-
PDF
- Split View
-
Views
-
Cite
Cite
Xin-Yuan Huang, Da-Wei Hu, Fang-Jie Zhao, Molybdenum: More than an essential element, Journal of Experimental Botany, Volume 73, Issue 6, 15 March 2022, Pages 1766–1774, https://doi.org/10.1093/jxb/erab534
- Share Icon Share
Abstract
Molybdenum (Mo) is an essential element for almost all living organisms. After being taken up into the cells as molybdate, it is incorporated into the molybdenum cofactor, which functions as the active site of several molybdenum-requiring enzymes and thus plays crucial roles in multiple biological processes. The uptake and transport of molybdate is mainly mediated by two types of molybdate transporters. The homeostasis of Mo in plant cells is tightly controlled, and such homeostasis likely plays vital roles in plant adaptation to local environments. Recent evidence suggests that Mo is more than an essential element required for plant growth and development; it is also involved in local adaptation to coastal salinity. In this review, we summarize recent research progress on molybdate uptake and transport, molybdenum homeostasis network in plants, and discuss the potential roles of the molybdate transporter in plant adaptation to their local environment.
Introduction
Molybdenum (Mo) is a transition element present in relatively small amounts in the environment, with 2.4 mg kg−1 in the lithosphere and 0.2–36 mg kg−1 in soils (Broadley et al., 2012). Despite its low abundance in the environment, it is an essential micronutrient for almost all living organisms except for certain strains of thermophilic anaerobes (archaea and bacteria that grow optimally at temperatures of 50 °C or higher)—which require tungsten instead of Mo for survival (Hille, 2002; Bevers et al., 2009). Inorganic Mo itself is biologically inactive and must be incorporated into metal cofactors by complex biosynthetic machinery, serving as the active site of molybdenum-requiring enzymes (molybdoenzymes). There are two types of Mo-related cofactors in nature, namely a pterin-based molybdenum cofactor (Moco) and an iron–sulfur-cluster-based iron–molybdenum cofactor (FeMoco) (Schwarz and Mendel, 2006; Schwarz et al., 2009). Moco is the ubiquitous form of cofactor found in most molybdoenzymes, while FeMoco is unique to bacterial nitrogenase (Schwarz et al., 2009). Molybdoenzymes exist in nearly all living organisms except for Saccharomyces and thermophilic anaerobes (Zhang and Gladyshev, 2008). In plants, there are five types of molybdoenzymes, including nitrate reductase, aldehyde oxidase, xanthine dehydrogenase, sulfite oxidase, and amidoxime reducing component (Schwarz and Mendel, 2006; Tejada-Jimenez et al., 2013). These enzymes participate in crucial biological processes such as nitrate assimilation, phytohormone biosynthesis, purine metabolism, and sulfite detoxification. Therefore, Mo is essential for plant growth and development.
As a transition element, Mo exists in several oxidation states ranging from zero to VI. In aqueous solution with a pH >4.3, Mo exists predominantly in its highest oxidized form, the molybdate oxyanion (MoO42−), which is thought to be the main form taken up by plant roots. When pH is lower than 4.3, molybdate is protonated to HMoO4− or MoO3(H2O)3, which is likely not bioavailable to plants (Broadley et al., 2012). Furthermore, in acid soils, MoO42− is strongly adsorbed by Fe oxyhydroxide and the adsorption of molybdate increases with decreasing soil pH (Marschner and Rengel, 2012). Hence, the bioavailability of Mo is much lower in acid soils, where Mo deficiency in plants often occurs. Plants deficient in Mo usually develop ‘whiptail’ phenotypes, including mottled lesions on the leaves, rolling of leaves and wilting of leaf edges (Arnon and Stout, 1939). In response to low Mo supply, plants have evolved mechanisms to regulate the uptake, transport, and storage of Mo to adapt to the Mo-deficient environment.
In this review, we summarize current understanding of molybdate uptake and transport and the Mo homeostasis network in plants. We also discuss the potential role of such a network in the regulation of plant adaptation to their local environment. We argue that Mo is not only an essential element for plant growth and development, but may also play a key role in the adaptation to adverse environments.
Uptake and transport of molybdenum in bacteria
Living organisms that require Mo to synthesize molybdoenzymes take up molybdate from the external environment through an energy-dependent Mo uptake process. An ABC-type transport system, which is encoded by modABC genes, was first identified as the high-affinity Mo transport system in bacteria. This Mo transport system (ModAB2C2) is composed of a monomer, ModA, a homodimer, ModB2, and a homodimer, ModC2, in which ModA functions as a periplasmic molybdate-binding protein, ModB acts as a membrane channel protein, and ModC is an energy-transducing ATPase protein (Fig. 1A) (Grunden and Shanmugam, 1997; Hollenstein et al., 2007). The heteropolymer ModAB2C2 transport system orchestrates the high-affinity molybdate uptake in bacteria. Plant genomes encode a large number of ABC transporters, for example at least 133 in rice and 130 in Arabidopsis (Verrier et al., 2008). However, none of the ABC transporters tested so far is involved in Mo-specific uptake in plants.
