-
PDF
- Split View
-
Views
-
Cite
Cite
Tingfa Dong, Baoli Duan, Helena Korpelainen, Ülo Niinemets, Chunyang Li, Asymmetric pruning reveals how organ connectivity alters the functional balance between leaves and roots of Chinese fir, Journal of Experimental Botany, Volume 70, Issue 6, 1 March 2019, Pages 1941–1953, https://doi.org/10.1093/jxb/erz013
- Share Icon Share
Abstract
The functional balance between leaves and roots is believed to be mediated by the specific location of shoots and roots, i.e. differences in transport distances and degrees of organ connectivity. However, it remains unknown whether the adaptive responses of trees to biomass removal depend on the relative orientation of leaf and root pruning. Here, we applied five pruning treatments to saplings of Cunninghamia lanceolata (Chinese fir) under field and glasshouse conditions, namely no pruning (control), half of lateral branches pruned, half of lateral roots pruned, half of the branches and roots pruned on the same side of the plant, and half of the branches and roots pruned on opposite sides of the plant. The effects of pruning on the growth, carbon storage and allocation, and physiology of leaves and fine roots on the same and opposite sides of the plant were investigated. Compared with the effect of root-pruning on leaves, fine roots were more limited by carbon availability and their physiological activity was more strongly reduced by shoot pruning, especially when branches on the same side of the plant were removed. Pruning of branches and roots on the opposite side of the plant resulted in the lowest carbon assimilation rates and growth among all treatments. The results of a stable-isotope labeling indicated that less C was distributed to fine roots from the leaves on the opposite side of the plant compared to those on the same side, but N allocation from roots to leaves depended less on the relative root and leaf orientation. The results collectively indicate that the functional responses of C. lanceolata to pruning are not only determined by the source–sink balance model but are also related to interactions between leaves and fine roots. We argue that the connectivity among lateral branches and roots depends on their relative orientation, which is therefore critical for the functional balance between leaves and fine roots.
Introduction
Balanced growth between shoots and roots that optimizes the carbon budget is crucial for the survival and maximization of competitive ability and reproduction of plants. However, heterogeneity among branches within a crown or root system, as induced by light, soil water, nutrients, or herbivores, is a common phenomenon in natural plant communities (Niinemets, 2007; Kawamura, 2010; Zhang et al., 2019). On the other hand, in some planted ecosystems, asymmetric disturbance among the organs is introduced through artificial manipulation (e.g. thinning) to reduce competition among neighboring individuals, thereby enhancing the amount of harvestable biomass (Quentin et al., 2012; Dong et al., 2016). Such imbalanced disturbances among organs usually change the growth of the whole plant, its defense, and its reproduction, since different organs do not grow independently (Arnold et al., 2004; De Kroon et al., 2009).
Plant responses to disturbances depend on the source–sink relationships among organs (Galiano et al., 2011; Wiley et al., 2017). These relationships are closely correlated with the strengths of sources (the ability of source organs to export carbohydrates) and sinks (the ability of sink organs to import and use carbohydrates), and hence imbalanced supply and use of carbohydrates can change the source–sink balance (Paul and Foyer, 2001; Arnold et al., 2004; Pinkard et al., 2011; Savage et al., 2016). Manipulations of source and sink organs (e.g. defoliation and root pruning) are often applied to investigate the source–sink balance of plants, as pruning alters the availability of carbohydrates and changes plant growth and allocation (Quentin et al., 2012; Wiley et al., 2017). Studies on partial pruning of roots or shoots of trees have found that modifications in the water and nutrient status, non-structural carbohydrate content, and gas exchange characteristics result in changes in growth and biomass allocation patterns (Galiano et al., 2011; Quentin et al., 2012; Wiley et al., 2013; Dong et al., 2016; Schmid et al., 2017). Generally, severe shoot pruning will decrease carbohydrate reserves in roots together with root growth and metabolic activity (e.g. water and nutrient uptake), increase root mortality (Snyder and Williams, 2003; Willaume and Pagès, 2006), and ultimately will lead to growth reduction of the whole tree, especially in evergreen species (Schmid et al., 2017). Severe root pruning, in turn, will cause a deficiency of water and nutrients, resulting in a down-regulation of photosynthetic metabolism (Dong et al., 2016). Moderate pruning of shoots or roots, however, may not affect tree growth and allocation (Wiley et al., 2013; Dong et al., 2016), as the growth of shoots and roots may be either synergistic or antagonistic depending on the extent to which leaf growth is limited by carbohydrates, water, and nutrients (Willaume and Pagès, 2006; Xu et al., 2008; Zhang et al., 2014). When a greater share of carbohydrates is required for leaf growth, mutual competition between leaves and roots for carbohydrates can lead to antagonistic responses (Willaume and Pagès, 2006). However, it is often unclear whether plant growth is limited by the source or sink, or both (Farrar and Jones, 2000; Asao and Ryan, 2015).
Plants are complex integrated systems in which organs interact via effective transport pathways for carbon, water, nutrients, and signaling molecules. Besides the properties of the sinks or sources, the whole long-distance transport system (xylem and phloem) may also influence the functional balance among sink and source organs (Minchin and Lacointe, 2005; Orians et al., 2005). The topology of vascular bundles (and hence the degree of vascular connectivity) and the distance between sources and sinks are two important characteristics of the long-distance translocation pathways between leaves and roots (Arnold et al., 2004). Because of these linkages, the properties of long-distance transport systems can affect the functional balance between organs, and thus affect plant growth (Orians et al., 2005; Nikinmaa et al., 2014). A longer pathway always causes higher hydraulic resistance in the xylem (Mäkelä and Valentine, 2006) and greater sugar concentration gradients in the phloem (Paljakka et al., 2017). In trees, differences in anatomical characteristics of the xylem or phloem between the axial and radial directions usually imply a functional difference (James et al., 2003; Domec et al., 2006), and the distance from lateral branches to the roots on the same side of the plant is generally shorter than the distance from branches to roots on opposite locations of the same node (Orians et al., 2002). Although these orientation-dependent differences in transport pathway lengths can alter the connectivity among plant organs, information of how the functional balance of trees is altered due to differences in the relative direction between branches and roots is still scarce.
Cunninghamia lanceolata (Chinese fir) is a widely planted, economically important, fast-growing evergreen conifer that occurs in warm monsoon climates in subtropical Asia, and it plays an important role in the regional carbon cycle (Liu et al., 2000). Branch and/or root pruning are common silvicultural practices used in subtropical forestry to enhance productivity and to reduce the length of cropping rotation. Previous studies have found that the leaves and roots of C. lanceolata are sensitive to changes in nutrient and water availability (Chen et al., 2015; Dong et al., 2016), and to artificial pruning (Dong et al., 2016). On the other hand, it has been demonstrated that significant compensation can occur after a new functional balance is achieved (Chen et al., 2015; Dong et al., 2016). In the present study, we compared the effects of different pruning treatments (above-ground versus belowground, the same versus opposite sides of the plant) on the growth, the morphological traits of leaves, branches, and fine roots, the non-structural carbohydrate (NSC) and nutrient contents, and the physiological properties of current-year leaves and fine roots in C. lanceolata grown in a field experiment for two seasons. These measurements were further replicated in a carbon- and nitrogen-isotope labeling experiment under greenhouse conditions. Our aims were to determine the following. (1) Whether growth and NSC contents of leaves and fine roots are different between partially pruned shoots and roots. (2) Whether the responses of lateral leaves and fine roots (e.g. morphology and physiological traits) depend on the relative location (same side versus opposite side) of the partial pruning and, if so, whether leaves or fine roots on the opposite side are more sensitive to partial pruning than those pruned on the same side. (3) Whether carbon allocation into fine roots from shoots or nitrogen allocation into leaves from roots are related to the relative location of pruning. We hypothesized that the orientation of leaf and root pruning will critically affect plant carbon balance, physiology, and growth.
