of N. benthamiana were used to measure the luminescence 48 h after the infiltration of Agrobacterium carrying the indicated constructs. Three independent experiments were carried out with similar results. Significant differences are indicated with asterisks (**P<0.01, ***P<0.001; Student’s t-test).
Furthermore, co-immunoprecipitation assays were conducted in N. benthamiana leaves co-transfected with constructs encoding ZmCCT-GFP and ZmFra a 1-HA, ZmWIPF2-HA, and ZmAux/IAA8-HA, respectively (Fig. 3E). The results showed that ZmCCT protein could directly interact with ZmFra a 1-HA, ZmWIPF2-HA, and ZmAux/IAA8-HA proteins. Luciferase complementation assays were used to confirm the direct interactions between ZmCCT and ZmFra a 1, ZmWIPF2, and ZmAux/IAA8, respectively, in vivo (Fig. 3F, G). The luciferase complementation assays showed strong LUC activity when N. benthamiana leaves were co-infiltrated with ZmCCT-nLUC and ZmFra a 1-cLUC constructs, whereas we detected no LUC activity with co-infiltration of the negative controls (Fig. 3G). We also observed strong LUC activity with ZmCCT-nLUC and ZmWIPF2-cLUC, and with ZmCCT-nLUC and ZmAux/IAA8-cLUC (Fig. 3G). These results confirmed that ZmCCT protein can interact strongly with ZmFra a 1, ZmWIPF2, and ZmAux/IAA8 both in vivo and in vitro.
ZmCCT co-localizes with ZmWIPF2 and ZmAux/IAA8 in vivo and promotes the nuclear translocation of ZmWIPF2
The co-infiltration of N. benthamiana leaves with full-length 35S::ZmFra a 1-mCherry and 35S::OsPIP1-GFP constructs led to strong red fluorescent protein (RFP) signals in the cell membrane and co-localization with OsPIP1-GFP, a cell membrane protein that has been used as a marker for the membrane system in rice (Fig. 4A). In contrast, co-infiltration of the ZmCCT-GFP and ZmAux/IAA8-mCherry constructs resulted in significant signals in the nuclei of the cells (Fig. 4B, C). Notably, when the ZmWIPF2-mCherry construct was infiltrated, RFP signals were observed in the cell membrane, while co-infiltration of the ZmWIPF2-mCherry and ZmCCT-GFP constructs resulted in co-localized signals in the nucleus of the cell, suggesting that ZmCCT is required for the nuclear translocation of ZmWIPF2 (Fig. 4B).
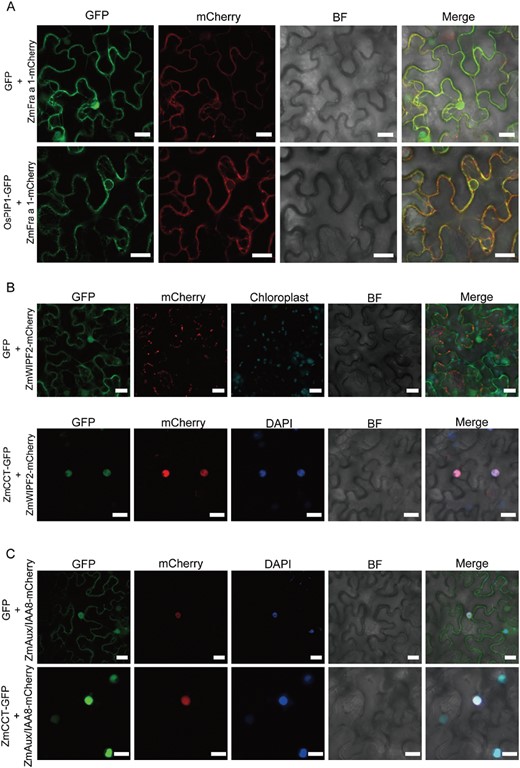
Subcellular localization of ZmCCT with ZmFra a 1, ZmWIPF2, and ZmAux/IAA8. (A) 35S::ZmFra a 1-mCherry or the empty vector and the proteins that localize on the membrane 35S::OsPIP1-3-GFP were co-transfected into the leaves of Nicotiana benthamiana. (B) 35S::ZmWIPF2-mCherry and (C) 35S::ZmAux/IAA8-mCherry or the empty vector and 35S::ZmCCT-GFP were co-transfected into the leaves of N. benthamiana. The leaves were subsequently incubated in 1 mM DAPI for 3 h before observation. Scale bars=20 μm. BF, bright field.
