-
PDF
- Split View
-
Views
-
Cite
Cite
Yu Xing, Wensuo Jia, Jianhua Zhang, AtMEK1 mediates stress-induced gene expression of CAT1 catalase by triggering H2O2 production in Arabidopsis, Journal of Experimental Botany, Volume 58, Issue 11, August 2007, Pages 2969–2981, https://doi.org/10.1093/jxb/erm144
- Share Icon Share
Abstract
Catalase and hydrogen peroxide (H2O2) have been extensively studied for their roles in various stress responses. However, little is known about the triggering mechanisms for stress-induced catalase gene expression or about H2O2 production as a stress signal. It is reported here that ABA-, drought-, and salt stress-induced gene expression of CAT1 catalase is mediated by AtMEK1, an Arabidopsis MAPK kinase, by triggering H2O2 signal production. Both CAT1 expression and AtMEK1 activity were activated by ABA, drought, and salt stresses. The mek1 mutant totally blocked stress-induced CAT1 expression and, interestingly, stress-induced H2O2 production was also blocked. Over-expression of AtMEK1 significantly promoted stress-induced CAT1 expression, and also promoted H2O2 production. These results conclusively indicate that stress-induced CAT1 expression is mediated by AtMEK1 and, furthermore, that the triggering of H2O2 production might be involved in this process, as further proved by the observation that CAT1 expression was induced by applied H2O2. Surprisingly, the signalling mechanisms for stress-induced gene expression of CAT2 and CAT3 were very different from that of CAT1. Except for drought stress, expression of CAT2 or CAT3 was also activated by salt stress or ABA treatment, and AtMEK1 was not proved to be involved in the drought-induced expression of CAT2 or CAT3. Further studies showed that stomatal movement was much less sensitive to ABA in AtMEK1 mutant (mek1), and over-expression of AtMEK1 in Arabidopsis increased plant resistance to drought or salt stress, which further demonstrated that AtMEK1 is a crucial mediator in plant stress signal transduction.
Introduction
Reactive oxygen species (ROS) have long been known to be deleterious to cellular activities, but their transient increase in cells has increasingly been suggested to act as signals and to mediate the regulation of various cellular activities, such as responses to biotic or abiotic stresses, cell death, stomatal movement, and root hair development (Adler et al., 1995, 1999; Mount, 1996; Lander, 1997; Dalton et al., 1999; Kamata and Hirata, 1999; Rhee, 1999; Guan et al., 2000). Among different ROS, only hydrogen peroxide (H2O2) can cross plant membranes and it has been increasingly proved to be one of the most important signals in plant cell signalling, especially in elicitor-induced defence responses (Neill et al., 2002; Vanderauwera et al., 2005; Pitzschke and Hirt, 2006; Gadjev et al., 2006).
Catalase (CAT) is the predominant enzyme controlling the H2O2 level. In Arabidopsis, catalases are encoded by a small multigene family consisting of CAT1, CAT2, and CAT3 (Frugoli et al., 1996, 1998; Zentgraf and Zinkernagel, 1996). While catalases have been extensively studied for many years, growing evidence suggests that catalase and other antioxidative enzymes are closely related to plant biotic or abiotic stress-tolerance, although the signalling processes of stress-induced gene expression of catalase are largely unknown (Beyer and Fridovich, 1987; Dempsey and Klessig, 1994; Foyer et al., 1994; Pinhero et al., 1997; Holmberg and Bulow, 1998; Foyer and Noctor, 2000).
Acting as one of the major signalling cascades, mitogen-activated protein kinase (MAPK) modules mediate a wide range of cellular responses to extracellular stimuli in plant cells (Hirt, 1997; Ligterink et al., 1997; Mizoguchi et al., 1997; Hirt and Asard, 2000; Nakagami et al., 2005). Several lines of evidence have indicated that the MAPK signalling cascade is, in different ways, related to H2O2-mediated signalling. In Arabidopsis, H2O2 has been shown to activate two MAPKs, i.e. AtMPK6 and AtMPK3 (Desikan et al., 1999, 2001; Grant et al., 2000; Kovtun et al., 2000), and H2O2 was also shown to induce expression of the Arabidopsis nucleotide diphosphate (NDP) kinase 2 (NDPK2), which again interact and activate AtMPK3 and AtMPK6 (Moon et al., 2003). In tobacco plants, H2O2 was shown to activate the orthologue of AtMPK6 and SIPK (Samuel et al., 2000) and, moreover, the activation of AtMPK3 and AtMPK6 by H2O2 was through a MAPKKK, ANP1 (Kovtun et al., 2000). In maize, H2O2 was recently reported to activate a 46 kDa MAPK (Zhang et al., 2006). Overall, it appears that MAPK cascades operate downstream of H2O2 action. Less information is available about the particular mechanisms for upstream H2O2 signal production, especially in the case of abiotic stress. Nevertheless, a few very recent investigations have provided some clues that MAPK cascades might also be involved in ROS generation. It has been shown that over-expression of a MAPKK could induce the expression of NbrbohB encoding the enzyme necessary for ROS production in Nicotianabenthamiana plants (Yoshioka et al., 2003). In Arabidopsis, it has been shown that expression of active mutants of AtMEK4 and AtMEK5 led to the generation of hydrogen peroxide in the cell (Ren et al., 2002). Thus, the relationship between MAPK and H2O2 signalling seems to be very complicated and many aspects of the link between MAPK and ROS signalling remain elusive (Pitzschke and Hirt, 2006).