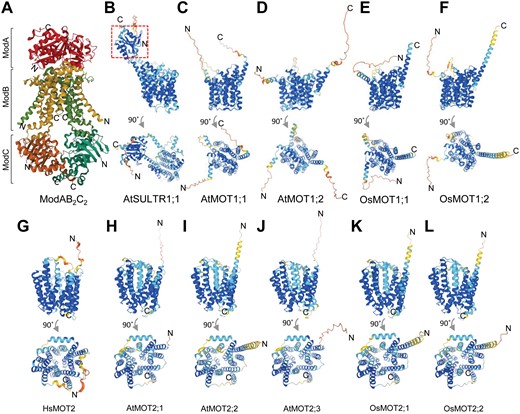
Three-dimensional (3D) structures of molybdate transporters in Archaeoglobus fulgidus, Arabidopsis, rice (Oryza sativa), and human. (A) Structures of ABC-type transport system in Archaeoglobus fulgidus. The monomer ModA, homodimer ModB, and homodimer ModC are shown in different colors. The structure was retrieved from UniProt (www.uniprot.org) with accession number O30142 (Hollenstein et al., 2007). (B–K) Predicted 3D structures of Arabidopsis sulfate transporter AtSULTR1;1 (B) and molybdate transporter family 1 (C–F) and family 2 (G–L) in Arabidopsis, rice, and human. The 3D structures were retrieved from AlphaFold Protein Structure Database (www.alphafold.ebi.ac.uk) with the accession numbers: AtSULTR1;1, Q9SAY1 (B); AtMOT1;1, Q9SL95 (C); AtMOT1;2, Q0WP36 (D); OsMOT1;1, Q6Z1Z0 (E); OsMOT1;2, Q9ASA0 (F); HsMOT2, Q6N075 (G); AtMOT2;1, Q9C5R0 (H); AtMOT2;2, Q6NLR2 (I); AtMOT2;3, Q9CA11 (J); OsMOT2;1, Q9CA11 (K); OsMOT2;2, Q6NLR2 (L). The red dash box in (B) shows the STAS domain of AtSULTR1;1. Different colors in the structures of (B–L) indicate the model confidence defined by a per-residue confidence score (pLDDT) between 0 and 100. Dark blue: very high (pLDDT>90); sky blue: confident (90>pLDDT>70); yellow: low (70>pLDDT>50); orange: very low (pLDDT<50). The structures in (B–L) are shown from the side view (top panel) or the top view (bottom panel).
Non-specific molybdate uptake pathway in plants
There are at least three molybdatate transport pathways in plants: a non-specific molybdate uptake pathway and molybdate transporter MOT1- and MOT2-mediated molybdate transport pathways. Molybdate is the main Mo form taken up by plant roots from soils. Given that molybdate has similar biochemical properties to sulfate, molybdate can be taken up non-specifically by the sulfate transport system. For example, the high-affinity sulfate transporter SHST1 in Stylosanthes hamata is able to mediate molybdate uptake when expressed in yeast cells (Fitzpatrick et al., 2008). Such non-specific molybdate uptake by sulfate transporters is largely dependent on the sulfur status in soils. Under S deficiency, molybdate uptake is strongly increased in wheat and oilseed rape (Shinmachi et al., 2010; Maillard et al., 2016). The enhancement of molybdate uptake in sulfate-limited conditions is due to the activation of sulfate transporter genes and less competition of sulfate with molybdate for sulfate transporters. The non-specific uptake of molybdate may be also mediated by the phosphate transport system as phosphorus deficiency enhances the uptake of molybdate in tomato plants (Heuwinkel et al., 1992). However, whether a phosphate transporter is responsible for the uptake of molybdate, and if so which, is not clear.