ZmFra a 1 is an Fra A 1-associated protein. ZmWIPF2 is a highly conserved family member 2 (WIPF2), which has a RING-H2 domain. ZmAux/IAA8 is an auxin-responsive protein. The Aux/IAA gene family encodes nuclear proteins that share four highly conserved domains (I–IV) (Luo et al., 2018; Wang et al., 2021). Phylogenetic analysis showed that ZmAux/IAA8 encodes 344 amino acids sharing >78% and 71% identity with rice OsIAA1 and OsIAA15, respectively (Supplementary Fig. S4A). Amino acid sequence alignments of the ZmAux/IAA8 proteins showed highly conserved domains I, II, III, and IV, suggesting that these proteins may play important roles in regulating the auxin response cascade (Supplementary Fig. S4B).
ZmWIPF2 has autoubiquitination activity and is dependent on its RINGC215C218 domain for activity
The full-length structure of ZmWIPF2, which is a member of the E3 ligase family, was predicted using AlphaFold2 (https://github.com/deepmind/alphafold). ZmWIPF2 was found to contain a RING_Ubox superfamily domain (by using https://www.ncbi.nlm.nih.gov/Structure/cdd/cdd.shtml to predict the conserved domain). ZmWIPF2 has a RING-H2 domain located at its C-terminus (amino acids 215–256) (Fig. 5A). A four-cysteine motif seizing Zn2+ was observed in the zinc finger domain (Supplementary Fig. S4C).
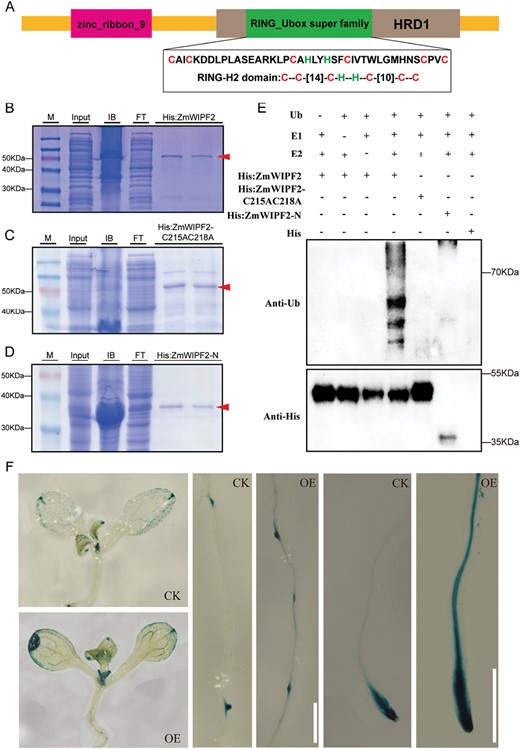
E3 ubiquitin ligase assay and protein domain analysis for ZmWIPF2. (A) Diagram of the conserved domain and full-length coding sequence of the ZmWIPF2 protein. (B–D) His:ZmWIPF2, His:ZmWIPF2 C215AC218A, and His:ZmWIPF2-N mutant fusion proteins purified from Escherichia coli and identified by SDS-PAGE. (E) Self-ubiquitination activity assay of ZmWIPF2. (F) Arabidopsis 10-day-old ZmCCT-overexpressing seedlings (OE) displayed more intense GUS staining in the leaf margin, lateral root primordia, and root tips compared with control (CK) seedlings. Scale bars=0.5 mm.
The structure of ZmAux/IAA8 was then modelled using Alphafold2. The N-terminal region of ZmAux/IAA8 (amino acids 1–111) was found to include an EAR motif, a KR motif, a GWPPV motif, and a bipartite nuclear localization signal, which, as anticipated, is completely flexible in this structure (Supplementary Fig. S4D). The C-terminal region of ZmAux/IAA8 (amino acids 112–220) contains a Phox and Bem1p (PB1) domain (Luo et al., 2018). The PB1 domain in ZmAux/IAA8 showed a globular ubiquitin-like structure and revealed a β-grasp fold with 5β strands surrounded by 3α helices, similar to the reported ARF5/7 and IAA17 structures in Arabidopsis (Shani et al., 2017). Moreover, 2β strands (152–167, located between α1 and β3) were observed in the modelled structure (Supplementary Fig. S4D). The structure of the sequence region between α1 and β3 is distinct and dynamic in the solved PB1 domain structures; thus, the exact function of these additional 2β strands is unknown.