Material and methods
Field experiments
Study site and materials
The study was conducted at the Hongya National Plantation Forestry Station (29°47′N, 103°18′E, 1100 m a.s.l.) in the Sichuan province, southwestern China. This area is characterized by a subtropical humid monsoon climate with warm and rainy summers and dry and cold winters. The average annual temperature is 16.6 °C and the average annual precipitation is 1436.5 mm. The soil is mountain yellow soil. The study site comprises an even-aged (5 years old at the beginning of the experiment) monoculture plantation forest of Cunninghamia lanceolata (Lamb.) Hook. with a leaf area index of ~6 m2 m−2. During the early growth phase, the crown architecture of C. lanceolata is conical (Dong et al., 2015). The root system is characterized by a robust taproot with abundant lateral roots. The space between the neighboring stems was ~2.0 m, and the lateral branches of adjacent saplings did not overlap during the study.
Experimental design
We randomly selected 100 5-year-old saplings (~2 m in height). The first pruning treatments were carried out on 22–24 July 2012. At that time, the expansion growth of current-year leaves (~4 months old) had been completed, the leaves were fully developed, and the trees were in a fast phase of height and diameter growth, whilst latewood had just started to form (Liu and Wen, 2005). Half of the lateral branches and lateral roots were pruned vertically with a sharp blade after digging a trench along the base of the trunk (0.5 m length × 0.2 m wide × 0.5 m depth). Five treatments were applied, namely no pruning (NP, control), half of lateral branches pruned (BP), half of lateral roots pruned (RP), half of the branches and roots on the same side (both branches and roots from the same azimuthal direction) pruned (SP), and half of the branches and roots on the opposite side (branches and roots from the opposite directions) pruned (OP). The orientation of pruning was randomly selected. The leaves and roots investigated are termed as NPL, BPL, RPSL (same-sided orientation with respect to pruned roots), RPOL (opposite-sided orientation with respect to pruned roots), SPL, and OPL for the leaves, and NPR, BPSR (same-sided orientation with respect to pruned branches), RPR (opposite-sided orientation with respect to pruned branches), RPR, SPR, and OPR for the roots (Fig. 1). In all cases, the plane of pruning was parallel to the stem and taproot. Each treatment was applied to 20 individuals, including four replications with five individuals in each replication. Repeated pruning treatments were carried out each month and involved the excision of new lateral branches and roots.
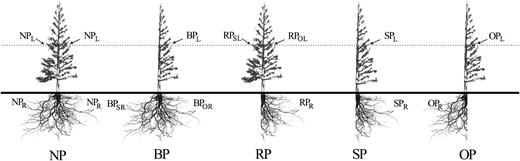
Illustration of the experimental design of the pruning treatments applied to C. lanceolata. NP, no pruning; BP, half of lateral branches pruned; RP, half of lateral roots pruned; SP, half of the lateral branches and roots pruned on the same side of the plant, i.e. the same azimuthal direction; and OP, half of the lateral branches and roots pruned on the opposite side of the plant, i.e. the opposite azimuthal direction. Leaves from the same internode in lateral branches in the mid-crown were selected for analyses. Codes for the branch and root samples taken are also shown as subscripts: R, roots; L, leaves; SR, roots on same side as branch pruning; OR, roots on opposite side to branch pruning; SL, leaves on same side as root pruning; OL, leaves on opposite side to root pruning.
Growth and morphology of foliage and fine roots
Field measurements were conducted on 22–24 July 2012 for basal stem diameter and height (the first pruning) and on 5–8 October 2012 (10 weeks after first pruning) and 26–28 September 2013 (60 weeks after first pruning) for all traits. Four randomly selected individuals per treatment were measured for stem height and basal stem diameter. The average weekly increments of basal stem diameter and height were calculated. Fine roots and current-year leaves of lateral branches of the same internode (target branches) from the mid-crown and were selected for all ecophysiological measurements.
Two target branches from each tree were harvested between 14.00–16.00 h. Current-year leaf samples were scanned and their area was estimated using the ImageJ software (https://imagej.nih.gov/ij/). The leaves and branches were then oven-dried at 70 °C to a constant mass, and the average area and dry mass per current-year leaf and the average length and dry mass per target branch were calculated.
Roots were carefully excavated from a depth of 0–30 cm. Three intact lateral roots were harvested per treatment and pooled as a single sample. The samples were carefully washed and transported on ice to the laboratory within a few hours. Fine roots (<2 mm in diameter) were separated and divided into two parts. One part was used for morphological analyses and for the measurement of respiration and non-structural carbohydrates, and the other part was used for other biochemical measurements. For the morphological analyses, the fine-root samples that were measured for respiration were also scanned to estimate their length using root system analysis software (WinRhizo, Regent Instruments, Inc., Québec, Canada). The samples were then oven-dried at 70 °C to a constant mass and the specific root length (SRL) was calculated as the ratio of the root length to dry mass.
Water potential of leaves
At 10 and 60 weeks after first pruning, the pre-dawn water potential was determined for healthy, fully expanded current-year leaves using a WP4 Dewpoint Potentiometer (Decagon Devices, Inc., Pullman, WA, USA). Measurements were taken on four randomly selected trees in each treatment. The leaf samples were cut with a sharp razor blade, sealed immediately in small plastic bags containing moist paper towels and kept in a cooler for a short time before analyses. In addition, to investigate the osmotic adjustment induced by the asymmetrical pruning, the concentrations of free proline and total free amino acids of leaves were determined according to Lei et al. (2006).
Gas exchange measurements
At 10 and 60 weeks after first pruning, measurements of leaf photosynthesis and fine-root respiration were conducted between 08.00–11.30 h using a LI-6400 portable gas exchange system (Li-Cor Inc., Lincoln, NE, USA). Measurements were taken on four randomly selected trees in each treatment.
The measurement conditions for leaf photosynthesis were as follows (Dong et al., 2015): leaf temperature 25 °C; relative air humidity 60%; CO2 concentration 400 μmol mol−1; leaf-to-air vapor pressure deficit 1.5±0.5 kPa; and photosynthetic photon flux density (PPFD) 1500 μmol m−2 s−1 provided by the LED light source of the equipment. Once photosynthesis was fully induced and steady-state gas exchange rates were observed under these conditions, the net photosynthesis rate (light-saturated rate, A; Dong et al., 2015), stomatal conductance (gs), and transpiration rate (E) were recorded. The leaf dark respiration rate was measured under the same conditions except for the absence of light. The respiration rate was recorded when a steady-state rate was observed, but the leaves were darkened for at least 5 min before recording the rates. After taking the measurements, the section of each leaf enclosed in the leaf chamber was scanned and its area was determined using ImageJ.
For the measurement of respiration of fine roots, three intact lateral roots were clipped from each of the trees and the cut surfaces were sealed with Vaseline to prevent water loss and callus respiration (respiration is usually higher from a wounded organ part than from a non-wounded part). The roots were then immediately washed carefully in deionized water. Excess water was removed using absorbent paper. The root samples were stored in an ice box and taken within 2 h from clipping to the lab and placed inside a plexiglass cuvette (7.5 cm diameter × 3.5 cm length) for measurement using the LI-6400 photosynthesis system. The cuvette was covered by a black cloth and measurements of respiration were carried out with a CO2 concentration of 400 μmol mol−1 and a cuvette temperature of 20 °C. The chamber temperature was controlled by a central air-conditioner. After completion of the measurements, root dry mass and length measurements were conducted, as described above. To examine the physiological responses of fine roots after pruning, the root vitality was determined according to Dong et al. (2016).