MOT1 transport pathway
In addition to the non-specific uptake pathway, two molybdate-specific transporter families, MOT1 and MOT2, have been identified in eukaryotes. The MOT1 transporter gene is present only in the genomes of algae, moss, and higher plant, and not in animals (Fig. 2A). The first eukaryotic high-affinity molybdate transporter, CrMOT1, was identified in the green alga Chlamydomonas reinhardtii (Tejada-Jimenez et al., 2007). Soon after that, two research groups independently identified the high-affinity molybdate transporter AtMOT1;1 in Arabidopsis, which shares high sequence identity to CrMOT1 (Tomatsu et al., 2007; Baxter et al., 2008). Both CrMOT1 and AtMOT1;1 possess high-affinity molybdate transport activity, with Km values of 7 nM for the former and 20 nM for the later (Tejada-Jimenez et al., 2007; Tomatsu et al., 2007). Knock-down of CrMOT1 by an antisense RNA strategy strongly inhibited the molybdate transport activity and decreased the activity of the molybdoenzyme nitrate reductase in C. reinhardtii (Tejada-Jimenez et al., 2007). Similarly, Arabidopsis AtMOT1;1 knock-out mutant or week allele accessions accumulated less Mo and displayed Mo deficiency symptoms when grown under limited Mo supply conditions, suggesting an essential role of AtMOT1;1 in the uptake of molybdate from soil (Tomatsu et al., 2007; Baxter et al., 2008). Recently, OsMOT1;1 has been identified in rice through genome-wide association studies (GWAS) or QTL mapping analysis based on rice grain Mo concentration (Yang et al., 2018; Huang et al., 2019; Wang et al., 2020). OsMOT1;1 mediated the molybdate uptake when heterogeneously expressed in yeast cells, and the knock-out mutant of OsMOT1;1 accumulated lower levels of Mo in both shoots and roots (Huang et al., 2019).
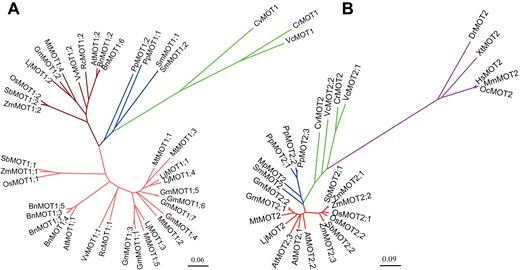
Phylogenetic analysis of MOT1 and MOT2 proteins in different species. The MOT1 (A) or MOT2 (B) proteins in different species are highlighted with different cluster colors: algae, green; moss, blue; higher plants, red; animals, purple. The dark red and light red in (A) represents two groups of MOT1 in higher plants. The phylogenetic tree was constructed by MEGA7 with the neighbor-joining method and modified slightly by FigTree software with the following accession numbers. MOT1 family: Arabidopsis: AtMOT1;1, At2G25680; AtMOT1;2, At1G80310; Chlamydomonas reinhardtii: CrMOT1, XP_001698673.2; Lotus japonicus: LjMOT1;1, Lj3g3v0821100.1; LjMOT1;2, Lj3g3v2236900.1; LjMOT1;3, Lj1g3v1932460.1; LjMOT1;4, Lj3g3v0819900.1; Medicago truncatula: MtMOT1;1, XP_013465770.1; MtMOT1;2, XP_013465776.1; MtMOT1;3, XP_013460259.1; MtMOT1;4, XP_013454709.1; MtMOT1;5, XP_003603486.1; Oryza sativa: OsMOT1;1, LOC_Os08g01120; OsMOT1;2, LOC_Os01g45830; Zea mays: ZmMOT1;1, XP_008664989.1; ZmMOT1;2, NP_001150854.1; Glycine max: GmMOT1;1, Glyma06g07780.1; GmMOT1;2, Glyma08g22300.1; GmMOT1;3, Glyma04g07690.1; GmMOT1;4, Glyma17g29950.1; GmMOT1;5, Glyma14g16610.2; GmMOT1;6, Glyma17g29830.2; GmMOT1;7, Glyma17g29850.2; Ricinus communis: RcMOT1;1, XP_002516602.2; RcMOT1;2, XP_002517550.1; Sorghum bicolor: SbMOT1;1, XP_021307562.1; SbMOT1;2, XP_021310787.1; Brassica napus: BnMOT1;1, XP_013709878.1; BnMOT1;2, XP_013743052.1; BnMOT1;3, XP_013667816.1; BnMOT1;4, CAF2049375.1; BnMOT1;5, XP_013748013.1; BnMOT1;6, CAF2209111.1; Vitis vinifera: VvMOT1;1, XP_002281989.2; VvMOT1;2, XP_002285217.1; Selaginella moellendorffi: SmMOT1;1, EFJ21108.1; SmMOT1;2, EFJ11971.1; Physcomitrium patens: PpMOT1;1, PNR60186.1; PpMOT1;2, PNR34408.1; Chlorella variabilis: CvMOT1, EFN52559.1; Volvox carteri: VcMOT1, EFJ47751.1. MOT2 family: Arabidopsis: AtMOT2;1, At4G27720; AtMOT2;2, At1G64650; AtMOT2;3, At3G49310; Chlamydomonas reinhardtii: CrMOT2, XP_001693567.2; Lotus japonicus: LjMOT2, Lj1g3v103017.1; Medicago truncatula: MtMOT2, XP_003602305.1; Oryza sativa: OsMOT2;1, LOC_Os03g02380; OsMOT2;2, LOC_Os10g37520; Zea mays: ZmMOT2;1, ONL92907.1; ZmMOT2;2, NP_001149961.1; ZmMOT2;3, XP_008672796.1; Glycine max: GmMOT2;1, Glyma04g232500; GmMOT2;2, Glyma06g132500; Sorghum bicolor: SbMOT2;1, XP_021303322.1; SbMOT2;2, XP_002466934.1; Homo sapiens: HsMOT2, BAC11137.1; Selaginella moellendorffi: SmMOT2, EFJ11977.1; Micromonas pusilla: MpMOT2, XP_003062454.1; Physcomitrium patens: PpMOT2;1, PNR33270.1; PpMOT2;2, PNR49445.1; PpMOT2;3, PNR28044.1; Chlorella variabilis: CvMOT2, XP_005843441.1; Volvox carteri: VcMOT2;1, XP_002948878.1; VcMOT2;2, XP_002949049.1; Danio rerio: DrMOT2, NP_001002713.1; Xenopus tropicalis: XtMOT2, NP_001015939.1; Mus musculus: MmMOT2, NP_598861.1; Oryctolagus cuniculus: OcMOT2, XP_008254730.1.