To further determine whether ZmWIPF2 possesses E3 ubiquitin ligase activity and whether this activity is dependent on the RING domain, an in vitro E3 ubiquitin ligase assay was conducted that included a series of negative controls lacking E1 or E2 (Fig. 5E). A point mutant ZmWIPF2 C215AC218A was constructed with a cysteine-to-alanine mutation in the RING domain, an N-terminal sequence (amino acids 1–214, ZmWIPF2-N) mutant protein, and the ZmWIPF2 protein in the activity assay (Fig. 5B–D). His:ZmWIPF2, His:ZmWIPF2 C215AC218A, and His:ZmWIPF2-N mutant fusion proteins were purified from E. coli and incubated with wheat (T. aestivum) E1, Arabidopsis E2 (UBC10), ubiquitin (Ub), and ATP, respectively. Immunoblot analysis showed that the signal of polyubiquitin products with high molecular weights was detected only in the presence of all the components, suggesting that ZmWIPF2 is an active E3 ubiquitin ligase. However, in the reactions with the ZmWIPF2 C215AC218A and ZmWIPF2-N mutant proteins, the E3 ubiquitin ligase activity was completely abolished (Fig. 5E), suggesting that an intact RING finger domain is required for the E3 ubiquitin ligase activity of ZmWIPF2 in vitro. Collectively, these results suggest that ZmWIPF2 functions as an E3 ligase and that its E3 activity is dependent on the C2H2 RING finger domain. It was previously suggested that Aux/IAA proteins are targeted for degradation by SCFTIR1 (an SCF ubiquitin protein ligase) in response to auxin (Gray et al., 2001).
We further speculated that ZmCCT and ZmAux/IAA8 may be substrates of ZmWIPF2. The Agrobacterium strain carrying the GFP:ZmCCT construct was co-infiltrated with the strain containing the ZmWIPF2:HA or ZmAux/IAA8:HA construct into N. benthamiana leaves, and the total protein was extracted from the treated leaves after 48 h. However, no E3 activity was detected on ZmCCT or ZmAux/IAA8, which were the possible substrates (Supplementary Fig. S5). Furthermore, we ectopically overexpressed ZmCCT in DR5:GUS Arabidopsis (Ulmasov et al., 1997; Ye et al., 2019). The transgenic lines have a significant increase in IAA content, which resulted in increased GUS activity in the shoots, lateral root primordia, and root tips (Fig. 5F). These findings indicate that ZmCCT positively regulates IAA biosynthesis.
ZmCCT disruption impairs the tolerance response to drought at the transcriptomic level
To investigate the role of ZmCCT in drought tolerance at the gene transcriptome scale, RNA-seq of drought-treated and control strains was performed for the Y331 and Y331-ΔTE genotypes (Supplementary Fig. S6). RNA-seq showed that there were 2951 up-regulated genes and 3265 down-regulated genes in drought-treated Y331-ΔTE in comparison to the control Y331-ΔTE plants. For these up-regulated and down-regulated genes, the degree of transcriptional change was modest in the drought-treated Y331 plants (Fig. 6B). Among the 2951 genes up-regulated in response to drought in Y331-ΔTE, 1600 were also activated in Y331, while a large proportion (1351/2951) was specifically activated in Y331-ΔTE. Similarly, genes that were responsive to drought in Y331-ΔTE were partly down-regulated in Y331 (Fig. 6A).
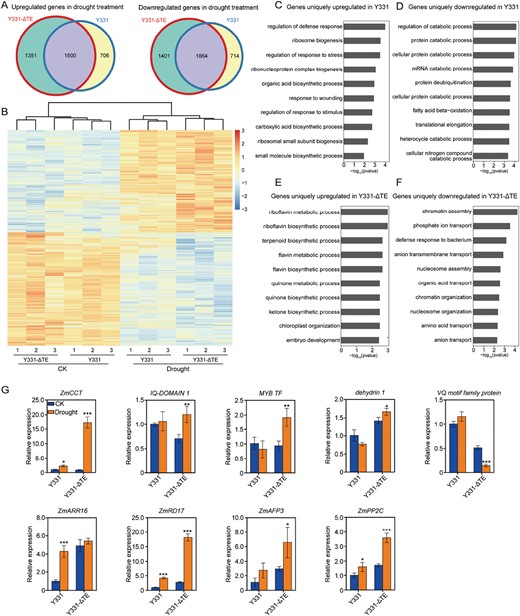
Transcriptomic analysis of the maize near-isogenic lines Y331 and Y331-ΔTE. (A) Venn diagram showing up-regulated and down-regulated genes between the Y331 and Y331-ΔTE plants under control and drought conditions. (B) Hierarchical clustering of the differentially expressed genes in lines Y331 and Y331-ΔTE. The indicated scale is the log2 value of the normalized level of gene expression. (C–F) Gene Ontology terms of the biological pathways enriched in the transgenic lines based on the up-regulated or down-regulated genes. (G) qRT–PCR verification of the increases in gene expression associated with plant drought responses and tolerance in the maize near-isogenic lines under control and drought conditions. Data are means ±SD. Significant differences are indicated with asterisks (**P<0.01, **P<0.001, ***P<0.001; Student’s t-test).