Leaf nitrogen and phosphorus concentrations
The leaves measured for gas exchange were oven-dried at 70 °C to constant mass. Dried samples were then ground in a ball mill and used for determination of nitrogen and phosphorus concentrations. The nitrogen concentration was measured using a Vario MAX CN analyser (Elementar Analysensysteme GmbH, Hanau, Germany) and the phosphorus concentration using induced plasma emission spectroscopy (Optima 8300, Perkin Elmer Inc., Norwalk, CT, USA).
Non-structural carbohydrate content of leaves and fine roots
The dried and fine-ground current-year leaf and fine-root samples from each treatment were incubated in 80% (w/v) ethanol at 80 °C for 30 min and centrifuged at 5000 g for 10 min. Total soluble sugars were determined in the ethanol extracts and the residues were used to determine the starch content after hydrolysing to glucose with 9.2 M HClO4. Total soluble sugars and starch contents were estimated by the anthrone assay using 0.2% anthrone in concentrated H2SO4 as the reagent. Total soluble sugars were detected colorimetrically at 625 nm (Yemm and Willis, 1954). The amount of total non-structural carbohydrates (NSCT) was calculated as the sum of soluble sugars and starch.
Isotope labeling experiments
Study site and materials
To examine the effects of partial pruning on carbon and nutrient translocation and allocation, 2-year-old C. lanceolata saplings with full root systems and attached soil were carefully excavated from the site of the field experiment. The saplings were transplanted to a greenhouse at the Chengdu Institute of Biology, Chinese Academy of Sciences (average day/night temperature ~25/15 °C and average relative humidity ~60% during the treatment period). Individual saplings were grown in the center of plastic pots (~60 l volume) filled with 50 kg of homogenized yellow soil from the experimental site. During the second growing season in the greenhouse, saplings with approximately the same crown size and height were chosen for the experiments.
The same pruning treatments as in the main experiment were used (NP, BP, RP, SP, OP). The first pruning treatments were applied on 10–11 May 2013 when the new leaves were fully expanded, and repeated pruning treatments each month involved the excision of new lateral branches and roots. On 20–23 August (15 weeks after pruning), three trees from each treatment were randomly chosen for 13C- and 15N-labeling experiments, and three trees from each treatment were used as controls (unlabeled).
13C and 15N labeling procedure
The 13C-labeling procedure was conducted according to Zhang et al. (2005) with some modifications. One healthy lateral branch from the middle crown per individual was chosen. A 0.5-g sample of Ba13CO3 (93% abundance of 13C; Shanghai Engineering Research Center of Stable Isotopes, China) was added into a 25-ml glass vial. After the branch was sealed and the CO2 concentration had decreased to near equilibrium, a pulse of 13CO2 was released by injecting 3 ml 70% H2SO4 from a syringe through a gas port into the Ba13CO3 solution. A similar procedure with an equal amount of Ba12CO3 was carried out for the control trees. The branches were exposed to 13CO2 (labeled) or 12CO2 (unlabeled) in a transparent polycarbonate bag. The labeling was carried out on a sunny day from 09.00–11.00 h. Three lateral fine-root samples (sub-samples from three intact and attached lateral roots per individual) and current-year leaf samples from lateral branches in the mid-crown from three individuals (forming one pooled sample per tree) were harvested from the labeled and control trees 72 h later.
For 15N-labeling, five intact and attached lateral roots per individual were selected. The roots were placed into a glass vial with a black cover that contained a solution of K15NO3 (99% abundance of 15N; Shanghai Engineering Research Center of Stable Isotopes, China) containing 100 μM N l−1 (20 mg per plant). A similar procedure with an equal amount of unlabeled K14NO3 was conducted for control trees. The solutions also contained 10 mg l−1 ampicillin to minimize microbial activity and 0.2 mM CaCl2 to maintain the function of roots (Warren and Adams, 2007). Three current-year leaf samples from lateral branches in the mid-crown and lateral fine-root samples of three individuals were harvested from the labeled and control trees 72 h later.
Carbon and nitrogen isotope analysis
δ13C of the fine-root samples and δ15N of current-year leaf samples from labeled and non-labeled individuals were measured with a combined system of an elemental analyser (Flash EA1112 HT; Thermo Fisher Scientific) and an isotope ratio mass spectrometer (DELTA V Advantage; Thermo Fisher Scientific). We determined excess δ13C (‰) and δ15N as deviations from baseline values (δ13Cbaseline or δ15Nbaseline; values for each tree from each treatment were calculated by averaging the δ13C or δ15N values of the non-labeled individuals) as excess δ13C = (δ13Csample−δ13Cbaseline) and excess δ15N = (δ15Nsample−δ15Nbaseline), respectively (Kagawa et al., 2006a).
Excess 13C of the fine-root samples (from the three target roots) or excess 15N of current-year leaf samples (from one target branch) were also calculated according to procedures described by Kagawa et al. (2006b). To determine different amounts of 13C and 15N translocated into specific tree parts, the ratios of excess 13C in target fine roots and 15N in target current-year leaves of each tree part relative to the excess 13C in the labeled current-year leaves of each target lateral branch or 15N of the fine roots of the target lateral root were calculated. The relative ratio of excess 13C was calculated as excess 13Ctarget fine roots/excess 13Clabeled current-year leaves, and the relative ratio of excess 15N was calculated as excess 15Ntarget current-year leaves/excess 15Nlabeled fine roots.
Statistical analyses
Differences in the effects of pruning treatments on growth, morphology, and physiology (means of data for each leaf/branch/root from each tree side in each individual in each treatment used) were examined using ANOVA. Tukey’s post hoc test was conducted if the differences were significant (P<0.05). Our previous studies had shown that the allocation, chemical, and physiological traits of C. lanceolata saplings were not significantly different across azimuthal directions in non-pruned control plants (Dong et al., 2015, 2016). Therefore, for branches, leaves, and fine roots in the controls (no pruning treatment) we averaged the ecophysiological data (dry mass per branch and leaf, leaf nutrient concentration, gas exchange traits, fine-root mass distribution among the quadrants) for each individual. Prior to ANOVA, the data were checked for normality and homogeneity of variances, and log-transformed to correct deviations from these assumptions when needed. All statistical analyses were carried out using the SPSS 18.0 for Windows statistical software package. Principal component analysis (PCA) of eco-physiological traits in the field experiment was also undertaken to examine the effects of position-related pruning.
Results
Effects of pruning on tree growth, and dry mass and morphology of branches
At the whole-tree level, growth increments of basal diameter and height were significantly different among the five treatments at both 10 and 60 weeks after pruning (Fig. 2). Height growth decreased following pruning, and the lowest values were observed when branches and roots on the opposite sides of the tree were pruned (OP treatment; 66.7% lower at 10 weeks and 37.8% at 60 weeks relative to the NP controls). An increase in basal diameter was observed in trees with half of the lateral roots pruned (RP). Trees with half of the lateral branches pruned (BP) had a lower basal diameter increment (27.3%) at 60 weeks compared with controls, but showed no difference in height. Pruning on opposite sides (OP) had a greater negative effect on basal diameter and height increments compared to pruning on the same side (SP).
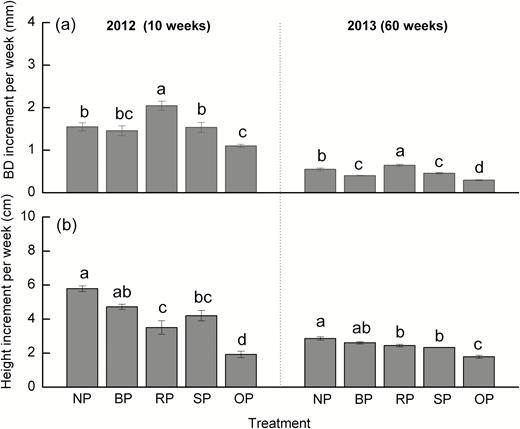
Weekly increments of (a) basal stem diameter (BD) and (b) tree height of C. lanceolata in each treatment as defined in Fig. 1, based on measurements taken 10 weeks and 60 weeks after first pruning. Data are means (±SE), n=4. Different letters indicate significant differences among the pruning treatments at each time according to Tukey’s tests (P<0.05).
