-
PDF
- Split View
-
Views
-
Cite
Cite
Karin Schlangen, Silvija Miosic, Jana Thill, Heidi Halbwirth, Cloning, functional expression, and characterization of a chalcone 3-hydroxylase from Cosmos sulphureus, Journal of Experimental Botany, Volume 61, Issue 12, July 2010, Pages 3451–3459, https://doi.org/10.1093/jxb/erq169
- Share Icon Share
Abstract
A chalcone 3-hydroxylase (CH3H) cDNA clone was isolated and characterized from Cosmos sulphureus petals accumulating butein (2′,3,4,4′-tetrahydroxychalcone) derivatives as yellow flower pigments. The recombinant protein catalyses the introduction of an additional hydroxyl group in the B-ring of chalcones, a reaction with high similarity to the hydroxylation of flavonoids catalysed by the well-studied flavonoid 3′-hydroxylase (F3′H). CH3H shows high specificity for chalcones, but a low F3′H activity was also detected. By contrast, the common F3′H from C. sulphureus does not accept chalcones as substrates and is therefore unlikely to be involved in the creation of the B-ring hydroxylation pattern of the yellow flower pigments. CH3H was primarily expressed in young buds, the main tissue for chalcone pigment formation. Expression levels in open flowers and 3-d-old seedlings were lower and almost no CH3H expression was observed in leaves. F3′H, in contrast, showed the highest expression also in buds, but comparable expression rates in all other tissues tested. Recombinant hybrid proteins constructed from CH3H and F3′H fragments demonstrated that amino acid residues at a substrate recognition site and an insertion of four amino acid residues in a putative loop region have an impact on chalcone acceptance. This is the first identification of a CH3H cDNA from any plant species.
Introduction
6′-Deoxychalcones frequently occur in floral tissues of plant species from the Asteraceae family, where they contribute to yellow flower colour and may also be involved in the formation of UV-honey guides (Harborne, 1967; Rieseberg and Schilling, 1985). In contrast to the 6-hydroxychalcones which are the immediate precursors for the formation of common flavonoids, 6′-deoxychalcones are not chemically isomerized to the corresponding flavanones (Forkmann and Heller, 1999). Furthermore, chalcone isomerases (CHIs) from Asteraceae species do not accept 6′-deoxychalcones as substrates. Therefore, accumulation of 6′-deoxychalcones as flower pigments is much more frequent compared to 6’-hydroxychalcones (Harborne, 1967). As a rule, two compounds with different hydroxylation patterns in ring B are present, isoliquiritigenin (2′,4,4′-trihdroxychalcone) and butein (2′,3,4,4′-tetrahdroxychalcone) (Fig. 1). Conversion of the 6′-deoxychalcone isoliquiritigenin to the 3-hydroxylated butein can be detected with microsomal enzyme preparations of several Asteraceae species, for example, Dahlia variabilis, Coreopsis grandiflora, and Cosmos sulphureus. This reaction is catalysed by a cytochrome P450-dependent mono-oxygenase (P450) (Wimmer et al., 1998; Schlangen et al., 2009). However, the molecular base for chalcone acceptance still remains a puzzle.
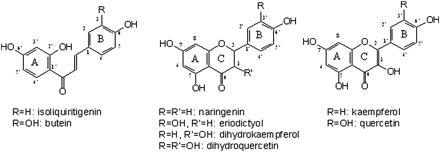
Chemical structures of selected 6’-deoxychalcones, flavanones, and dihydroflavonols.
Recently it has been shown that hydroxylation of chalcones in position 3 is not a general property of flavonoid 3′-hydroxylases (F3′H) (Schlangen et al., 2009) but can be catalysed by distinct F3′Hs with special structural prerequisites. Thus, two allelic variants from Dahlia variabilis encoding flavonoid 3′-hydroxylases were isolated, which differed only in three amino acids within their 508 residues (Schlangen et al., 2010). In this case, the acceptance of chalcones as substrates was clearly related to the presence of a valine in position 425 which is closely located to the P450 pattern (Prosite pattern PS00086), a highly conserved region in cytochrome P450-dependent mono-oxygenases (Gotoh, 1992). However, chalcone 3-hydroxylation is only a side activity of the F3′H_var2 (Accession no. GQ281059) and additional, up to now unknown, structural features seem to be involved.
Apart from the CytP450 pattern, only a few domains are highly conserved in CytP450 enzymes, and the sequence identities of CytP450 enzymes are often very low. By sequence comparison of CytP450 enzymes Gotoh (1992) identified six regions, which could be involved in substrate recognition and binding, substrate recognition sites 1–6 (SRS1–6). Seitz et al. (2007) described in recent studies that three of the regions (SRS4–6) are highly conserved between amino acid sequences from several Asteraceae F3′Hs and F3′,5′Hs, whereas a high sequence variation in SRS1–3, which are located near the N-terminal end of the enzymes, is observed. Furthermore, it is assumed, that substrate specificities of F3′Hs and of flavonoid 3′,5′-hydroxylases are determined near their N-terminal ends (Seitz et al., 2007).
In this report, cloning and functional expression of a CH3H from Cosmos sulphureus, which shows F3′H activity as a side activity, is described for the first time. In order to identify regions which determine CH3H activity, alignment studies with F3′Hs from C. sulphureus and further Asteraceae species, which are not able to catalyse chalcone 3-hydroxylation (Schlangen et al., 2009, 2010) were performed. The impact of two candidate regions was studied with hybrid cDNAs and their corresponding recombinant hybrid proteins.
Materials and methods
Plant material
The investigations were performed on petals of C. sulphureus cv. Sunny Goldgelb (Austrosaat, Vienna, Austria). The plant material was collected during summer 2006 and summer 2007, frozen in liquid nitrogen and stored at –80 °C. Petals from closed buds with 5 mm length, open flowers with petals of 15–18 mm length, young leaves with 20 mm length, and fully developed leaves with 50 mm length were harvested. Seedlings were obtained by germinating seeds for 3 d that had been rolled in wet filter paper.