The transcriptomes of the Y331-ΔTE and Y331 plants were compared under both favourable and drought conditions (Fig. 6C–F; Supplementary Fig. S7). GO enrichment analysis showed that biological pathways responsive to defence response and stimulus response regulation were greatly enriched among the identified genes uniquely up-regulated in Y331, whereas those responsive to protein deubiquitination and the regulation of catabolic processes were especially enriched among the genes uniquely down-regulated in Y331, compared with the untreated samples (Fig. 6C, D). Under drought treatment, the expression of ZmCCT was much higher in Y331-ΔTE than in Y331 (Fig. 6G). Although some genes responsive to riboflavin metabolic process and riboflavin biosynthetic process were greatly enriched among the up-regulated genes in Y331-ΔTE, and those responsive to chromatin assembly and phosphate ion transport were enriched among the down-regulated genes (Fig. 6E, F), there were several well-known drought-inducible genes, such as ZmRD17 (LOC100281087), ZmAFP3 (LOC100280792) and ZmPP2C (LOC103641516) in Y331-ΔTE inbred lines, and ZmARR16 (LOC100217235) in Y331 inbred lines (Supplementary Table S2). In contrast, some new genes were identified, such as dehydrin1 (LOC542373), MYB (LOC100272675), a VQ motif family protein gene (LOC100280842), and a protein IQ-DOMAIN 1 gene (LOC103641958). We verified the significantly changing expression patterns of these genes in Y331-ΔTE inbred lines using qRT–PCR (Fig. 6G), and hypothesized that these transcriptomic changes may contribute to the early reduction in TR, quick stomatal closure, and better protection of the photosynthetic machinery in ZmCCT inbred lines when responding to drought. Most of the identified genes are involved in drought tolerance, ABA responsiveness, and defence responses. These results suggest that drought responsiveness is greatly impaired in plants in which the expression level of ZmCCT is insufficient.
Altered ZmCCT expression increased sensitivity to exogenous NAA/ABA in maize and positively regulated IAA-response gene expression in Arabidopsis
Given that ZmCCT interacts with ZmAux/IAA8, and ZmAux/IAA8 may be an auxin-responsive protein with multiple auxin-response elements in the promoter region, we investigated whether the expression level of ZmCCT could affect the sensitivity of maize near-isogenic lines exposed to exogenous NAA. The performance of the Y331 and Y331-ΔTE seedlings grown for 2 weeks in Hoagland medium with or without NAA or ABA was observed (Fig. 7). There were no significant differences in plant height and RDW between Y331 and Y331-ΔTE plants grown in Hoagland medium without NAA (Fig. 7B, G). However, the RL, RFW, SFW, and SDW of Y331-ΔTE plants grown in Hoagland medium with 5 µM NAA were all significantly higher than those of the control Y331 inbred lines (Fig. 7C–F). The same experiment was performed using exogenous ABA. There were no significant differences in plant height and RDW between Y331 and Y331-ΔTE plants grown in Hoagland medium without ABA (Fig. 7H–N). Plant height, SFW, and RDW of Y331-ΔTE plants grown in Hoagland medium with higher concentrations of ABA were significantly higher than those of the control Y331 inbred lines (Fig. 7I, L, M). These results demonstrate that ZmCCT enables maize near-isogenic lines to be more hypersensitive to NAA and ABA, indicating that ZmCCT may be a regulator of NAA and ABA signalling in maize.
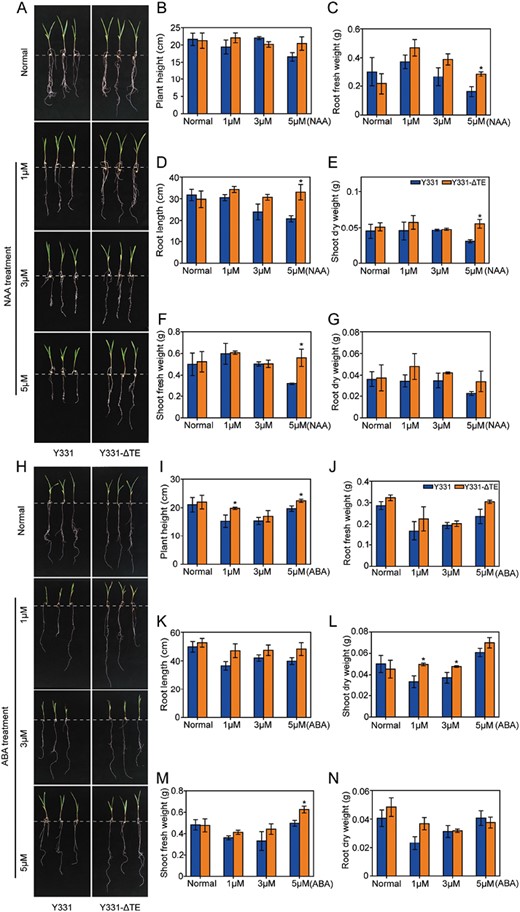
Slight reduction in ABA and NAA sensitivity in the maize near-isogenic lines Y331 and Y331-ΔTE during their seedling development stages. Growth performance of Y331 and Y331-ΔTE maize plants grown in Hoagland solution containing 0, 1, 3, or 5 µM NAA (A–G) or ABA (H–N). Shoot and root lengths and fresh/dry weights of the maize plants are shown in panels B–G and I–N. Data are means ±SE (n=3). Asterisks indicate significant differences between Y331 and Y331-ΔTE (*P<0.05; by Student’s t-test).