The BP treatment resulted in the highest values of branch mass (30.3% higher at 10 weeks and 40.7% higher at 60 weeks after pruning relative to controls) and length (14.8% higher at 10 weeks and 22.3% higher at 60 weeks) (Table 1). The lowest values were recorded in the OP treatment. In the RPS treatment (same orientation of pruned roots and branches) there were reductions in branch mass (20.2% lower) and length (15.1% lower) compared with the RPO treatment (opposite orientation of pruning) at 10 weeks, but values were similar at 60 weeks. There were no significant differences in branch mass and length between SP and NP at either time-point.
Effects of different pruning treatments on the structural characteristics of branches and current-year leaves, and on nutrient concentrations and water potential of current-year leaves in C. lanceolata at 10 weeks and 60 weeks after pruning
. | Treatment . | Branch mass (g branch−1) . | Branch length (cm branch−1) . | Leaf mass (mg leaf−1) . | Leaf area (cm2 leaf−1) . | Nitrogen (mg g−1) . | Phosphorus (mg g−1) . | Water potential (MPa) . |
---|---|---|---|---|---|---|---|---|
10 weeks (2012) | NPL | 26.7±0.2b | 56.2±0.7b | 15.3±0.4a | 1.25±0.03ab | 18.9±0.5ab | 1.14±0.03b | –0.73±0.03ab |
BPL | 34.8±1.4a | 64.5±1.3a | 13.3±0.5ab | 1.33±0.06a | 20.8±1.1a | 1.35±0.02a | –0.54±0.04a | |
RPSL | 19.7±1.3c | 45.0±1.5c | 11.4±0.9b | 1.08±0.06b | 16.1±0.3c | 1.10±0.04b | –0.97±0.04c | |
RPOL | 24.7±0.3b | 53.0±1.1b | 13.5±0.2ab | 1.27±0.06ab | 15.4±0.2c | 1.14±0.03b | –0.81±0.03bc | |
SPL | 25.6±1.2b | 56.8±1.3b | 14.5±0.9ab | 1.30±0.07ab | 16.7±0.2bc | 1.18±0.04b | –0.92±0.04c | |
OPL | 16.8±0.8c | 38.9±2.4c | 8.1±0.3c | 0.79±0.01c | 17.6±0.2bc | 1.37±0.03a | –1.5±0.07d | |
F | 41.27*** | 39.96*** | 17.96*** | 14.45*** | 14.35*** | 12.55*** | 58.01*** | |
60 weeks (2013) | NPL | 36.6±0.9b | 79.1±1.0b | 14.8±0.6a | 1.27±0.04ab | 17.1±0.9ab | 1.21±0.05bc | –0.87±0.04ab |
BPL | 51.1±0.7a | 96.6±3.0a | 13.1±0.4a | 1.22±0.02ab | 18.4±1.3a | 1.48±0.07a | –0.59±0.09a | |
RPSL | 35.7±1.9b | 73.7±1.7b | 12.4±0.2a | 1.09±0.02b | 16.3±0.5ab | 1.35±0.02ab | –1.0±0.05b | |
RPOL | 36.5±1.1b | 79.2±1.6b | 13.7±0.8a | 1.22±0.08ab | 13.9±0.3bc | 1.16±0.03bc | –0.89±0.01ab | |
SPL | 36.7±1.1b | 78.6±1.0b | 13.7±0.4a | 1.33±0.03a | 16.9±0.6ab | 1.31±0.04ab | –1.2±0.1b | |
OPL | 28.0±1.3c | 63.5±0.9c | 7.0±0.6b | 0.68±0.06c | 12.5±0.7c | 1.02±0.05c | –1.8±0.1c | |
F | 38.27*** | 40.12*** | 27.41*** | 25.33*** | 7.85*** | 12.56*** | 27.34*** |
. | Treatment . | Branch mass (g branch−1) . | Branch length (cm branch−1) . | Leaf mass (mg leaf−1) . | Leaf area (cm2 leaf−1) . | Nitrogen (mg g−1) . | Phosphorus (mg g−1) . | Water potential (MPa) . |
---|---|---|---|---|---|---|---|---|
10 weeks (2012) | NPL | 26.7±0.2b | 56.2±0.7b | 15.3±0.4a | 1.25±0.03ab | 18.9±0.5ab | 1.14±0.03b | –0.73±0.03ab |
BPL | 34.8±1.4a | 64.5±1.3a | 13.3±0.5ab | 1.33±0.06a | 20.8±1.1a | 1.35±0.02a | –0.54±0.04a | |
RPSL | 19.7±1.3c | 45.0±1.5c | 11.4±0.9b | 1.08±0.06b | 16.1±0.3c | 1.10±0.04b | –0.97±0.04c | |
RPOL | 24.7±0.3b | 53.0±1.1b | 13.5±0.2ab | 1.27±0.06ab | 15.4±0.2c | 1.14±0.03b | –0.81±0.03bc | |
SPL | 25.6±1.2b | 56.8±1.3b | 14.5±0.9ab | 1.30±0.07ab | 16.7±0.2bc | 1.18±0.04b | –0.92±0.04c | |
OPL | 16.8±0.8c | 38.9±2.4c | 8.1±0.3c | 0.79±0.01c | 17.6±0.2bc | 1.37±0.03a | –1.5±0.07d | |
F | 41.27*** | 39.96*** | 17.96*** | 14.45*** | 14.35*** | 12.55*** | 58.01*** | |
60 weeks (2013) | NPL | 36.6±0.9b | 79.1±1.0b | 14.8±0.6a | 1.27±0.04ab | 17.1±0.9ab | 1.21±0.05bc | –0.87±0.04ab |
BPL | 51.1±0.7a | 96.6±3.0a | 13.1±0.4a | 1.22±0.02ab | 18.4±1.3a | 1.48±0.07a | –0.59±0.09a | |
RPSL | 35.7±1.9b | 73.7±1.7b | 12.4±0.2a | 1.09±0.02b | 16.3±0.5ab | 1.35±0.02ab | –1.0±0.05b | |
RPOL | 36.5±1.1b | 79.2±1.6b | 13.7±0.8a | 1.22±0.08ab | 13.9±0.3bc | 1.16±0.03bc | –0.89±0.01ab | |
SPL | 36.7±1.1b | 78.6±1.0b | 13.7±0.4a | 1.33±0.03a | 16.9±0.6ab | 1.31±0.04ab | –1.2±0.1b | |
OPL | 28.0±1.3c | 63.5±0.9c | 7.0±0.6b | 0.68±0.06c | 12.5±0.7c | 1.02±0.05c | –1.8±0.1c | |
F | 38.27*** | 40.12*** | 27.41*** | 25.33*** | 7.85*** | 12.56*** | 27.34*** |
Data are means (±SE), n=4. Values followed by the same letter in the same column are not significantly different at P<0.05 according to Tukey’s tests. All factorial analyses (ANOVA) are significant at ***P≤0.001. Treatments are as defined in Fig. 1.