MOT1;1 proteins show sequence similarity to sulfate transporters and were previously clustered in group V of the sulfate transporter superfamily. However, neither AtMOT1;1 nor OsMOT1;1 exhibits sulfate transport activity when expressed in yeast cells (Tomatsu et al., 2007; Huang et al., 2019). This is likely due to the lack of a STAS (sulfate transporters and anti-sigma factor antagonist) domain at the C-terminus of MOT1;1 (Fig. 1B–F), which is essential for controlling the transport activity for sulfate. Furthermore, the predicted three-dimensional structures of MOT1 family proteins and Arabidopsis high-affinity sulfate transporter AtSULTR1;1 suggest that they all have 13 transmembrane domains with the last two domains at the C-terminus not fully integrated into the membrane (Fig. 1B–F). However, the arrangement of the transmembrane domains seems very similar among MOT1 proteins but is distinct from AtSULTR1;1, which may also explain their different transport substrates.
Legume genomes generally encode more copies of MOT1, which may be due to the relatively large amounts of Mo required for the biosynthesis of FeMoco for nitrogenase during symbiotic nitrogen fixation. Among the five MOT1 transporters in M. truncatula, MtMOT1;2 and MtMOT1;3 have been confirmed to have molybdate transport activities (Tejada-Jimenez et al., 2017; Gil-Diez et al., 2019). MtMOT1;2 locates in the endodermal cells in roots and nodules and functions to transport molybdate into the endodermal cells for further delivery to the nodules (Gil-Diez et al., 2019). Knock-out of MtMOT1;2 leads to lower levels of Mo in the nodules. However, mutation of MtMOT1;3 results in more Mo accumulation in the mutant nodules even though the nitrogenase activity is almost completely lost, suggesting MtMOT1;3 is not responsible for direct molybdate delivery to the nodules but may mediate the efflux of Mo into the symbiosome space (Tejada-Jimenez et al., 2017). In Lotus japonicas, LjMOT1 (corresponding to LjMOT1;2 in Fig. 2A) is responsible for molybdate uptake from soil but may not be involved in the delivery of Mo to the nodules as the knock-out mutant shows similar nitrogen fixation activity to the wild-type (Gao et al., 2016; Duan et al., 2017). The functions of other legume MOT1 family proteins, especially the seven members of MOT1 proteins in soybean, remain to be characterized.
The localization of AtMOT1;2 and OsMOT1;2 has been clearly shown to be at the tonoplast (Gasber et al., 2011; Ishikawa et al., 2021). However, the subcellular localization of AtMOT1;1 is still controversial as two different localizations of this protein, plasma membrane and mitochondria, have been reported. Based on transient expression in tobacco BY-2 cells with green fluorescent protein (GFP) fused to the N-terminus, AtMOT1;1 was shown to localize to the plasma membrane and the endomembrane (Tomatsu et al., 2007). In contrast, transient or stable expression of AtMOT1;1 fused with GFP at the C-terminus showed that the protein is localized to the mitochondria in Arabidopsis (Baxter et al., 2008). The different localizations of AtMOT1;1 in these two studies might be due to the different sites of GFP fusion. In silico analysis suggested the existence of a mitochondrial localization signal at the N-terminus of AtMOT1;1 (Baxter et al., 2008). Therefore, fusion of GFP to the N-terminus of AtMOT1;1 might block the mitochondrial target peptide and likely lead to the mislocation of GFP–AtMOT1;1 (Baxter et al., 2008). However, LjMOT1 with GFP fusion at the C-terminus was found to be localized to the plasma membrane when transiently expressed in tobacco leaves (Gao et al., 2016), suggesting the GFP fusion site might not be the critical factor determining the protein localization. Similarly, both MtMOT1;2–GFP and MtMOT1;3–GFP were also localized to the plasma membrane when transiently expressed in tobacco leaves, further confirmed by gold-immunohistochemistry (Tejada-Jimenez et al., 2017; Gil-Diez et al., 2019). By transient expression of OsMOT1;1–GFP in rice protoplast, OsMOT1;1 was found to localize to the plasma membrane as well as there being a stronger GFP signal in the cytosol (Ishikawa et al., 2021).