ZmAux/IAA8 directly binds to and regulates some of the drought-response genes
To investigate the genomic regions of Aux/IAA8 protein binding, we performed DAP-seq of ZmAux/IAA8. The DAP-seq assay produced approximately 91 million reads with two biological replicates (Fig. 8A). Approximately 68% of the two peak sets were consistent (Supplementary Fig. S8A). We also plotted the read number located within the consensus peak set (the 8128 union peaks), which showed a high correlation of read density of peaks between the two replicates (r=0.98; Supplementary Fig. S8B). Of these, approximately 86 million reads were uniquely mapped to the maize reference genome with a 95% effective mapping ratio. A total of 4227 DAP-seq peaks were obtained that were ZmAux/IAA8 (q≤1E-8) genome wide and are potential in vivo binding sites. Of the detected peaks, approximately 16% (676 peaks) were located in genic regions, including the promoter (+1 kb upstream to –1 kb downstream of the TSS), 5ʹ and 3ʹ untranslated regions, intron, and exon. A majority of the ZmAux/IAA8 binding peaks were distal to the genic regions (83.58% intergenic; Fig. 8C), which suggests that ZmAux/IAA8 and related proteins may function in distal regulatory regions, such as enhancers. A 10 bp binding motif was also obtained from the top 1000 binding peaks (Fig. 8B). A total of 548 peaks across the entire maize genome in the two biological replicates were significantly associated with one motif, CCCCAGCATT (P-value <1E-90), and the 60 most enriched metabolic pathways among the genes with ZmAux/IAA8 binding sites were identified by DAP-seq (Supplementary Fig. S9). To confirm whether ZmAux/IAA8 could bind to the CCCCAGCATT motif, an EMSA was performed with a purified recombinant ZmAux/IAA8 protein in E. coli and labelled DNA probes containing the ZmAux/IAA8 binding sites. As shown in Fig. 8E, ZmAux/IAA8 protein could bind to the CCCCAGCATT motif. The addition of 20× and 50× unlabelled competitors reduced the detected binding sites of ZmAux/IAA8. These results clearly confirmed the specific binding of the ZmAux/IAA8 protein to the CCCCAGCATT motif.
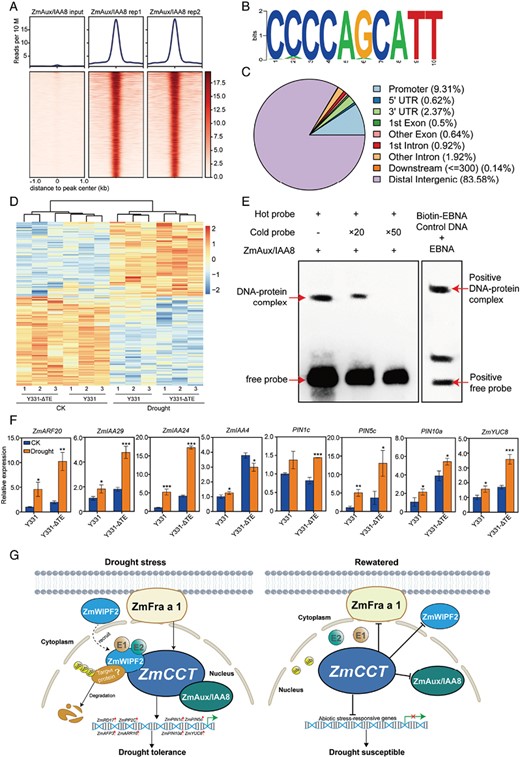
DAP-seq analysis of ZmAux/IAA8 target genes. (A) Heatmaps of input and ZmAux/IAA8 density in the region ±1 kb around the ZmAux/IAA8 peaks. (B) ZmAux/IAA8 binds to the CCCCAGCATT motif as identified by MEME-ChIP. (C) Distribution of the ZmAux/IAA8 binding sites in the maize V5 genome. (D) Heatmap showing the 230 differentially expressed genes with the drought treatment in Y331-ΔTE CK, control condition. (E) Results of EMSA confirming the binding of ZmAux/IAA8 to the CCCCAGCATT motif. Biotin-EBNA control DNA can bind to EBNA protein. (F) qRT–PCR verification of the increased gene expression involved in plant IAA responses in the maize near-isogenic lines under control and drought conditions. Data are means ±SD. Significant differences are indicated with asterisks (**P<0.01, **P<0.001, ***P<0.001; Student’s t-test). (G) A proposed working model for ZmCCT-mediated molecular mechanisms for drought resistance in maize. Under drought, ZmCCT has a positive feedback effect on IAA biosynthesis and regulates drought tolerance by interacting with ZmFra a 1, ZmWIPF2, and ZmAux/IAA8 to regulate the expression of auxin response genes in maize. ZmCCT presumably acts as an integral regulator, suggesting that it has multiple roles in activating plant responses to abiotic stress.