Chemicals
[14C]ISO was synthesized as described by Halbwirth et al. (2006) from 4-hydroxy[ring-U-14C]benzaldehyde (33.1 MBq mg−1) (Amersham International, UK). Synthesis of [14C]NAR, [14C]DHK, [14C]KAM, and [14C]API were performed according to Halbwirth and Stich (2006). NAR was synthesized from [2-14C]malonyl-coenzyme A (55 mCi mmol−1) (Amersham International, UK) with recombinant chalcone synthase (Schroeder, University of Freiburg, Germany) and recombinant chalcone isomerase. Synthesis of DHK, KAM, and API, was performed using recombinant flavanone 3-hydroxylase from Petunia hybrida, recombinant flavonol synthase from Arabidopsis thaliana, and microsomal enzyme preparations with high flavone synthase II activity from Dahlia variabilis cv. Katzelsdorf (Wirth, Vienna, Austria).
Cloning of Cosmos sulphureus CH3H
mRNA was extracted from C. sulphureus petals using the μMACS mRNA isolation kit (Miltenyi Biotec) and reverse transcription was performed with the RevertAid H Minus MuLV reverse transcriptase (Fermentas Life Science) with the oligo(dT) anchor primer GACCACGCGTATCGATGTCGAC(T)16V. RT-PCR was performed with the degenerated primers TGGMGDATGCTKMGGAARATYTG (forward primer) and GCCCATTCMAYNGTRCTAGATGA (reverse primer), which were designed from conserved regions from Asteraceae F3′H sequences deposited in GenBank: Rudbeckia hirta (Accession no. FJ216431), Echinops bannaticus (Accession no. FJ753549), Centaurea cyanus (Accession no. FJ753550), Gerbera hybrida (Accession no. ABA64468), Osteospermum hybrida (Accession no. ABB29899), Cichorium intybus (Accession no. FJ753548), and Callistephus chinensis (Accession no. AF313488) under the following cycling conditions: 2 min at 94 °C, 35× (30 s at 94 °C, 60 s at 60 °C, 1.5 min at 72 °C) and 7 min at 72 °C. The sequences of the amplified cDNA fragments were verified by a commercial supplier (MWG-Biotech AG, Ebersberg, Germany) and lacking 3′ and 5′-ends where generated via RACE techniques using the SMART™ Race cDNA amplification kit (Clontech, Takara Bio Company) according to the manufacturer's instructions. Final amplification of the entire ORF was carried out with the specific primers ATGACTATTCTACCCCTACTACTC (forward primer) and CCTTAATGACTTCCATACACGTG (reverse primer) which have been designed from obtained 3′- and 5′-fragments under following conditions: 2 min at 94 °C, 35× (30 s at 94 °C, 60 s at 60 °C, 2 min at 72 °C) and 7 min at 72 °C.
Sequence analysis and construction of hybrid genes
Pairwise and multiple sequence alignments were performed with ClustalW (Thompson et al., 1994, 2002) in order to identify candidate regions which could be involved in the determination of substrate specificity. The following F3′H sequences, mostly from Asteraceae species, were used for multiple sequence alignments: Antirrhinum majus (Accession no. DQ272592), Arabidopsis thaliana (Accession no. AF271651), Centaurea cyanus (Accession no. FJ753550), Cichorium intybus (Accession no. FJ753548), C. sulphureus (Accession no. FJ216426), Dahlia variabilis (Accession no. FJ216428), Echinops bannaticus (Accession no. FJ753549), Gerbera hybrida (Accession no. ABA64468), Osteospermum hybrida (Accession no. ABB29899), Rudbeckia hirta (Accession no. FJ216431) and Tagetes erecta (Accession no. FJ216430) (Schlangen et al., 2009). Pairwise sequence alignment was performed with the C. sulphureus F3′H (Accession no. FJ216426). Hybrid genes were constructed according to Seitz et al. (2007). In brief, the fragments were amplified with Pfu DNA polymerase (Promega, Mannheim, Germany) in separate PCR reactions and ligated for 10 min with T4 DNA ligase (Promega, Mannheim, Germany) in a total volume of 20 μl using 50 ng cDNA of each fragment. The diluted ligation was used as a template for a subsequent proofreading PCR, in which the whole hybrid gene was amplified with a Taq/Pwo polymerase system (Invitrogen, Paisley, UK).
Insertion or deletion of amino acid residues
Insertion of amino acid residues was achieved using primers with overhanging bases, coding for the respective amino acid residues which should be inserted. Deletion was done by amplification of two fragments of the respective F3′H cDNA lacking the bases, which code for the amino acid residues to be deleted. The amplified fragments were fused and the entire open reading frame (ORF) was amplified with proofreading PCR as described above for the construction of hybrid genes.
Site-directed mutagenesis
Site-directed mutagenesis was performed via Megaprimer-PCR (Barik, 1997). In a first PCR step, a megaprimer with the desired mutation using a plasmid with the insert cDNA to be mutated was amplified using Pfu DNA polymerase (Promega). For the amplification of the mutated megaprimer, an internal primer annealing in the region which should be mutated and consisting of defined altered bases and an external expression primer was used. This amplified mutated fragment was used for the second PCR step as primer together with a corresponding expression primer, in order to amplify the entire ORF carrying the desired mutation(s), using the ‘Expand High Fidelity PCR System’ (Roche).
Heterologous expression in yeast
For heterologous expression, proofreading cDNA amplicons were ligated into the yeast expression vector pYES2.1/V5-His-TOPO® (Invitrogen, Paisley, UK) and transformed into E. coli TOP10F' (Invitrogen, Paisley, UK). Identified sense constructs were isolated and confirmed by commercial sequencing (Eurofins MWG Operon, Ebersberg, Germany). Plasmids were transformed into the yeast strain INVSc 1 (Invitrogen, Paisley, UK). Heterologous expression was performed according to Pompon et al. (1996) and recombinant proteins were shock frozen in liquid nitrogen and stored at –80 °C. The protein content was quantified by a modified Lowry procedure (Sandermann and Strominger, 1972) with crystalline BSA as standard.