None of the above studies demonstrated that the MOT1 protein localized to either plasma membrane or mitochondria is functional. Recently, Huang et al. (2019) showed that OsMOT1;1–GFP localizes to mitochondria in both stable transgenic rice and Arabidopsis, and further confirmed that the mitochondrially localized OsMOT1;1–GFP is functional in both rice and Arabidopsis. This is supported by the fact that overexpression of OsMOT1;1-GFP strongly enhanced the accumulation of Mo in both rice and Arabidopsis, and expression of OsMOT1;1-GFP in the atmot1;1 mutant under the control of AtMOT1;1 native promoter completely rescued the low Mo phenotype of atmot1;1 (Huang et al., 2019). Although the plasma membrane localization of MOT1;1 proteins is consistent with its function in molybdate uptake, further studies are required to validate the localization by testing their functionalities in stable transgenic lines. The mitochondrial localization of OsMOT1;1 suggests that OsMOT1;1 may transport Mo from cytosol into mitochondria. Knock-out of OsMOT1;1 results in less Mo transported into mitochondria and more Mo accumulation in cytosol, which may inhibit the uptake of Mo and lead to the low Mo phenotype of the osmot1;1 mutant (Huang et al., 2019).
While there is only one member of MOT1 in C. reinhardtii, up to seven MOT1 are found in higher plants (Fig. 2A). For example, there are two copies of MOT1 in Arabidopsis, rice, and maize, and more than four copies in the legume plants Lotus japonicas, Medicago truncatula, and soybean (Glycine max) (Fig. 2A). The MOT1 family in higher plants could be grouped into two clusters. In contrast to more than one copy of MOT1 in the MOT1;1 cluster, there is only one copy of MOT1 in the MOT1;2 cluster for diploid plants (Fig. 2A). The tonoplast-localized Arabidopsis AtMOT1;2 (previously misnamed MOT2) may function as a vacuolar molybdate exporter and regulate the distribution of Mo in different tissues and organs, although its molybdate transport activity was not confirmed (Gasber et al., 2011). Very recently, OsMOT1;2 was also shown to be involved in inter-organ Mo distribution in rice (Ishikawa et al., 2021). Knock-out of OsMOT1;2 resulted in decreased accumulation of Mo in shoots and grains but increased accumulation in roots. However, the molybdate transport activity of OsMOT1;2 was not detected in yeast cells (Ishikawa et al., 2021).
MOT2 transport pathway
Unlike MOT1, which only exists in plants and algae, MOT2 is present in algae, plants, and animals including human (Fig. 2B). MOT2 proteins belong to the major facilitator superfamily with no sequence similarity to MOT1. There is only one copy of MOT2 in C. reinhardtii and human while more than two copies are found in plants, for example two and three copies in rice and Arabidopsis, respectively (Fig. 2B). Three-dimensional structure prediction suggests that MOT2 proteins in human, rice, and Arabidopsis all have 13 transmembrane domains (Fig. 1G–L). The arrangements of transmembrane domains of MOT2s in these three species are very similar but are distinct from that of MOT1 proteins (Fig. 1B–L).
The first MOT2 family protein, CrMOT2, was identified in C. reinhardtii. Similar to CrMOT1, CrMOT2 is also a high-affinity molybdate transporter with a Km value of 550 nM (Tejada-Jimenez et al., 2011), which is higher than that of CrMOT1 (7 nM) and AtMOT1;1 (20 nM) (Tejada-Jimenez et al., 2007; Tomatsu et al., 2007). Transport competition studies revealed that CrMOT2 is a specific molybdate transporter with no transport activities for sulfate, similar to that of CrMOT1. In contrast to CrMOT1, whose expression remains unchanged, the transcription of CrMOT2 is induced by molybdate deficiency. However, CrMOT1 but not CrMOT2 is activated by the presence of nitrate (Tejada-Jimenez et al., 2007; Tomatsu et al., 2007). Therefore, CrMOT1 and CrMOT2 may play distinct roles in controlling Mo homeostasis in C. reinhardtii. CrMOT1 is involved in molybdate transport connected to nitrate reductase activities, whereas CrMOT2 plays essential roles in molybdate uptake under Mo-limited condition.