To identify potential target genes, the nearest promoters were identified within a 50 kb distance from the ZmAux/IAA8 binding peaks. The results showed 2775 genes in 100 kb, 2303 genes in 50 kb, and 1000 genes in 10 kb (Supplementary Table S3). These potential target genes are mainly involved in cellular protein localization, protein polyubiquitination, and the regulation of stress responses (Supplementary Table S3). To further validate the expression levels of these genes as putative targets directly regulated by ZmAux/IAA8, the overlapping genes between the DEGs that were differentially expressed upon drought treatment in the Y331-ΔTE strains were analysed. Among the 2303 genes corresponding to the nearest 50 kb promoters, 230 genes were differentially expressed upon drought treatment in Y331-ΔTE. Evidently, the trends of these gene expression changes were weakened in the Y331 strains, in which ZmCCT was non-functional (Fig. 8D). GO analysis for these 230 DEGs indicated that they are likely involved in processes such as circadian rhythm, protein folding, rhythmic process, and response to temperature stimulus (Supplementary Fig. S10). In addition, auxin-related genes such as PIN1c (LOC103641958), PIN5c (LOC100273056), PIN10a (LOC100383548), ZmYUC8 (LOC103649553), ZmARF20 (LOC103626605), ZmIAA29 (LOC107522023), ZmIAA24 (LOC100280600), and ZmIAA4 (LOC103634643) were verified as DEGs in Y331-ΔTE inbred lines using qRT–PCR (Fig. 8E). These results showed that ZmAux/IAA8 directly binds to a proportion of the genes that are responsive to drought treatment, and these expression responses were impaired in ZmCCT-deficient strains.
Discussion
More than 70% of the maize-growing areas in China are threatened by drought (Min et al., 2016; Jin et al., 2019). Excessive exposure to drought leads to water loss from plant cells, resulting in osmotic and oxidative stress (Aslam et al., 2015). Drought reduces the leaf area of plants and also leads to leaf wilting (Ma and Zhou, 2018). Under such conditions, leaves cannot normally provide the required organic matter for plant growth and, consequently, the yield is reduced (Avramova et al., 2015; Dordas et al., 2018).
ZmCCT has multiple roles in both biotic and abiotic stress responses in maize
ZmCCT is a quantitative disease-resistance gene with multiple functions in plant defence (Yang et al., 2010; Gou et al., 2023). Hung et al. (2012) first identified ZmCCT in maize using genome-wide association and targeted high-resolution linkage mapping, and their results proved that ZmCCT plays a negative role in the regulation of flowering time. Yang et al. (2013) identified a CACTA-like transposable element insertion in the promoter of ZmCCT, which resulted in photoperiod sensitivity and accelerated the post-domestication spread of maize. In 2017, we demonstrated that ZmCCT is quantitatively involved in resistance to Gibberella stalk rot, with rapid and bivalent histone H3K4me3 and H3K27me3 epigenetic modifications during pathogen infection (Wang et al., 2017). Further to this, metabolomic analysis revealed that many chemical compounds were altered in pathways associated with phenylpropanoid biosynthesis and phenylalanine metabolism, which may play key roles in conferring resistance to Gibberella stalk rot (Tang et al., 2022). ZmCCT is a CCT domain-containing gene and a homologue of the rice photoperiod response regulator Ghd7, which regulates grain number, plant height, and heading date (Li et al., 2022; Sun et al., 2022). The triple complex of Ghd8–OsHAP5C–Ghd7 directly binds to the promoter of Hd3a and down-regulates the expression of Ehd1, Hd3a, and RFT1, resulting in delayed heading (Li et al., 2022; Sun et al., 2022). Thus, Ghd7 and/or DTH8 weaken the promotion effect of Hd1. In the long term, the combination of Ghd7, DTH8, and Hd1 synergistically suppresses the Ehd1–Hd3a–RFT1 pathway to varying degrees (Sun et al., 2022). Here, we proved that ZmCCT can interact with ZmFra a 1, which is an Fra a1-associated protein (Fig. 3). In strawberry, Fra a1 belongs to the group of pathogenesis-related 10 (PR-10) proteins and is a homologue of the major birch pollen Bet v 1 and apple allergen Mal d 1 (Franz-Oberdorf et al., 2017). PR-10 proteins are part of the highly complex plant inducible defence mechanism and are expressed in response to biotic (pathogen infection) and abiotic (wounding, salt, heat) stressors. Our results showed that ZmFra a 