Effects of different pruning treatments on the structural characteristics of branches and current-year leaves, and on nutrient concentrations and water potential of current-year leaves in C. lanceolata at 10 weeks and 60 weeks after pruning
. | Treatment . | Branch mass (g branch−1) . | Branch length (cm branch−1) . | Leaf mass (mg leaf−1) . | Leaf area (cm2 leaf−1) . | Nitrogen (mg g−1) . | Phosphorus (mg g−1) . | Water potential (MPa) . |
---|---|---|---|---|---|---|---|---|
10 weeks (2012) | NPL | 26.7±0.2b | 56.2±0.7b | 15.3±0.4a | 1.25±0.03ab | 18.9±0.5ab | 1.14±0.03b | –0.73±0.03ab |
BPL | 34.8±1.4a | 64.5±1.3a | 13.3±0.5ab | 1.33±0.06a | 20.8±1.1a | 1.35±0.02a | –0.54±0.04a | |
RPSL | 19.7±1.3c | 45.0±1.5c | 11.4±0.9b | 1.08±0.06b | 16.1±0.3c | 1.10±0.04b | –0.97±0.04c | |
RPOL | 24.7±0.3b | 53.0±1.1b | 13.5±0.2ab | 1.27±0.06ab | 15.4±0.2c | 1.14±0.03b | –0.81±0.03bc | |
SPL | 25.6±1.2b | 56.8±1.3b | 14.5±0.9ab | 1.30±0.07ab | 16.7±0.2bc | 1.18±0.04b | –0.92±0.04c | |
OPL | 16.8±0.8c | 38.9±2.4c | 8.1±0.3c | 0.79±0.01c | 17.6±0.2bc | 1.37±0.03a | –1.5±0.07d | |
F | 41.27*** | 39.96*** | 17.96*** | 14.45*** | 14.35*** | 12.55*** | 58.01*** | |
60 weeks (2013) | NPL | 36.6±0.9b | 79.1±1.0b | 14.8±0.6a | 1.27±0.04ab | 17.1±0.9ab | 1.21±0.05bc | –0.87±0.04ab |
BPL | 51.1±0.7a | 96.6±3.0a | 13.1±0.4a | 1.22±0.02ab | 18.4±1.3a | 1.48±0.07a | –0.59±0.09a | |
RPSL | 35.7±1.9b | 73.7±1.7b | 12.4±0.2a | 1.09±0.02b | 16.3±0.5ab | 1.35±0.02ab | –1.0±0.05b | |
RPOL | 36.5±1.1b | 79.2±1.6b | 13.7±0.8a | 1.22±0.08ab | 13.9±0.3bc | 1.16±0.03bc | –0.89±0.01ab | |
SPL | 36.7±1.1b | 78.6±1.0b | 13.7±0.4a | 1.33±0.03a | 16.9±0.6ab | 1.31±0.04ab | –1.2±0.1b | |
OPL | 28.0±1.3c | 63.5±0.9c | 7.0±0.6b | 0.68±0.06c | 12.5±0.7c | 1.02±0.05c | –1.8±0.1c | |
F | 38.27*** | 40.12*** | 27.41*** | 25.33*** | 7.85*** | 12.56*** | 27.34*** |
. | Treatment . | Branch mass (g branch−1) . | Branch length (cm branch−1) . | Leaf mass (mg leaf−1) . | Leaf area (cm2 leaf−1) . | Nitrogen (mg g−1) . | Phosphorus (mg g−1) . | Water potential (MPa) . |
---|---|---|---|---|---|---|---|---|
10 weeks (2012) | NPL | 26.7±0.2b | 56.2±0.7b | 15.3±0.4a | 1.25±0.03ab | 18.9±0.5ab | 1.14±0.03b | –0.73±0.03ab |
BPL | 34.8±1.4a | 64.5±1.3a | 13.3±0.5ab | 1.33±0.06a | 20.8±1.1a | 1.35±0.02a | –0.54±0.04a | |
RPSL | 19.7±1.3c | 45.0±1.5c | 11.4±0.9b | 1.08±0.06b | 16.1±0.3c | 1.10±0.04b | –0.97±0.04c | |
RPOL | 24.7±0.3b | 53.0±1.1b | 13.5±0.2ab | 1.27±0.06ab | 15.4±0.2c | 1.14±0.03b | –0.81±0.03bc | |
SPL | 25.6±1.2b | 56.8±1.3b | 14.5±0.9ab | 1.30±0.07ab | 16.7±0.2bc | 1.18±0.04b | –0.92±0.04c | |
OPL | 16.8±0.8c | 38.9±2.4c | 8.1±0.3c | 0.79±0.01c | 17.6±0.2bc | 1.37±0.03a | –1.5±0.07d | |
F | 41.27*** | 39.96*** | 17.96*** | 14.45*** | 14.35*** | 12.55*** | 58.01*** | |
60 weeks (2013) | NPL | 36.6±0.9b | 79.1±1.0b | 14.8±0.6a | 1.27±0.04ab | 17.1±0.9ab | 1.21±0.05bc | –0.87±0.04ab |
BPL | 51.1±0.7a | 96.6±3.0a | 13.1±0.4a | 1.22±0.02ab | 18.4±1.3a | 1.48±0.07a | –0.59±0.09a | |
RPSL | 35.7±1.9b | 73.7±1.7b | 12.4±0.2a | 1.09±0.02b | 16.3±0.5ab | 1.35±0.02ab | –1.0±0.05b | |
RPOL | 36.5±1.1b | 79.2±1.6b | 13.7±0.8a | 1.22±0.08ab | 13.9±0.3bc | 1.16±0.03bc | –0.89±0.01ab | |
SPL | 36.7±1.1b | 78.6±1.0b | 13.7±0.4a | 1.33±0.03a | 16.9±0.6ab | 1.31±0.04ab | –1.2±0.1b | |
OPL | 28.0±1.3c | 63.5±0.9c | 7.0±0.6b | 0.68±0.06c | 12.5±0.7c | 1.02±0.05c | –1.8±0.1c | |
F | 38.27*** | 40.12*** | 27.41*** | 25.33*** | 7.85*** | 12.56*** | 27.34*** |
Data are means (±SE), n=4. Values followed by the same letter in the same column are not significantly different at P<0.05 according to Tukey’s tests. All factorial analyses (ANOVA) are significant at ***P≤0.001. Treatments are as defined in Fig. 1.
Effects of pruning on dry mass, morphology, nutrient content, and physiology of leaves
At the leaf level, the average dry mass (ML) and area (AL) per leaf were the lowest in the OP treatment (47.1% lower for ML and 36.8% for AL at 10 weeks, and 52.7% lower for ML and 46.5% lower for AL at 60 weeks compared to the controls) (Table 1). ML was lower in RPS than in NP (25.5% and 16.2% lower at 10 and 60 weeks, respectively). In the RP treatments, ML of RPS was slightly lower than that of RPO.
Leaf nitrogen and phosphorus concentrations observed in the BP treatment were higher than those in RP (Table 1). The phosphorus concentration was highest in the OP treatment at 10 weeks after pruning but lowest at 60 weeks. The leaf nitrogen concentration was similar in the SP and OP treatments at 10 weeks, but it was lower in OP at 60 weeks. Nitrogen and phosphorus were not significantly different between RPO and RPS, and between SP and NP.
The BP treatment increased the light-saturated photosynthetic rate (A) at 60 weeks after pruning, but it did not affect the leaf respiration rate (RL) at either time-point (Fig. 3a, b). The OP treatment decreased A and increased RL at 60 weeks after pruning. At 10 weeks, stomatal conductance (gs) was generally higher in the pruning treatments compared to no pruning, but values were similar among treatments at 60 weeks (Fig. 3c). Pruning tended to increase the leaf transpiration rate (E) at 10 weeks and caused significant increases in all treatments at 60 weeks (Fig. 3d) and, with the exception of BP at 10 weeks, it consistently decreased the water-use efficiency (WUE, ratio of A/E) (Supplementary Fig. S1b). The transpiration rate was greater in the RP treatment than in the non-pruned treatment, and E in RPSL was similar with that in RPOL at either time-point (Fig. 3d). E was higher in OP than in SP at 10 weeks. At 60 weeks, E was similar in all the pruned treatments and was significantly higher than in the non-pruned control. Pruning also resulted in reduced leaf water potential (Ψ), except in BP (Table 1). The lowest values of WUE and Ψ were found in OP, while WUE and Ψ values in RPSL and RPOL treatments were similar (Table 1; Supplementary Fig. S1b). Photosynthetic nitrogen-use efficiency (NUE) and phosphorus-use efficiency (PUE) were not affected by pruning treatments (Supplementary Fig. S1c, d). The amounts of free proline and free amino acids (FAA) of leaves were positively affected by pruning for SP and OP treatments (Supplementary Fig. S2).