Enzyme assays and product identification
The reaction mixture in a final volume of 100 μl contained: 1 (CH3H) –20 (F3′H) μg of the recombinant wild type protein and 50 μg of the hybrid proteins, 0.25 nmol of [14C]-labelled substrate, 5 μl 10 mM NADPH, and 0.1 M KH2PO4-K2HPO4 buffer (containing 0.4% Na-ascorbate, pH 7.5). After incubation at 30 °C for 30 min, the enzyme reactions were stopped with 10 μl glacial acetic acid, and the phenolic compounds were extracted twice with EtOAc. The organic phases were transferred to a precoated cellulose plate (Merck, Germany) and chromatographed in CAW (chloroform:acetic acid:water, 10:9:1 by vol.). Detection and quantification of radioactivity was performed using a TLC analyser (Berthold, Wildbad, Germany).
Kinetic data
Kinetic data were calculated from Lineweaver–Burk plots. Apparent Michaelis constant (Km) and maximum reaction velocity (Vmax) for the different substrates were obtained using the Hyperbolic regression analysis software HYPER 32 (J.S. Easterby, University of Liverpool, UK).
Quantitative PCR assays (qPCR)
The qPCR assays were performed in 20 μl reactions with SYBR® GREEN PCR Master Mix (Applied Biosystems) with 0.375 μM of each primer using the ‘Step One Plus Real Time PCR System’ (Applied Biosystems). The reaction was run for 40 cycles at 94 °C for 1 min and 59 °C for 2 min. Following melt curve analysis was performed in order to ensure the amplification of one single same-sized fragment. Quadruplicate qPCR assays were performed for each sample. Standards for each transcript were generated with serially diluted plasmids carrying the cDNA fragments to be determined, respectively, as inserts. The straight line equation obtained by plotting the cycle threshold (Ct) value of the standards against their dilutions was used to determine the abundance of the qPCR product from the Ct value of each sample. All primers used for the qPCR are listed in Table 2.
Results
Cloning, sequence analysis, and functional activity of the recombinant CH3H
In this work, a novel cDNA from C. sulphureus was isolated using degenerated primers from several Asteraceae F3′H sequences (Accession nos FJ216431, FJ753549, FJ753550, ABA64468, ABB29899, FJ753548, and AF313488). The deduced amino acid sequence of the full-size clone showed all conserved motifs found in membrane bound P450s, for example, the N-terminal hydrophobic membrane anchor and the highly conserved heme domain (Fig. 2). The sequence of the cDNA clone was deposited in the EMBL/GenBank data libraries under Accession no. FJ216429. The deduced amino acid sequence of the isolated cDNA from C. sulphureus shared high identity with sequences of F3′Hs which do not accept chalcones as substrates (Schlangen et al., 2009). However, the amino acid sequence was different than the previously published F3′H from C. sulphureus (Accession no. FJ216426) and shared only 84% amino acid sequence identity (Fig. 2).
![Pairwise alignment of CH3H and F3′H amino acid sequences from C. sulphureus (Accession nos FJ216429 and FJ216426). Alignment was performed with ClustalW (Thompson et al., 1994, 2002). Grey-shaded boxes highlight substrate recognition site 1 (SRS1: Gotoh, 1992) and conserved P450 patterns. I, Hydrophobic membrane anchor; II, proline-rich hinge [PI]-P-G-P-X-[GP]-P (Kemper, 2004); III, oxygen-binding motif [AG]-G-X-[ED]-T-[TS]; IV, EXXR triad (Ravichandran et al., 1993); V, the P450-pattern [FW]-[SNGH]-X-[GD]-{F}-[RKHPT]-{P}-C-[LIVMFAP]-[GAD] (Prosite pattern PS00086; Hulo et al., 2006).](https://oup.silverchair-cdn.com/oup/backfile/Content_public/Journal/jxb/61/12/10.1093_jxb_erq169/1/m_jexboterq169f02_ht.gif?Expires=1750238237&Signature=LiBGXC1RiwkpiTkFaYj5Bd~rqfXok0W0xNMb87d7LGcd0iNQTrkX9~27AZegydPovv-EUYO~kp7PWKCzIuQ2iC3t7p3u~it8sYOD5qGvYkDWkq5Kai7wYbYxhHpX~ZvRBR6-37I1uxKB~CPMghgMfhXCGPdIIeoBD5r~-Ic1PCVTl~L9J9ZJO-S3t8MrHsiLHh0hQEYnpt0V2VeiDgtDZK5HqkhBaNeqI-209sERbvCblq5drKWmv-4Sb-~9-vS9RYqolpqKFzKjkfQRyfXFseA5JdcwiVSet24O79dHrIafwroV5si908ZuWp8ro8PFBAbcnr3NkYVmZCsMFcyn2g__&Key-Pair-Id=APKAIE5G5CRDK6RD3PGA)
Pairwise alignment of CH3H and F3′H amino acid sequences from C. sulphureus (Accession nos FJ216429 and FJ216426). Alignment was performed with ClustalW (Thompson et al., 1994, 2002). Grey-shaded boxes highlight substrate recognition site 1 (SRS1: Gotoh, 1992) and conserved P450 patterns. I, Hydrophobic membrane anchor; II, proline-rich hinge [PI]-P-G-P-X-[GP]-P (Kemper, 2004); III, oxygen-binding motif [AG]-G-X-[ED]-T-[TS]; IV, EXXR triad (Ravichandran et al., 1993); V, the P450-pattern [FW]-[SNGH]-X-[GD]-{F}-[RKHPT]-{P}-C-[LIVMFAP]-[GAD] (Prosite pattern PS00086; Hulo et al., 2006).