1 is localized in the cell membrane, suggesting that ZmFra a 1 may function as a cell membrane allergen to sense pathogens and abiotic stress. Further studies could help to interpret the molecular mechanisms of ZmCCT and how it interacts with ZmFra a 1 during pathogenesis and other environmental stress conditions. Recent studies have shown that the ZmCCT locus underlies both stalk rot resistance and photoperiod sensitivity in maize (Su et al., 2021). The ZmCCT haplotype H5 was also found to improve yield, stalk rot resistance, and drought tolerance in maize (Tong et al., 2022). We speculate that ZmCCT co-activator proteins, or some key enzymic proteins, may play multiple roles in regulating downstream genes in stalk rot resistance, drought tolerance, high salt tolerance, low nitrogen tolerance, and/or other abiotic stresses. Our results clearly indicated that ZmCCT is involved in stress tolerance. Both the Y331-ΔTE inbred lines, including the full ZmCCT allele and 83B28H5 haplotype lines, showed significant responses to drought treatment (Figs 1, 2). Consistent with the results of a previous study, the T0OE-ZmCCT transgenic plants in our study also showed a significant increase in plant height but decreased fertility compared with WT KN5585 plants (Supplementary Fig. S2), possibly due to the responses of ZmCCT to photoperiod regulation.
Our RNA-seq analysis showed that ZmCCT may regulate several well-known drought-inducible genes, such as ZmRD17, ZmAFP3, ZmPP2C, ZmRD29B, and ZmARR16. These results are highly consistent with those of previous studies that reported the promotion of ZmRD17, ZmAFP3, ZmPP2C, and ZmARR16 expression by drought (Mao et al., 2015; Su et al., 2021). We also identified a few genes that have not been previously reported to respond to drought (Fig. 6G). These results clearly suggest that ZmCCT expression improves stress tolerance by regulating the downstream stress-response genes.
ZmCCT–ZmWIPF2–ZmAux/IAA8 may function as an E3 ubiquitin ligase complex for auxin feedback in plant drought tolerance
During plant drought tolerance, the majority of the auxin-responsive genes were also suppressed after the induction of systemic acquired resistance; enhanced resistance to disease would necessitate the repression of auxin signalling (Shani et al., 2017). A recent report also showed that ZmAuxRP1 is an auxin-regulated protein that promotes IAA biosynthesis while suppressing the biosynthesis of benzoxazinoids. Consequently, elevated ZmAuxRP1 transcript levels result in high IAA and low benzoxazinoid levels (Ye et al., 2019).
Aux/IAA genes are rapidly induced by auxin, and Aux/IAA proteins are targeted by transport inhibitor response1/auxin receptor F-box proteins (Kepinski and Leyser, 2004), which are involved in the ubiquitin-mediated degradation of tagged proteins. A previous study showed that OsIAA18 is an Aux/IAA transcription factor gene involved in salt and drought tolerance. The overexpression of OsIAA18 up-regulated the genes involved in the ABA biosynthesis and signalling pathways, the proline biosynthesis pathway, and the reactive oxygen species-scavenging system in rice plants exposed to salt stress and drought (Wang et al., 2021). In this investigation, ZmAux/IAA8 was found to be homologous to rice OsIAA1 and OsIAA15. ZmAux/IAA8 shares more than 78% and 71% amino acid identity with OsIAA1 and OsIAA15, respectively (Guo et al., 2021). OsIAA1 is a rice Aux/IAA family member involved in auxin and brassinosteroid hormone responses and plant morphogenesis (Song et al., 2009). Our previous multi-omics analysis also showed up-regulation of genes in the ZmCCT-containing Y331-ΔTE line as a response to the salicylic acid signalling pathway and auxin signalling pathway when inoculated with F. graminearum, among which the auxin-related genes AFB6, PIF4, and SAUR56 were activated, while ARF7, ARF24, and syntaxin SYP132 were decreased during pathogenesis (Tang et al., 2022). In this study, the amino acid sequence alignment of ZmAux/IAA8 proteins revealed the highly conserved domains I, II, III, and IV, suggesting that these proteins may play important roles in regulating the auxin response cascade. ZmWIPF2 is a novel protein and its biochemical activity has not been previously identified. In this study, we did not detect E3 ubiquitin ligase activity of ZmWIPF2 on ZmCCT or ZmAux/IAA8. Further assays will be conducted to identify the substrates of ZmWIBF2.