Heterologous expression of human HsMOT2 in yeast cells reveals its capacity for transport of molybdate with similar Km value (546 nM) to that of CrMOT2 (Tejada-Jimenez et al., 2011). The molybdate transport activity of HsMOT2 is also not inhibited by external supply of sulfate, suggesting that it is a molybdate-specific transporter. The molybdate transport activity of MOT2 in higher plants has not been investigated. Recently, OsMOT2;1, also named OsCd1, was found to be involved in root cadmium (Cd) uptake and Cd accumulation in rice grain through GWAS on grain Cd concentration (Yan et al., 2019). Expression of OsMOT2;1/OsCd1 in yeast leads to targeting of the protein to the plasma membrane and increases the sensitivity to Cd stress with more Cd accumulation in the cells, suggesting a role in Cd uptake. Knockout of OsMOT2;1/OsCd1 results in decreased Cd accumulation in shoots, roots, and grains of rice plants. The accumulation of Mn, but not Zn or Fe, was also decreased in shoots and roots of the oscd1 knockout mutant (Yan et al., 2019). Whether OsMOT2;1/OsCd1 transports Mo was not investigated. Given that the chemical properties of MoO42− are completely different from Cd2+, it is unlikely that OsMOT2;1/OsCd1 has molybdate transport activity.
The second MOT2 member in rice, OsMOT2;2, has also been characterized previously. OsMOT2;2, also named ABNORMAL SHOOT IN YOUTH (ASY), plays an important role in the regulation of early shoot development in rice (Hibara et al., 2013). The asy mutant, with mutation in an mRNA splice site, showed abnormal phenotypes during the early vegetative growth stage, including leaf abnormalities and even seedling lethality. However, the Mo concentration in the grains of the asy mutant is similar to the wild-type (Hibara et al., 2013), suggesting that OsMOT2;2/ASY may also not participate in molybdate transport. The mutants of both OsMOT2;1 and OsMOT2;2 show growth defects, indicating they may play more general roles in plant development. There are three copies of AtMOT2 in Arabidopsis while their molybdate transport activities and functions have not been investigated. Further studies are required to confirm whether MOT2 in higher plants has molybdate transport activity.
Mo transport and local adaptation
Plants grow in diverse environments including the highly heterogeneous soil system with variable levels of essential mineral nutrients. As sessile organisms, they have developed sophisticated mechanisms to maintain optimized level of mineral nutrients and trace elements (also known as the ionome) in cells in order to adapt to the spatially and temporally heterogeneous soil environments. Taking advantage of ionomic approaches combined with genetic analysis such as QTL mapping and GWAS, several ionomic alleles with adaptive variation to particular soil environments have been identified (Huang and Salt, 2016). AtMOT1;1 is one such ionomic allele that has a potential role in adaptation to local environments.
By using a QTL mapping approach, AtMOT1;1 was identified as the causal gene for the reduced shoot Mo concentration in Arabidopsis accession Landsberg erecta (Ler) (Tomatsu et al., 2007; Baxter et al., 2008). A 53-bp deletion lacking the TATA box in the promoter region of AtMOT1;1 leading to reduced expression level of AtMOT1;1 is responsible for the low Mo concentration in shoots of Ler. Further GWAS based on the variance heterogeneity of leaf Mo concentrations in 283 Arabidopsis accessions identified a 330-bp-long duplication located inside a transposable element in front of AtMOT1;1 (Shen et al., 2012; Forsberg et al., 2015). This duplicated sequence also contributes to the variation of shoot Mo concentrations across populations. In contrast to the reduced expression of AtMOT1;1 by the 53-bp deletion, the 330-bp duplication increases the expression of AtMOT1;1 and results in higher accumulation of Mo in shoots (Forsberg et al., 2015). The variable function of AtMOT1;1 is not solely due to sequence polymorphisms in the promoter region. A single nucleotide polymorphism in the coding region of AtMOT1;1 resulting in an amino acid change has been shown to disrupt the function of AtMOT1;1 in the Arabidopsis accession Sha (Poormohammad Kiani et al., 2012). The accessions containing a Ler-like or Sha-like hypofunctional AtMOT1;1 allele display defective growth under acidic soil conditions in which Mo is less bioavailable (Poormohammad Kiani et al., 2012). The AtMOT1;1 knock-out mutant is also more sensitive to low pH compared with the wild-type (Tomatsu et al., 2007). Furthermore, in wild Arabidopsis populations from diverse regions, accessions with a defective AtMOT1;1sha allele grow on soils with high water-extractable Mo while the accessions with a strong allele are generally found in the regions with relatively low Mo in soils (Poormohammad Kiani et al., 2012). This suggests that the distribution of different AtMOT1;1 alleles is associated with the Mo level in soils. The functional AtMOT1;1 allele thus plays adaptive role in accessions grown in low Mo soils in order to take up sufficient Mo. Therefore, the allelic heterogeneity at the AtMOT1;1 locus is involved in adaptation to variable Mo levels in soils.