The functional activity of the enzyme was studied with the recombinant protein. Isoliquiritigenin (ISO) was completely converted to butein when incubated with the recombinant enzyme in the presence of NADPH. By contrast, only a low conversion rate of naringenin (NAR), dihydrokaempferol (DHK), and kaempferol (KAM) was observed under the same conditions (Table 1). Higher conversion rates with flavonoids substrates could be achieved only when enzyme concentration and/or incubation time was increased above the linearity range of ISO conversion. The kinetic data confirmed ISO being the preferred substrate with the highest Vmax/Km ratio (18 l s−1 kg−1) and a low Km value (4 μM) compared with the majority of the flavonoid substrates used. Therefore, the protein from C. sulphureus was named chalcone 3-hydroxylase (CH3H). For comparison, recombinant F3′H from C. sulphureus (Accession no. FJ216426) was tested with the same substrates (Table 1). These tests confirmed the F3′H activity and the absent CH3H activity of this enzyme as previously described by Schlangen et al. (2009). Buds showed the highest expression of both CH3H and F3′H compared with all other tissues investigated. However, the expression profiles of CH3H and F3′H were comparable only in buds, seedlings, and flowers, whereas particularly in leaf tissues F3′H expression drastically dominated over CH3H expression (Fig. 3).
pH-optima, conversion rates and kinetic values of the recombinant CH3H and F3′H from C. sulphureus
Substrate | ||||
Isoliquiritgenin | Naringenin | Dihydrokaempferol | Kaempferol | |
Recombinant CH3H | ||||
Conversion rate | 72.5 | 11.6 | 10.2 | 13.7 |
pH | 7.25 | 7.25 | 7.00 | 7.75 |
Km [μM] | 4.0 | 40.0 | 47.0 | 1.3 |
Vmax (μmol s−1 kg−1) | 71.5 | 150.0 | 170.0 | 16.0 |
Vmax/Km (l s−1 kg−1) | 18.0 | 3.7 | 3.6 | 11.9 |
Recombinant F3′H | ||||
Conversion rate | – | 29.3 | 45.1 | 35.6 |
pH | 8.25 | 7.25 | 8.5 | |
Km [μM] | 2.1 | 2.7 | 0.7 | |
Vmax (μmol s−1 kg−1) | 2.7 | 1.9 | 1.4 | |
Vmax/Km (l s−1 kg−1) | 1.3 | 0.7 | 2.0 |
Substrate | ||||
Isoliquiritgenin | Naringenin | Dihydrokaempferol | Kaempferol | |
Recombinant CH3H | ||||
Conversion rate | 72.5 | 11.6 | 10.2 | 13.7 |
pH | 7.25 | 7.25 | 7.00 | 7.75 |
Km [μM] | 4.0 | 40.0 | 47.0 | 1.3 |
Vmax (μmol s−1 kg−1) | 71.5 | 150.0 | 170.0 | 16.0 |
Vmax/Km (l s−1 kg−1) | 18.0 | 3.7 | 3.6 | 11.9 |
Recombinant F3′H | ||||
Conversion rate | – | 29.3 | 45.1 | 35.6 |
pH | 8.25 | 7.25 | 8.5 | |
Km [μM] | 2.1 | 2.7 | 0.7 | |
Vmax (μmol s−1 kg−1) | 2.7 | 1.9 | 1.4 | |
Vmax/Km (l s−1 kg−1) | 1.3 | 0.7 | 2.0 |
pH-optima, conversion rates and kinetic values of the recombinant CH3H and F3′H from C. sulphureus
Substrate | ||||
Isoliquiritgenin | Naringenin | Dihydrokaempferol | Kaempferol | |
Recombinant CH3H | ||||
Conversion rate | 72.5 | 11.6 | 10.2 | 13.7 |
pH | 7.25 | 7.25 | 7.00 | 7.75 |
Km [μM] | 4.0 | 40.0 | 47.0 | 1.3 |
Vmax (μmol s−1 kg−1) | 71.5 | 150.0 | 170.0 | 16.0 |
Vmax/Km (l s−1 kg−1) | 18.0 | 3.7 | 3.6 | 11.9 |
Recombinant F3′H | ||||
Conversion rate | – | 29.3 | 45.1 | 35.6 |
pH | 8.25 | 7.25 | 8.5 | |
Km [μM] | 2.1 | 2.7 | 0.7 | |
Vmax (μmol s−1 kg−1) | 2.7 | 1.9 | 1.4 | |
Vmax/Km (l s−1 kg−1) | 1.3 | 0.7 | 2.0 |
Substrate | ||||
Isoliquiritgenin | Naringenin | Dihydrokaempferol | Kaempferol | |
Recombinant CH3H | ||||
Conversion rate | 72.5 | 11.6 | 10.2 | 13.7 |
pH | 7.25 | 7.25 | 7.00 | 7.75 |
Km [μM] | 4.0 | 40.0 | 47.0 | 1.3 |
Vmax (μmol s−1 kg−1) | 71.5 | 150.0 | 170.0 | 16.0 |
Vmax/Km (l s−1 kg−1) | 18.0 | 3.7 | 3.6 | 11.9 |
Recombinant F3′H | ||||
Conversion rate | – | 29.3 | 45.1 | 35.6 |
pH | 8.25 | 7.25 | 8.5 | |
Km [μM] | 2.1 | 2.7 | 0.7 | |
Vmax (μmol s−1 kg−1) | 2.7 | 1.9 | 1.4 | |
Vmax/Km (l s−1 kg−1) | 1.3 | 0.7 | 2.0 |
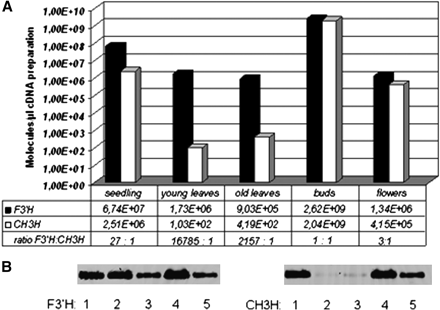
Gene expression profiles of F3′H and CH3H in five different Cosmos tissues: seedlings (1), young leaves (2), old leaves (3), buds (4), and flowers (5). (A) Displayed via a logarithmic y-axis. Transcript levels were quantified using plasmids harbouring a copy of the respective cDNA clone. The number of F3′H and CH3H transcripts and the ratio F3′H:CH3H are also shown. (B) qPCR assays run in 1.5% agarose gel, left, F3′H; right, CH3H.