Overexpression of ZmCCT in maize resulted in another interesting phenotype, that of increased root growth in Y331-ΔTE compared with Y331 inbred lines in response to the exogenous application of NAA or ABA (Fig. 7). The results showed that ZmCCT regulates drought tolerance via the plant phytohormones IAA and ABA. The role of ABA in plant responses to drought stress has been widely studied and it is known to play important roles in plant adaptations to environmental stress conditions. Under osmotic conditions, there may be crosstalk between ABA and IAA in ZmCCT-containing strains that enable drought tolerance. Further studies are required to further elucidate this.
Our results show that ZmCCT can directly interact with ZmFra a 1, ZmWIPF2, and ZmAux/IAA8 in response to abiotic stress conditions, which suggests that ZmCCT might function in a complex with ZmFra a 1, ZmWIPF2, and ZmAux/IAA8 (Fig. 3; Fig. 8G). ZmWIPF2 contains a conserved C3H4 RING finger domain, and we detected strong autoubiquitination activity of ZmWIPF2 in an in vitro assay. Moreover, although ZmCCT can interact with ZmWIPF2, we did not detect any E3 activity on either ZmCCT or ZmAux/IAA8, which showed that there must be other subunits or co-activators for ZmWIPF2 E3 ubiquitin activity during downstream Aux/IAA target gene expression (Fig. 8; Supplementary Fig. S5A, B). In addition, we did not detect any interactions between ZmWIPF2 and ZmAux/IAA8. Based on the genetic and biochemical evidence presented in this study, we propose a working model for the potential role of the ZmCCT in regulating drought stress in maize. In this model, ZmCCT has a positive feedback effect on IAA biosynthesis, which is associated with the environmental factors that regulate auxin gene expression and is dependent on ZmFra a 1, ZmWIPF2, and ZmAux/IAA8 protein interactions to facilitate stress tolerance (Fig. 8G).
Supplementary data
The following supplementary data are available at JXB online.
Fig. S1. Determination of the physiological indices of the maize near-isogenic lines Y331 and Y331-ΔTE under drought stress.
Fig. S2. Acquisition and identification of ZmCCT-overexpressing lines.
Fig. S3. Transcriptional activation activity assay for ZmCCT.
Fig. S4. Evolutionary tree for ZmAux/IAA8 and protein structure prediction for ZmWIPF2.
Fig. S5. Degradation of ZmCCT or ZmAux/IAA8 in the presence of ZmWIPF2 in Nicotiana benthamiana.
Fig. S6. Heat map showing the maize transcriptomic analysis results from Y331-ΔTE and Y331 under the CK and drought conditions.
Fig. S7. Hierarchical clustering of the genes commonly up-regulated and down-regulated by ZmCCT in Y331 and Y331-ΔTE.
Fig. S8. Binding sites analysis of ZmAux/IAA8 between two biological replicates.
Fig. S9. GO annotation of the targeted genes bound by ZmAux/IAA8 protein.
Fig. S10 Gene Ontology analysis of the 230 DEGs upon drought treatment in Y331-ΔTE strains.
Table S1. Primer sequences used in this study.
Table S2. Differentially expressed genes in the Y331 and Y331-ΔTE plants under drought treatment.
Table S3. The potential target genes to the ZmAux/IAA8 binding peaks.
Author contributions
WW designed the project; WW, ZZ, JQ, ML, XZ, ZL, YS, CL, YX, LW, YL, CW, MX, ZN, QC, QS, JP, WL, and XL performed the experiments; WW and ZZ performed the data analysis and interpretation of the results; WW and ZZ wrote the manuscript.
Conflict of interest
The authors have no conflicts to declare.
Funding
This work was supported by the National Natural Science Foundation of China (grant 31871638 to WW), the Special Scientific Research Project of Beijing Agriculture University (YQ201603), the Research Fund for Academic Degree & Graduate Education of Beijing University of Agriculture (2019YJS037), the ‘Youth Talent Support Plan’ of Beijing Educational Committee (CIT&TCD201904053), and the Research Fund of the State Key Laboratory for Biology of Plant Diseases and Insect Pests (SKLOF202102).
Data availability
The raw data of RNA-seq and DAP-seq are deposited in the NCBI GEO database under accession number GSE240962 (https://www.ncbi.nlm.nih.gov/geo/query/acc.cgi?acc=GSE240962). All other data supporting the findings of this study are available within the paper and within its supplementary data published online.
References
Author notes
Zhaoheng Zhang, Jiayue Qu and Min Lu These authors contributed equally to this work.
Comments