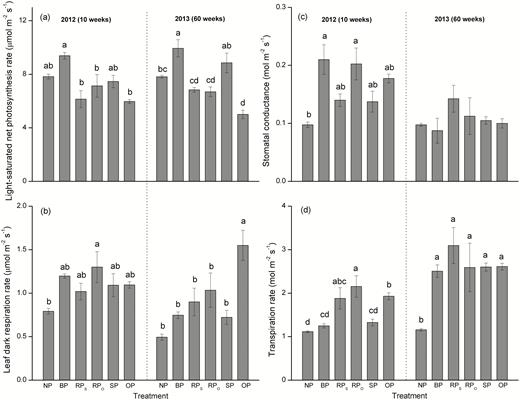
Light-saturated photosynthetic rate (a), dark respiration rate (b), stomatal conductance (c), and transpiration rate (d) of current-year leaves of C. lanceolata in each treatment as defined in Fig. 1 at 10 weeks and 60 weeks after first pruning. Data are means (±SE), n=4. Different letters indicate significant differences among the pruning treatments at each time according to Tukey’s tests (P<0.05).
Effects of pruning on dry mass, morphology, physiology, and nutrient contents of roots
The specific root length (SRL) of the fine roots varied significantly among pruning treatments (Fig. 4a). Compared with the controls (NP), SRL was highest in RP (24.0% greater at 10 weeks, 36.4% greater at 60 weeks) and lowest in OP (35.0% lower at 10 weeks, 43.4% lower at 60 weeks). SRL values in the BP treatments for roots on the same (BPSR) and opposite (BPOR) side to pruning were lower than in the control (25.1% lower for BPSR and 31.7% lower for BPOR at 10 weeks, and 47.10 lower for BPS and 14.1% lower for BPOR at 60 weeks). The SRL of BPSR was 38.2% lower than that of BPOR at 60 weeks.
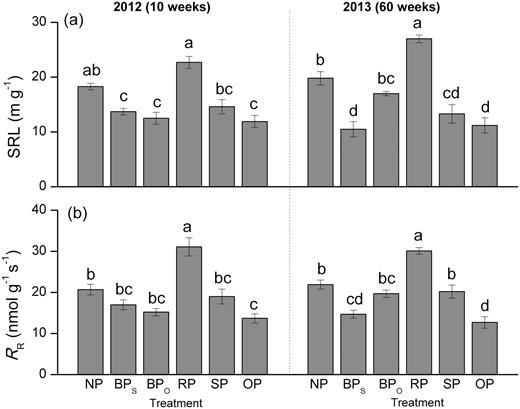
Specific fine-root length (SRL) and fine-root respiration rate (RR) of C. lanceolata in each treatment as defined in Fig. 1 at 10 weeks and 60 weeks after first pruning. Data are means (±SE), n=4. Different letters indicate significant differences among the pruning treatments according to Tukey’s tests (P<0.05).
Analyses of differences in root vitality indicated that it was increased by RP but decreased by BP (Supplementary Fig. S3). The highest rate of respiration in fine roots (RR) was observed in RP, and the lowest in OP (Fig. 4b). At 60 weeks, RR of BPSR was slightly lower than that of BPOR, and it was significantly lower in OP than in SP. The SP treatment did not change root vitality relative to the control, but in OP the vitality was lower than in the other treatments apart from BPSR. A greater difference in root vitality of BPSR than of BPOR to NPR was observed (Supplementary Fig. S3).
Effects of pruning on leaf and root non-structural carbohydrates
At 10 weeks after pruning, the contents of leaf soluble sugars and total non-structural carbohydrate (NSCT) in the various pruning treatments were not significantly different from those in the control plants (Fig. 5a); however, at 60 weeks, pruning generally decreased leaf sugar and NSCT contents, and both were lowest in BP. In the RP treatment, leaf sugars and NSCT were lower in RPSL than in RPOL at 10 weeks, but these differences were not observed at 60 weeks. Compared to SP, leaf soluble sugars and NSCT were slightly higher in the OP treatment. The starch content was the lowest in SP at 10 weeks, and in OP at 60 weeks.
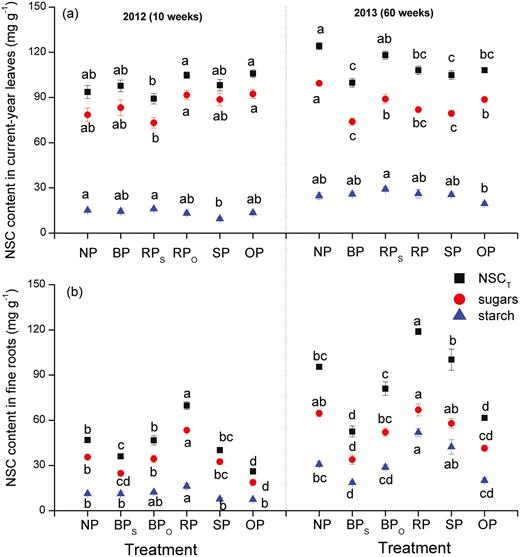
Contents of soluble sugars, starch, and total non-structural carbohydrates (NSCT, equal to soluble sugars + starch) per unit dry mass in current-year leaves (a) and fine roots (b) of C. lanceolata in each treatment as defined in Fig. 1 at 10 weeks and 60 weeks after first pruning. Data are means (±SE), n=4. Different letters indicate significant differences among the pruning treatments according to Tukey’s tests (P<0.05).
In fine roots, starch and NSCT increased in the RP treatment, especially at 60 weeks (Fig. 5b). In the BP treatments, NSCT was lower in BPSR than in BPOR at both time-points, but the starch content was only lower at 60 weeks. Both the NSCT and starch contents were similar to those of the control at both time-points. When both shoots and roots were pruned, NSCT was lower in OP than in SP at both time-points but the starch content was only lower at 60 weeks. The contents of sugar, starch, and NSCT were similar to the control at both time-points.
Effects of pruning on carbon and nitrogen translocation and allocation
Both the excess δ13C and excess 13C of fine roots located on the same side of the plant as the labeled branch were significantly higher than those in roots on the opposite side (Fig. 6a, b). Compared to the NP control, the excess δ13C of fine roots located on the same side as the labeled branch was lower in BP. There were no significant differences in δ13C of fine roots located on the opposite side relative to the labeled branch in BP, RP and OP treatments compared to NP(Fig. 6a), but the value of δ13C of BPOR was lower than that of the OP treatment. Excess 13C and the relative ratio of 13C (excess 13Ctarget fine roots/excess 13Clabeled current-leaves) in fine roots were highest in the RP treatment, both for the same and opposite sides to the labeled branch (Fig. 6b, c); apart from the relative ratio of 13C in OP, there were no differences among NP, BP and OP.