Identification of regions putatively involved in chalcone 3-hydroxylation
The pairwise alignment of CH3H and F3′H from C. sulphureus showed an insertion of four amino acid residues in the CH3H sequence compared with the F3′H (SAGG-region, Fig. 2) with divergent adjacent residues (XSAGGXX-region; Fig. 2). Multiple alignment with additional F3′H sequences confirmed that the region D(192)GSAGGDP(199) is only present in the CH3H amino acid sequence (Fig. 4). Homology modelling studies showed that the XSAGGXX-region putatively is a loop region (Fig. 5). F3′Hs with a proven lack of CH3H activity (Schlangen et al., 2009) had no or only a modified insertion. Thus, F3′Hs from Centaurea cyanus, Cichorium intybus, and Echinops bannaticus showed an insertion of D(192)GSGGGD(198) instead. In addition, CH3H showed differences in a region, which was predicted as a substrate recognition site (SRS1) in CytP450 enzymes (Gotoh, 1992). These regions were putatively involved in the chalcone acceptance of the CH3H enzyme.
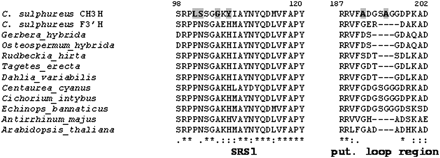
Alignment of selected regions of the CH3H and F3′H amino acid sequences. Bold letters on a grey background indicate amino acid residues only present in CH3H.
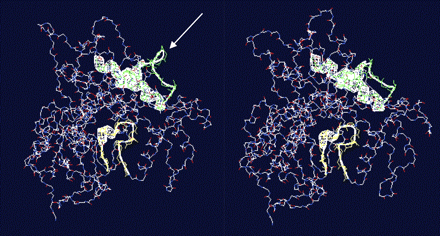
Visualization of the homology modelled enzymes CH3H (left) and F3′H (right) using swissmodel. The white arrow marks the putative loop region at the CH3H surface.
Five hybrid genes containing fragments of C. sulphureus CH3H and F3′H were constructed to investigate the impact of the regions putatively involved in chalcone 3-hydroxylation. An overview of the primers used is listed in Table 2 and a schematic illustration of the corresponding hybrid proteins (C1–C5) is given in Table 3. C1 consists of an N-terminal part (amino acid residues 1–196) of F3′H and a C-terminal part (corresponding amino acid residues 201–512) of CH3H. C2–C4 were constructed from the N-terminal parts of CH3H and the C-terminal parts of F3′H with varying lengths. In the case of C2, the N-terminal part of CH3H coding for the first 210 amino acid residues included its SRS1 and the putative loop region, whereas in C3 and C4 the SRS1 from CH3H, but not the putative loop region were present (C3: amino acid residues 1–119; C4: amino acid residues 1–193 CH3H). C5, by contrast, is a combination of the N-terminal part of the CH3H with its SRS1 (amino acid residues 1–119) and the C-terminal part of F3′H in which the putative loop region of CH3H (amino acid residues 194–197) was inserted (Table 2). Amino acid positions are based on the pairwise alignment of C. sulphureus F3′H and CH3H. All recombinant hybrid proteins showed functional activity (Table 2), but conversion rates were generally lower compared with the recombinant parent proteins as frequently reported (Schalk and Croteau, 2000). Therefore, higher protein amounts had to be used for testing to obtain the conversion rates shown in Table 3. Hydroxylation of chalcones was observed only with the hybrid genes C1, C2, and C5. The recombinant enzyme C1, however, showed only a low CH3H activity compared with its F3′H activity and to the CH3H activities of the other recombinant enzymes.