Recently, Busoms et al. (2021) showed that the AtMOT1;1 allele with the 53-bp deletion in the promoter region plays a role in local adaptation to coastal saline habitats as this allele occurs primarily in coastal environments. Previous GWAS based on the means of leaf Mo concentrations revealed that the variation of leaf Mo concentration is not only associated with multiple alleles of AtMOT1;1 (the 53-bp deletion and the 330-bp-long duplication at the AtMOT1;1 locus as mentioned above), but also with multiple loci (AtMOT1;1, a single nucleotide polymorphism (SNP) approximately 25 kb downstream of AtMOT1;1, and a second SNP ~600 kb upstream of AtMOT1;1; Forsberg et al., 2015). Although the potential causal gene for the first associated SNP ~25 kb downstream of AtMOT1;1 is not clear, Copper Transporter 6 (COPT6) was shown to be the candidate gene for the association at the associated SNP approximately 600 kb upstream of AtMOT1;1 by using a T-DNA knockout line. This suggests the existence of a genetic link between Mo and Cu homeostasis. Such crosstalk between Mo and Cu is consistent with the fact that both Mo and Cu are required for the biosynthesis of Moco. In the last step of Moco biosynthesis, Mo is inserted into molybdopterin by replacing Cu to form Moco, which is finally allocated to molybdoenzyme (Schwarz and Mendel, 2006; Mendel, 2007). In Arabidopsis accessions with the 53-bp deletion AtMOT1;1 allele causing lower expression, the expression of COPT6 is elevated perhaps to take up sufficient Cu from the coastal soils in which the Cu concentration is generally low (Busoms et al., 2015, 2021). Furthermore, the concentrations of abscisic acid (ABA), an important abiotic stress-responsive hormone, is also increased in these accessions with the AtMOT1;1 weak allele particularly under salt stress condition (Busoms et al., 2021). Therefore, the AtMOT1;1 weak allele plays a role in local adaptation to coastal saline habitats by enhancing the expression of COPT6 and the biosynthesis of Moco and ABA. However, it is still unclear how the interruption of AtMOT1;1 promotes Cu uptake and Moco and ABA biosynthesis.
Given that the mitochondrial localization of OsMOT1;1 is functional, as expression of OsMOT1;1 under the control of the AtMOT1;1 native promoter completely rescued the low Mo phenotype of atmot1;1 (Huang et al., 2019), AtMOT1;1 is more likely localized to mitochondria. The mitochondrial localization of AtMOT1;1 suggests that AtMOT1;1 may function as a molybdate transporter mediating the transport of Mo from cytosol into mitochondria (Fig. 3). The weak allele of AtMOT1;1, for example the allele with 53-bp deletion resulting in lower expression level, would lead to less Mo transport into mitochondria and more Mo accumulation in the cytosol. On one hand, the elevation of Mo level in cytosol may suppresses the uptake of Mo through an unknown signal transduction pathway to avoid overaccumulation of Mo in cells; this is consistent with the low Mo phenotype of Arabidopsis accessions with AtMOT1;1 weak alleles (Tomatsu et al., 2007; Baxter et al., 2008). On the other hand, the increasing level of Mo in cytosol may also enhance the biosynthesis of Moco. This is supported by the increasing expression levels of CNX1 and CNX6, two genes encoding enzymes involved in Moco biosynthesis, in the AtMOT1;1 weak allele accessions (Busoms et al., 2021). Given Cu is also required for the biosynthesis of Moco (Schwarz and Mendel, 2006; Mendel, 2007), the enhancement of Moco biosynthesis would require more Cu, perhaps by activation of the plasma membrane-localized copper transporter COPT6 (Fig. 3).
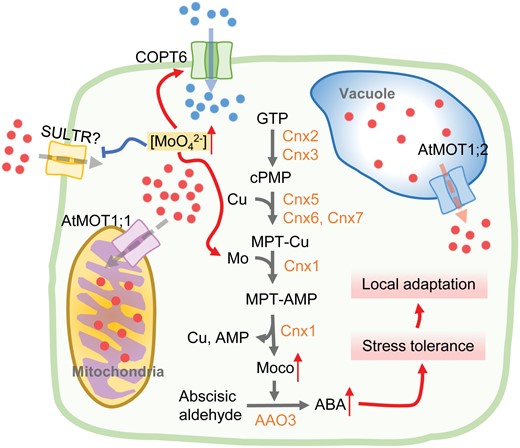
Proposed model of potential roles of AtMOT1;1 in local adaptation to coastal saline habitats. The mitochondrial localization of AtMOT1;1 functioning as a molybdate transporter mediates the efflux of Mo (red circles in the figure) into mitochondria. The weak allele of AtMOT1;1 with lower expression level results in less Mo transported into mitochondria and more Mo accumulation in the cytosol. The elevation of Mo level in cytosol may enhance the biosynthesis of Moco, which acts as a cofactor of aldehyde oxidase AAO3 and further promotes oxidation of abscisic aldehyde to produce bioactive ABA. The increasing level of abiotic stress-responsive hormone enables the Arabidopsis accessions with weak AtMOT1;1 to be more tolerant to salinity stress and more adaptive to coastal saline habitats. As Cu (blue circles) is also required for the biosynthesis of Moco, the enhancement of Moco biosynthesis would require more Cu perhaps by activation of COPT6 in plasma membrane. AMP, adenylated molybdopterin; Cnx, cofactor for nitrate reductase and xanthine dehydrogenase; cPMP, cyclic pyranopterin monophosphate; GTP, guanosine-5-triphosphate; MPT, molybdopterin; SULTR, sulfate transporter.