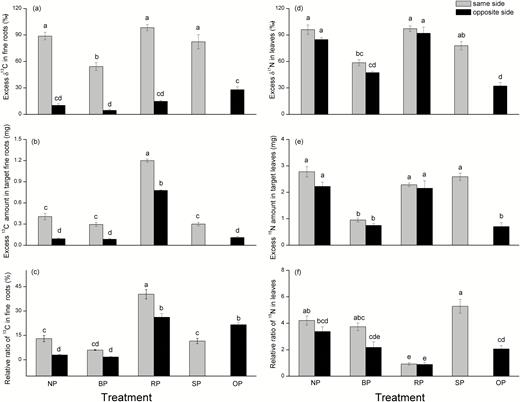
(a) Excess δ13C and (b) excess 13C in sampled (target) fine roots, and (c) relative ratio of excess 13C in fine roots (excess 13C in sampled fine roots/excess 13C in the labelled current-year leaves), (d) excess δ15N and (e) excess 15N in sampled current-year leaves, and (f) relative ratio of excess 15N in current-year leaves (excess 15N in sampled current-year leaves/excess 15N in the labelled fine roots) for samples from C. lanceolata taken from either the same or opposite side of the plant relative to the pruning treatment as defined in Fig. 1; isotope labelling was applied at 15 weeks after pruning and samples were taken 72 h after labelling. Data are means (±SE), n=3.
The vales of excess δ15N, excess 15N, and relative ratio of 15N (excess 15Ntarget current-leaves/excess 15Nlabeled fine roots) were similar for current-year leaves located on the same and opposite sides of the plants relative to the labeled roots (Fig. 6d–f). SP and NP had similar values for all three traits. The lowest values of excess δ15N and excess 15N were observed in the OP treatment. The BP and OP treatments resulted in a decrease in leaf excess δ15N, while RP and SP did not have any effect (Fig. 6d). Excess 15N was decreased in the RP and SP treatments (Fig. 6e). The lowest relative ratio of 15N in current-year leaves among pruning treatments was observed in RP (Fig. 6f).
Relationships among traits in shoots under different pruning treatments
The PCA showed clear delineation based on trait combinations in the different pruning treatments (Fig. 7). The treatments were well separated from each other at both 10 weeks (Fig. 7a) and 60 weeks after pruning (Fig. 7b). The two-component PCA models explained 57.1% and 67.5% of the observed total variance at 10 weeks and 60 weeks, respectively. PC1 was strongly influenced by height growth, leaf area and mass, photosynthetic rate, transpiration rate, and water potential. PC2 was strongly influenced by diameter growth, leaf non-structural carbohydrates, and phosphorus concentrations.
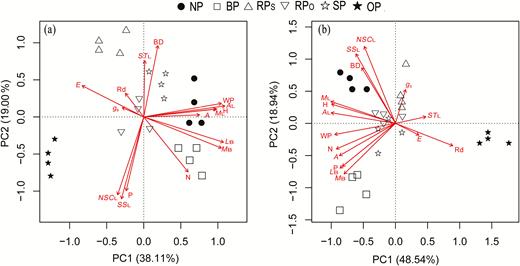
Principal component analysis (PCA) based on eco-physiological shoot traits in each pruning treatment as defined in Fig. 1. at (a) 10 weeks and (b) 60 weeks after pruning. BD, stem basal diameter increment per week; H, stem height increment per week; MB, dry mass per branch; LB, length per branch; ML, dry mass per leaf; AL, area per leaf; A, photosynthetic rate; gs, stomatal conductance; Rd, dark respiration rate of leaf; E, transpiration rate; N, leaf nitogen concentration; P, leaf phosphorus concentration; SSL, leaf soluble sugar concentration; STL, leaf starch concentration; NSCL, leaf non-structural carbohydrate concentration; WP, leaf water potential.
Discussion
We found that partial branch and root pruning changed tree growth, carbon and nitrogen allocation, water and nutrient status, and physiological processes of leaves and fine roots in ways similar to some previous studies on trees (e.g. Li et al., 2002; Eyles et al., 2013; Aguadé et al., 2015; Wiley et al., 2017). However, the responses varied depending on the relative lateral positions of the pruned branches and roots. There was a significantly higher allocation of photosynthate to fine roots from the branches on the same side of the tree compared to those on the opposite side, while nitrogen allocation to leaves was not significantly different between roots on the same or opposite sides of the. Differences in morphology and physiology between fine roots on the same and opposite sides to branch pruning increased with time, but differences between current-year leaves on the same and opposite sides to root pruning decreased. Our results thus demonstrated that (1) the position of pruning had a strong influence on growth, photosynthesis, and carbon storage and remobilization, as well as on water- and nutrient-related physiological responses; and (2) compared to leaves, carbon availability for fine roots was more strongly affected by partial pruning.
Plant responses to shoot pruning versus root pruning
Once the distribution of shoots or roots is altered, adjustments in physiology, morphology, and growth will begin, and these adjustments may happen at the organ or whole-plant level until a new balance is reached (Pinkard et al., 2011; Eyles et al., 2013; Dong et al., 2016). Although the growth of branch and root apical parts and secondary stem thickening form characteristic sinks for photoassimilates during the summer, we observed decreases in growth of basal stem diameter (Fig. 2) and root respiration rate (RR) (Fig. 4b) at the longer pruning time (60 weeks) and root vitality (Supplementary Fig. S3), and lower root non-structural carbohydrates content in BPSR (Fig. 5b) when branches were pruned. Overall, our results showed that the source limitation induced by branch pruning resulted in carbon depletion and lowered metabolic capacity in roots, and consequently in decreased uptake of water and nutrients (see also Kosola et al., 2002; Kobe et al., 2010). This was supported by the nitrogen isotope labeling study (Fig. 6d, e). A decrease in growth may be beneficial for maintaining the concentration of non-structural carbohydrates in leaves (Wiley et al., 2013). Our finding that stem growth (height and basal diameter increments) had a positive relationship with leaf NSC (Fig. 7) at 60 weeks may explain the observation that leaf NSC decreased (Fig. 5a). On the other hand, plants can have compensatory responses after partial branch pruning including increases in the photosynthetic rate, and the biomass and length of remaining branches (Paul and Foyer, 2001; Pinkard et al., 2011; Eyles et al., 2013; Asao and Ryan, 2015). In our study, partial branch pruning (about 50% leaf area lost) did not significantly affect the stem height and basal diameter growth increments (except for basal diameter at 60 weeks). This weak response reflected increases in leaf area and photosynthetic rate (Fig. 7), with the latter increase being due to increased leaf nitrogen and phosphorus concentrations in the remaining foliage (Table 1), but not due to changes in nitrogen- and phosphorus-use efficiencies (Supplementary Fig. S1c, d). These results further confirmed the onset of plant compensatory responses after branch pruning.
Under only partial root pruning (RP), the basal stem diameter growth was greater, despite the decreased height growth. When branch and leaf growth were compared to the non-pruned control plants, a lower dry mass per branch and leaf (mainly seen with root pruning on the same side) was detected in RP 10 weeks after pruning (Table 1), but there were on effects on the growth of branches and leaves at 60 weeks after pruning. Our results indicated that physiological (e.g. root respiration rate and root vitality) and morphological (e.g. specific fine root length) compensation responses of the fine roots could maintain the water and nutrient supply to the leaves, and this conclusion was further supported by the results from the nitrogen isotope labelling (Fig. 6d). The down-regulation of photosynthesis after partial pruning of the roots may have mainly been caused by the limitation of the carbon sink storage capacity (initial root and shoot height growth decreased) rather than by water or nitrogen limitation at the whole-plant level (Farrar and Jones, 2000; Paul and Foyer, 2001; Eyles et al., 2013; Dong et al., 2016). In addition, we suggest that the increased basal stem diameter in the RP treatment was mainly caused by greater carbohydrate availability for stem growth, as lateral pruning of roots restricts carbohydrate translocation to the remaining roots (Asao and Ryan, 2015).
Our results clearly demonstrated that the branch-pruning treatment caused a negative effect on fine root growth and metabolic activity, although it also increased the growth and photosynthesis of the remaining branches. On the other hand, a positive effect on the growth of remaining fine roots and metabolic activity was detected in the root-pruning treatment. These responses highlight the acclimation changes in the source–sink balance of carbon allocation after pruning. Excess δ13C, excess 13C, and the relative ratio of 13C in fine roots to that in labelled leaves were lower in the branch-pruning treatments than in the root-pruning treatments (Fig. 6a–c), clearly indicating that photoassimilate partitioning to the roots increased in response to root pruning. Overall, the data also suggested that the carbon status (respiration and NSC concentration) of heterotrophic fine roots changed more strongly than that of autotrophic leaves during asymmetric pruning of shoot and roots.