cDNA clone | Template | Manipulation | Forward primer | Reverse primer |
Hybrid genes | ORF region | |||
C1 | 5′-fragment: F3′H | 1–196 | ATGACTATTCTACCCCTACTACTC | CTTCGCATCACGCTCACCG |
3′-fragment: CH3H | 197–508 | P-GCGGATGAGTTCAAGGATATGG | CCTTAATGACTTCCATACACGTG | |
C2 | 5′-fragment: CH3H | 1–210 | ATGACTATTCTACCCCTACTACTC | CTTCGGATCACCACCTGCA |
3′-fragment: F3'H | 211–512 | P-GCGGATGAGTTCAAG GAT ATG G | AGGATGTCTCAACTTACAACAATAAACC | |
C3 | 5′-fragment: CH3H | 1–119 | ATGACTATTCTACCCCTACTACTC | GTACGGTGCAAACACCATATC |
3′-fragment: F3′H | 120–508 | P- GGTCCTCGGTGGCGGAT | AGGATGTCTCAACTTACAACAATAAACC | |
C4 | 5′-fragment: CH3H | 1–193 | ATGACTATTCTACCCCTACTACTC | P- TTCGCATCTCCGTCGGC |
3′-fragment: F3′H | 194–508 | GGCGGATGAGTTCAAGGATATG | AGGATGTCTCAACTTACAACAATAAACC | |
C5 | 5′-fragment: CH3H | 1–119 | ATGACTATTCTACCCCTACTACTC | GTACGGTGCAAACACCATATC |
3′-fragment: M2 | 120–512 | P- GGTCCTCGGTGGCGGAT | AGGATGTCTCAACTTACAACAATAAACC | |
Mutated genes | Mutation | |||
M1 | 5′-fragment: CH3H | Deletion of S(194)AAG(197) | ATGACTATTCTACCCCTACTACTC | TCCGTCGGCGAATACTCTC |
3′-fragment: CH3H | P-GATCCGAAGGCGGATGAGTT | CCTTAATGACTTCCATACACGTG | ||
M2 | 5′-fragment F3′H | Insertion of S(194)AAG(197), exchange of R(193) into G and A(199) into P | ATGACTATTCTACCCCTACTACTC | CACCTGCACTTCCGTCACCGAATACTCTCCTGCC (SAGG from CH3H/F3′H) |
3′-fragment: F3′H | P-GTGATGCGAAGGCGGATGAG | AGGATGTCTCAACTTACAACAATAAACC | ||
M3 | CH3H | CCG -> GCG P(199) -> A | ATCCGCCTTCGCATCACCA |
cDNA clone | Template | Manipulation | Forward primer | Reverse primer |
Hybrid genes | ORF region | |||
C1 | 5′-fragment: F3′H | 1–196 | ATGACTATTCTACCCCTACTACTC | CTTCGCATCACGCTCACCG |
3′-fragment: CH3H | 197–508 | P-GCGGATGAGTTCAAGGATATGG | CCTTAATGACTTCCATACACGTG | |
C2 | 5′-fragment: CH3H | 1–210 | ATGACTATTCTACCCCTACTACTC | CTTCGGATCACCACCTGCA |
3′-fragment: F3'H | 211–512 | P-GCGGATGAGTTCAAG GAT ATG G | AGGATGTCTCAACTTACAACAATAAACC | |
C3 | 5′-fragment: CH3H | 1–119 | ATGACTATTCTACCCCTACTACTC | GTACGGTGCAAACACCATATC |
3′-fragment: F3′H | 120–508 | P- GGTCCTCGGTGGCGGAT | AGGATGTCTCAACTTACAACAATAAACC | |
C4 | 5′-fragment: CH3H | 1–193 | ATGACTATTCTACCCCTACTACTC | P- TTCGCATCTCCGTCGGC |
3′-fragment: F3′H | 194–508 | GGCGGATGAGTTCAAGGATATG | AGGATGTCTCAACTTACAACAATAAACC | |
C5 | 5′-fragment: CH3H | 1–119 | ATGACTATTCTACCCCTACTACTC | GTACGGTGCAAACACCATATC |
3′-fragment: M2 | 120–512 | P- GGTCCTCGGTGGCGGAT | AGGATGTCTCAACTTACAACAATAAACC | |
Mutated genes | Mutation | |||
M1 | 5′-fragment: CH3H | Deletion of S(194)AAG(197) | ATGACTATTCTACCCCTACTACTC | TCCGTCGGCGAATACTCTC |
3′-fragment: CH3H | P-GATCCGAAGGCGGATGAGTT | CCTTAATGACTTCCATACACGTG | ||
M2 | 5′-fragment F3′H | Insertion of S(194)AAG(197), exchange of R(193) into G and A(199) into P | ATGACTATTCTACCCCTACTACTC | CACCTGCACTTCCGTCACCGAATACTCTCCTGCC (SAGG from CH3H/F3′H) |
3′-fragment: F3′H | P-GTGATGCGAAGGCGGATGAG | AGGATGTCTCAACTTACAACAATAAACC | ||
M3 | CH3H | CCG -> GCG P(199) -> A | ATCCGCCTTCGCATCACCA |
cDNA clone | Template | Manipulation | Forward primer | Reverse primer |
Hybrid genes | ORF region | |||
C1 | 5′-fragment: F3′H | 1–196 | ATGACTATTCTACCCCTACTACTC | CTTCGCATCACGCTCACCG |
3′-fragment: CH3H | 197–508 | P-GCGGATGAGTTCAAGGATATGG | CCTTAATGACTTCCATACACGTG | |
C2 | 5′-fragment: CH3H | 1–210 | ATGACTATTCTACCCCTACTACTC | CTTCGGATCACCACCTGCA |
3′-fragment: F3'H | 211–512 | P-GCGGATGAGTTCAAG GAT ATG G | AGGATGTCTCAACTTACAACAATAAACC | |
C3 | 5′-fragment: CH3H | 1–119 | ATGACTATTCTACCCCTACTACTC | GTACGGTGCAAACACCATATC |
3′-fragment: F3′H | 120–508 | P- GGTCCTCGGTGGCGGAT | AGGATGTCTCAACTTACAACAATAAACC | |
C4 | 5′-fragment: CH3H | 1–193 | ATGACTATTCTACCCCTACTACTC | P- TTCGCATCTCCGTCGGC |
3′-fragment: F3′H | 194–508 | GGCGGATGAGTTCAAGGATATG | AGGATGTCTCAACTTACAACAATAAACC | |
C5 | 5′-fragment: CH3H | 1–119 | ATGACTATTCTACCCCTACTACTC | GTACGGTGCAAACACCATATC |
3′-fragment: M2 | 120–512 | P- GGTCCTCGGTGGCGGAT | AGGATGTCTCAACTTACAACAATAAACC | |
Mutated genes | Mutation | |||
M1 | 5′-fragment: CH3H | Deletion of S(194)AAG(197) | ATGACTATTCTACCCCTACTACTC | TCCGTCGGCGAATACTCTC |
3′-fragment: CH3H | P-GATCCGAAGGCGGATGAGTT | CCTTAATGACTTCCATACACGTG | ||
M2 | 5′-fragment F3′H | Insertion of S(194)AAG(197), exchange of R(193) into G and A(199) into P | ATGACTATTCTACCCCTACTACTC | CACCTGCACTTCCGTCACCGAATACTCTCCTGCC (SAGG from CH3H/F3′H) |
3′-fragment: F3′H | P-GTGATGCGAAGGCGGATGAG | AGGATGTCTCAACTTACAACAATAAACC | ||
M3 | CH3H | CCG -> GCG P(199) -> A | ATCCGCCTTCGCATCACCA |
cDNA clone | Template | Manipulation | Forward primer | Reverse primer |
Hybrid genes | ORF region | |||
C1 | 5′-fragment: F3′H | 1–196 | ATGACTATTCTACCCCTACTACTC | CTTCGCATCACGCTCACCG |
3′-fragment: CH3H | 197–508 | P-GCGGATGAGTTCAAGGATATGG | CCTTAATGACTTCCATACACGTG | |
C2 | 5′-fragment: CH3H | 1–210 | ATGACTATTCTACCCCTACTACTC | CTTCGGATCACCACCTGCA |