The Arabidopsis molybdoenzyme aldehyde oxidase AAO3 (also known as ABA3), which requires Moco for full activity, catalyses the oxidation of abscisic aldehyde to produce bioactive ABA (Seo et al., 2000a, b). The enhancement of Moco biosynthesis may promote the biosynthesis of ABA, which plays key roles in various abiotic stress tolerances including salinity stress. Therefore, the Arabidopsis accession with AtMOT1;1 weak allele is more adaptive to coastal saline habitats (Fig. 3).
Similar to Arabidopsis, the natural variation in grain Mo concentration in rice is attributed to a variable expression level of OsMOT1;1, which is caused by the variation in the promoter sequence (Yang et al., 2018; Huang et al., 2019). Knock-out of OsMOT1;1 leads to hypersensitivity to low pH growth medium with less bioavailable Mo (Huang et al., 2019). However, the distribution of different OsMOT1;1 alleles in rice germplasm and their correlations to the Mo level in soils as well as local adaptation are not clear. Recently, two rice preharvest sprouting mutants were identified with mutations in Moco biosynthesis enzymes OsCNX6 and OsCNX2 (Liu et al., 2019). ABA concentration was decreased in these mutants due to low activities of the Moco-dependent enzyme aldehyde oxidase, which is necessary for ABA biosynthesis. The impairment in ABA biosynthesis resulted in the lack of seed dormancy and preharvest sprouting (Liu et al., 2019). Seed dormancy is a key trait to ensure plants germinate under favorable environments, suggesting that genes involved in Moco biosynthesis in rice might also be related to local adaptation.
Conclusions and future perspectives
As Mo is a trace element essential for most living beings, its level in cells and organisms must be tightly controlled. Prokaryotes such as bacteria use the ABC-type transport system to facilitate high-affinity molybdate uptake consisting of three different components. Such a molybdate transport complex is likely not present in eukaryotes. In contrast, eukaryotes possess the MOT1- and/or MOT2-mediated molybdate transport system. The MOT1 transporters can be found in algae, moss, and higher plants, but not in animals. Given MOT1 belongs to group V of the sulfate transporter family and animals lack the sulfate uptake and assimilation system, it is likely that MOT1 genes have been lost together with sulfate transporter genes in animals during evolution. In animals, molybdate transport is mediated by the MOT2 transporting system. Although MOT2 genes exist in the genomes of higher plants, the molybdate transport activities have not been confirmed. The knock-out mutants of rice OsMOT2;1 and OsMOT2;2 do not display Mo-deficient phenotypes (Hibara et al., 2013; Yan et al., 2019), suggesting a lack of molybdate transport activities of these two transporters. Therefore, it is likely that the molybdate transport in higher plants is mediated by MOT1. Interestingly, both MOT1 and MOT2 function as molybdate transporters with distinct roles in maintenance of Mo homeostasis in the unicellular alga C. reinhardtii (Tejada-Jimenez et al., 2007, 2011). The underlying mechanisms and evolutionary significance of the diverse molybdate transport systems in different species are not clear and require further study.
In addition to Mo being an essential micronutrient for plant growth and development, emerging evidence suggests that it may also play a key role in local adaptation to adverse environments. This is evidenced by the critical role of the weak allele of AtMOT1;1 in the adaptation of Arabidopsis to coastal saline habitats (Busoms et al., 2021). Future studies are required to fully reveal the underlying adaptive mechanisms mediated by AtMOT1;1 to saline environments. Whether and how other molybadate transport processes as well as Mo metabolism play a role in local adaptation is worth future investigation.
Conflict of interest
The authors declare no conflict of interest.
Funding
This work was supported by the Natural Science Foundation of China (31970271, 31772382) and the Natural Science Foundation of Jiangsu Province for Distinguished Young Scholars (BK20180023).
References
Comments