Bidirectional responses of branches, leaves, and fine roots under partial pruning of branches or roots
It has been demonstrated in non-pruned C. lanceolata plants that the dry mass of branches and leaves, gas exchange characteristics, nutrient concentrations, and water-use traits of leaves and fine roots are similar bilaterally (Dong et al., 2015, 2016). However, when half of the lateral branches or roots were pruned, differences in morphological and physiological responses between roots/branches located on the same and opposite sides to the pruning became significant. Pruning half of the branches induced greater decreases in fine root traits (SRL, RR, vitality, and NSC) on the pruned side than on the opposite side (Figs 4, 5b; Supplementary Fig. S3), while pruning of half the roots did not lead to any differences in most of the leaf traits (e.g. biomass, area, physiological characteristics, and nutrient concentrations) between the pruned and non-pruned sides (Table 1; Fig. 3). These results indicated that the within-plant ecophysiological responses of C. lanceolata were related to the relative positions of the lateral branches and roots, and that the leaf and fine-root responses were different during asymmetric disturbances depending on the position, which suggests that the xylem has better interconnects than the phloem (Thorn and Orians, 2011). Collectively, the results indicate that the plant long-distance transport system (xylem and phloem) plays a crucial role in determining the response of roots and leaves to pruning, as has also been shown in a number of previous studies (Minchin and Lacointe, 2005; De Kroon et al., 2009; Savage et al., 2016).
Thorn and Orians (2011) applied 15N to one half of the root system of Ocimum basilicum and found that it accumulated more in orthostichous leaves (growing at an angular distance of 0°) than in leaves from the opposite sector. This suggests that tighter vascular links exist between roots and branches on the same side of the plant. In our study, excess δ13C and excess 13C (Fig. 6a, b) in fine roots were always higher on the same side than on the opposite side relative to the lateral branches, and the pattern did not change with pruning. However, excess δ15N and excess 15N (Fig. 6d, e) in current-year leaves were not different between the same and opposite sides relative to the pruned lateral roots, despite the mean values being slightly higher on the same side than on the opposite side. The values of excess 13C and excess 15N characterize new carbon and nitrogen allocation within the plant (Kagawa et al., 2006a, 2006b). Thus, the results from our isotope labelling suggest that nitrogen allocation among leaves from different target branches was not related to their orientation. Different allocation patterns between nitrogen and photosynthates in leaves and fine roots may be related to the anatomy and physiology of the xylem and phloem (Savage et al., 2016) rather than to source–sink relationships. Therefore, our results for carbon and nitrogen allocation partially support our expectations.
Responses of branches, leaves, and fine roots to partial pruning
The integrated transport system at the whole-plant level is adaptive as it allows the achievement of high growth rates (Orians et al., 2005; Zanne et al., 2006; Schenk et al., 2008) and improved resistance to biotic stresses (Aguadé et al., 2015), and therefore it has been subject to positive evolutionary selection. The functional integration (transport of water, sugars, and nutrients) between branches and roots within a tree can be mediated by vascular connections (Orians et al., 2002, 2005; Zanne et al., 2006). The key result of our study on C. lanceolata was that the smallest effects on growth and the largest effects on leaf and fine-root morphology and physiology (e.g. stem increment, gas-exchange traits, leaf water and nutrients, and root carbon status) were detected when opposite-side branches and roots were pruned (OP), while branch and fine-root traits were not affected when branches and roots from the same side of the plant were pruned (SP). These results provide the first evidence for the view that trees can maintain the functional balance between lateral branches and roots in orthostichous positions, while the functional linkage in branches and roots on the opposite sides of the plant is relatively easily disturbed. This evidence comes from our isotope-labelling experiments, in which both the excess 15N of leaves and excess 13C of fine roots were significantly lower under opposite-side pruning than under same-side pruning (Fig. 6). This may be due to differences in resistance (related to the distance between source and sink organs and their structures) between transport pathways in the xylem and phloem in different lateral positions (Orians et al., 2002; James et al., 2003; Thorn and Orians, 2011). Thus, the different responses of branches, leaves, and fine roots between OP and SP supports the view that they are related to the position of pruning (Thorn and Orians, 2011). Our results also suggest that nutrients can be transported in transverse directions via the xylem (James et al., 2003; Snyder and Williams, 2003).
Some of the compensatory responses that we observed might reflect physiological adjustments of leaves that enhance the stress resistance of the foliage (Niinemets et al., 2015). The decreased growth under OP was caused by pruning effects on both photosynthesis and carbon assimilation efficiency (photosynthesis rate divided by respiration rate; Supplementary Fig. S1), while gs (Fig. 3c) was not significantly influenced (although a slight increase was observed at 10 weeks after the treatment). This evidence suggests that the decreased photosynthesis rate under OP may have resulted from the reduced maximum carboxylation activity of Rubisco (Flexas et al., 2016). However, a higher transpiration rate (E) was found in OP compared to SP and the control treatment (no pruning). Higher E implies increased leaf water loss, but higher E is also compatible with a greater mass flow from roots to leaves and would be expected to result in a greater nutrient uptake. This possibility was supported by similar nitrogen and higher phosphorus concentrations in leaves (Table 1). We observed greater amounts of soluble sugars, proline, and total free amino acids (FAA) in leaves in the OP treatment (Supplementary Fig. S2), while the leaf water potential was lower in OP than in SP (Table 1). These responses suggest that the OP treatment caused a greater water stress than SP (Lei et al., 2006). Thus, our results indicate that in the OP treatment, C. lanceolata had a higher metabolic cost of leaves (higher rates of respiration and transpiration, lower carbon fixation efficiency and water-use efficiency), whilst these changes may be associated with enhanced nutrient uptake (Dong et al., 2015).
Conclusions
To our knowledge, this study is the first investigation of the responses of both branches and roots to different types of lateral pruning, specifically on the functional balance of carbon, water, and nutrients in branches and roots of tree saplings. We have shown that pruning half of the shoot and/or root system significantly alters tree growth, branch morphology, current-year leaf and fine-root traits, and physiology in C. lanceolata and that these effects are related to the horizontal position of pruning, and same-side versus opposite-side pruning. Our experiments support the source–sink interaction model of branches and roots, and emphasize that the functional balance of carbon distribution between branches and roots is more readily achieved for same-side compared with opposite-side pruning of roots and leaves. Our results provide novel insights into the functional balance of tree carbon and nutrient acquisition and distribution, and water acquisition and use, and into the effects of standard forest management practices on forest carbon storage. Further studies are required to examine the mechanisms of the coupling of carbon and nutrient transport through the xylem and phloem between leaves and roots, and to determine whether similar patterns are observed in other species.
Supplementary data
Supplementary data are available at JXB online.
Fig. S1. Carbon assimilation efficiency, water-use efficiency, photosynthetic nitrogen-use efficiency, and photosynthetic phosphorus-use efficiency of current-year leaves in each treatment at 10 and 60 weeks after pruning.
Fig. S2. Proline content and total free amino acid content of current-year leaves in each treatment at 10 and 60 weeks after pruning.
Fig. S3. Root vitality as determined by the capacity for reduction of triphenyltetrazolium chloride in fine roots in each treatment at 10 and 60 weeks after pruning.
Acknowledgments
This work was supported by the Talent Program of the Hangzhou Normal University (2016QDL020). We are very grateful to Dr Gang Xu, Dr Junyu Li, and Dr Lei Yu for assistance during the field work and to Mr Yafu Yuan for providing the field site. The authors declare that they have no conflicts of interest.
Comments