3′-fragment: F3'H | 211–512 | P-GCGGATGAGTTCAAG GAT ATG G | AGGATGTCTCAACTTACAACAATAAACC | |
C3 | 5′-fragment: CH3H | 1–119 | ATGACTATTCTACCCCTACTACTC | GTACGGTGCAAACACCATATC |
3′-fragment: F3′H | 120–508 | P- GGTCCTCGGTGGCGGAT | AGGATGTCTCAACTTACAACAATAAACC | |
C4 | 5′-fragment: CH3H | 1–193 | ATGACTATTCTACCCCTACTACTC | P- TTCGCATCTCCGTCGGC |
3′-fragment: F3′H | 194–508 | GGCGGATGAGTTCAAGGATATG | AGGATGTCTCAACTTACAACAATAAACC | |
C5 | 5′-fragment: CH3H | 1–119 | ATGACTATTCTACCCCTACTACTC | GTACGGTGCAAACACCATATC |
3′-fragment: M2 | 120–512 | P- GGTCCTCGGTGGCGGAT | AGGATGTCTCAACTTACAACAATAAACC | |
Mutated genes | Mutation | |||
M1 | 5′-fragment: CH3H | Deletion of S(194)AAG(197) | ATGACTATTCTACCCCTACTACTC | TCCGTCGGCGAATACTCTC |
3′-fragment: CH3H | P-GATCCGAAGGCGGATGAGTT | CCTTAATGACTTCCATACACGTG | ||
M2 | 5′-fragment F3′H | Insertion of S(194)AAG(197), exchange of R(193) into G and A(199) into P | ATGACTATTCTACCCCTACTACTC | CACCTGCACTTCCGTCACCGAATACTCTCCTGCC (SAGG from CH3H/F3′H) |
3′-fragment: F3′H | P-GTGATGCGAAGGCGGATGAG | AGGATGTCTCAACTTACAACAATAAACC | ||
M3 | CH3H | CCG -> GCG P(199) -> A | ATCCGCCTTCGCATCACCA |
Schematic illustration of generation of hybrid (C1–C5) and mutated (M1–M3) proteins and resulting conversion rates of the recombinant enzymes
![]() |
![]() |
CH3H derived sequences are shown as black bars, F3′H derived sequences in grey. The results are based on at least three independent heterologous expression experiments. Please note that different protein amounts were used for parent proteins (Table 1) and modified proteins in this table.
Schematic illustration of generation of hybrid (C1–C5) and mutated (M1–M3) proteins and resulting conversion rates of the recombinant enzymes
![]() |
![]() |
CH3H derived sequences are shown as black bars, F3′H derived sequences in grey. The results are based on at least three independent heterologous expression experiments. Please note that different protein amounts were used for parent proteins (Table 1) and modified proteins in this table.
In order to evaluate if the XSAGGXX-region in C. sulphureus CH3H alone is responsible for the ability of chalcone hydroxylation, the nucleotides coding for the four additional amino acid residues SAGG were deleted from the CH3H (Tables 2, 3; M1). By contrast, the XSAGGXX-region (Fig. 2) from CH3H was introduced into the F3′H sequence and the neighbour residues were substituted as follows: R193G and A199P (Tables 2, 3; M2). In a third mutation (M3), P199 from the XSAGGXX-region was changed to A199 because an alanine is present at this position in the C. sulphureus F3′H sequence (Fig. 2). Primers used for the amplification of the three mutated cDNAs (M1–M3) are listed in Table 3. Substrate acceptance studies with the recombinant proteins showed that the deletion of the SAGG-region from CH3H did not significantly change its ability to hydroxylate chalcones in position 3 (Table 2). Likewise, the introduction of the XSAGGXX-region in F3′H did not result in CH3H activity. The recombinant M3 protein, however, did not show any functional activity.
Discussion
Up to now, chalcone 3-hydroxylation was observed in only one case—as a side activity of a special F3′H (Schlangen et al., 2010)—and the molecular basis for chalcone 3-hydroxylation remained unclear. In this work, a specific CH3H from C. sulphureus was identified for the first time, which is primarily expressed in buds. In leaves, almost no CH3H expression could be observed, and in open flowers and seedlings only to a lower extent. The data in Fig. 2 are shown via a logarithmic y-axis to allow the simultaneous visibility of the expression profiles in all tissues despite the high expression rates in buds. However, this should not hide the fact that CH3H expression in buds is increased by a factor of E+03 compared with seedlings and by a factor of E+07 compared to leaves. F3′H expression by contrast was highest in buds, but showed comparable rates in all other tissues. This reflects the requirement for flavonoid 3′-hydroxylation in many different plant tissues, where flavonoids fulfil important functions, which are frequently influenced by the B-ring hydroxylation pattern (Harborne and Williams, 2000; Halbwirth, 2010). Particularly in leaves, the F3′H expression was increased by a factor of E+04 compared with CH3H expression. The specific CH3H expression in the main tissues relevant for the synthesis of 6′-deoxychalcone based pigments and the specificity of the recombinant CH3H for the 6′-deoxychalcone ISO, which is the native chalcone substrate in C. sulphureus supports the thesis that the novel enzyme is responsible for chalcone 3-hydroxylation in planta. However, the physiological relevance of CH3H expression in seedlings remains open.
The F3′H from C. sulphureus (Accession no. FJ216426) was not able to catalyse the hydroxylation of chalcones in the B-ring (Schlangen et al., 2009), despite sharing 84% amino acid sequence identity with the novel CH3H. Sequence alignments of both enzymes from C. sulphureus and further F3′Hs, which are not able to convert chalcones (Schlangen et al., 2009) identified two CH3H regions which are highly heterogeneous in contrast to the conserved ones found in F3′H sequences. In a region involved in substrate recognition according to Gotoh (1992)—the so-called substrate recognition site 1 (SRS1)—amino acid residues of CH3H were clearly different from those of F3′H sequences (Figs 2, 4). Thus, this was one of the regions putatively involved in chalcone acceptance. The second candidate region was composed of four additional amino acid residues, which was present in the CH3H sequence at positions 193–196 but not in the F3′H sequence from Cosmos (Fig. 2). The adjacent amino acids also showed some differences. In order to assess the impact of these regions on the CH3H activity, hybrid proteins were constructed consisting of F3′H and CH3H fragments from C. sulphureus. In addition, the regions of interest were mutated to investigate the resulting changes in substrate acceptance.
The functional activity studies with the hybrid proteins demonstrated that CH3H activity is primarily determined in the N-terminal region. Replacement of the N-terminal part of F3′H with the corresponding CH3H sequence resulted in a high CH3H activity of the hybrid protein C2, although the major part was derived from F3′H. In the same way, a dramatic loss of CH3H activity was observed, when the N-terminal part of CH3H was replaced by the N-terminal part of F3′H, although the major part of the hybrid protein C1 derived from the CH3H. However, in contrast to the F3′H, a low CH3H activity was still observed indicating that the remaining CH3H sequence contributes to the substrate specificity although only to a slight extent.
The presence of CH3H SRS1 alone did not determine CH3H activity as demonstrated by the hybrid proteins C3 and C4, which did not accept chalcones as substrates (Table 3). In the same way, the introduction of the XSAGGXX-region into the F3′H sequence (M1) did not result in CH3H activity, and removal of the XSAGGXX-region did not convert CH3H into a common F3′H either. CH3H activity was primarily dependent on the presence of both the SRS1 of CH3H and the XSAGGXX-region because only the hybrid proteins C2 and C5, which contained both regions, were able to catalyse chalcone 3-hydroxylation to a considerable extent.
Homology modelling studies with C. sulphureus F3′H and CH3H enzymes indicate that the XSAGGXX-region is a part of a loop region at the molecule surface (Fig. 5). Unfortunately, the computed F3′H and CH3H models are not conclusive because they are based on a template which only shares 27% identity. A more appropriate template for the modelling process, however, is not available to date. The high number of gaps and of glycine and proline residues in this region (Fig. 2) support the model's suggestion, because these are indicators for loop regions. Proline frequently acts as a structural disruptor of α helices and β sheets and is, therefore, often found in loops or turns and at the end of α helices. Several studies have demonstrated that loop regions can be of importance for substrate binding (Tamura et al., 1995; Smilda et al., 1998; Fuller-Schaefer and Kadner, 2005). Therefore, it is possible that the putative loop region influences the substrate binding property of the C. sulphureus CH3H.
The XSAGGXX-region seems to have an impact on enzyme activity in general. Introduction of the XSAGGXX region into C. sulphureus F3′H noticeably increased the catalytic activity of C. sulphureus F3′H (M2). Chalcone 3-hydroxylation, however, could not be observed (Fig. 3). Substitution of P199A from the XSAGGXX-region in CH3H led to the loss of general enzyme activity (M3). This supports the hypothesis that the proline residue could have an influence on protein folding and structural arrangement in the CH3H enzyme. Thus, the proline residue, combined with the four additional amino acid residues, could therefore have, in combination with the specific CH3H SRS1 region, an influence on the substrate specificity of the C. sulphureus.
The fact that common F3′Hs do not show CH3H activity but CH3H shows remaining F3′H activity suggests that CH3H has evolved from F3′H. This is supported by the presence of an F3′H with CH3H side activity (Accession no. GQ281059) in Dahlia variabilis, which shows divergent chalcone acceptance compared with its allelic F3′H variant (Accession no. GQ281058) (Schlangen et al., 2010). However, comparison of the kinetic data obtained with chalcone and flavonoid substrates clearly distinguishes the novel CH3H from the F3′H with CH3H side activity. Evolution of a CH3H from F3′H could have been a consequence of gene duplication following the evolution of two different genes coding for proteins with different catalytic activities as discussed for other proteins (Des Marais and Rauscher, 2008). With regard to substrate specificity, F3′Hs with CH3H side activity could represent an intermediate step in the evolution of CH3H.
Summarizing, our studies identified the first CH3H cDNA from any plant species. Although the specificity for chalcones is primarily determined in the N-terminal part of the enzyme, which contains a CH3H specific substrate recognition site and a loop region located at the surface of the molecule, a full understanding of all CH3H specific elements will depend on the availability of further CH3H cDNA clones or a suitable template for homology modelling studies. The novel cDNA clone completes the set of genes involved in the formation of anthochlor pigments, which includes chalcone synthase, aureusidine synthase, chalcone reductase and a few glucosyltransferases (Forkmann and Heller, 1999). This will allow the formation of anthochlor pigment-based UV-honey guides to be studied in Asteraceae species and could also support current molecular breeding approaches for yellow flower colour (Davies et al., 2001). In addition, the enzyme could be of broader relevance because it influences the hydroxylation pattern of chalcones which are formed in the majority of plants. As observed for polyphenols in general, the hydroxylation pattern may have a strong impact on the physiological function in the plant and also on human health (Halbwirth, 2010).
These investigations were supported by a grant from the Austrian Science Fund (FWF): [Project V18-B03]. J Thill acknowledges her Marie Curie scholarship from EU (MRTN_CT-2006-035805).
Comments