AtMEK1 was first identified nearly 10 years ago (Morris et al., 1997). Based on yeast two-hybrid analysis, it was shown that a protein–protein interaction occurred between AtMEK1 and AtMPK4, and between AtMEKK1 (a MAPKKK) and MEK1, and co-expression of AtMPK4 and MEK1 complemented a growth defect of the yeast mpk1 and bck1 mutants (Ichimura et al., 1998). Also, an in vitro assay showed that AtMPK4 could be activated by AtMEK1 through threonine phosphorylation (Huang et al., 2000). AtMPK4 was identified as being activated by the bacterial elicitor, harpin (Desikan et al., 2001). More recently, it was shown that wounding, cold, drought, and high salt stresses could elevate AtMEK1 phosphorylation activity toward that of AtMPK4, at least in vitro, while AtMEK1 protein did not change significantly (Matsuoka et al., 2002). These findings apparently suggest that AtMEK1 might be involved in abiotic and biotic stress signalling. Nevertheless, the exact roles and detailed mechanisms for AtMEK1-mediated signalling remain unknown. In this study, it was found that ABA-, drought-, and high salt-induced CAT1 expression was mediated by AtMEK1. Furthermore, it has been demonstrated that AtMEK1 can mediate the ABA-triggered generation of H2O2, which again mediates CAT1 expression. With this, a major signalling cascade of the AtMEK1-mediated stress response has been identified which was proved to play crucial roles in the drought and salt responses in Arabidopsis.
Materials and methods
Plant materials and stress treatments
Arabidopsis thaliana (L.) plants were kept in a growth chamber at 22±2 °C with 16/8 h of light/dark and a relative humidity of 90%. For NaCl treatment, 2-week-old seedlings grown on MS agar plates were transferred onto filter paper saturated with 300 mM NaCl and the plants were incubated under light for different time-courses. For ABA treatment, 0.1 mM ABA was sprayed directly onto the seedlings growing on the MS agar plates and they were incubated in the light until sample collection. For the drought-stress treatment, 2-week-old seedlings growing in soil were harvested and dehydrated to 80% of their original fresh weight before being incubated in sealed containers at 100% relative humidity at 25 °C for different time-courses.
Identification and isolation of mek1 mutants
The T-DNA insertion mutant (SALK_015914) of the AtMEK1 gene, generated by the Salk Institute Genomic Analysis Laboratory (http://signal.salk.edu/), was obtained from the ABRC. The seeds were planted on agar plates containing kanamycin and the kanamycin-resistant plants were transferred to soil. Seeds were harvested separately from individual plants. Subsequently, to confirm the mutant line as homozygous, PCR was performed with the genomic DNA of mek1 mutants using gene-specific oligonucleotides (LP, 5′-AGATGGAATCCAGACCGTGTCTC-3′; RP, 5′-CGAGCAAAACCAGTCCCA A-3′) and left-border-specific primers (LBb1, 5′-GCGTGGACCGCTTGCTGCAACT- 3′).
Plasmid construction
The pCAT1-GFP, pCAT2-GFP, and pCAT3-GFP were prepared using pHBT95-GFP, which has a CaMV 35S promoter. The promoter of CAT1, CAT2, or CAT3 was substituted for the CaMV 35S promoter by XhoI and ApaI sites. XhoI and ApaI sites were introduced into the promoters of CAT1, CAT2, and CAT3 by PCR using the following primers: CAT1 promoter forward primer (5′-ACGCTCGAGAAACCATGAGGGATATTCGTG-3′) and reverse primer (5′-ATTCCCGGGTGCATCATTTTAACCGAGAG-3′); CAT2 promoter forward primer (5′-ACGCTCGAGAACCCGCGGTTTACCGCAG-3′) and reverse primer (5′-ATTCCCGGGAAATTAACATCAGAGTAATTTC-3′); CAT3 promoter forward primer (5′-ACGCTCGAGTATGTATATATGTATGATAT-3′) and reverse primer (5′-ATTCCCGGGGAAATCAACATCATCAACTTAG-3′).
For construction of the vector containing β-glucuronidase (GUS), the vector pHBT95-GFP was reconstructed and GUS substituted for GFP by using the sites NcoI and NotI on either side of the initiation codon and the stop codon of sGFP. The vector was named pHBT95-GUS. Then the promoter of CAT1, CAT2, or CAT3 was substituted for the CaMV 35S promoter by XhoI and ApaI sites as described above to gain pCAT1-GUS, pCAT2-GUS, and pCAT3-GUS.
Total RNA extraction, semi-quantitative RT-PCR and northern blot
Seedlings grown for 2 weeks on MS agar plates under continuous light were treated with NaCl, ABA, or drought stress as described above. Total RNA was extracted using the RNeasy Plant Mini Kit (Qiagen Inc.) according to the manufacturer's instructions and stored at –80 °C before being used for RT-PCR. Total RNA was used as a template for first-strand cDNA synthesis using the SuperScript II First-Strand Synthesis System for RT-PCR (Invitrogen, Carlsbad, CA). A PTC-100 Programmable Thermal Controller (MJ Research, Watertown, MA) was used. PCR products were separated on 1.5% agarose gels.
Fifteen micrograms of total RNA was separated on a 1% formaldehyde-containing agarose gel with an RNA molecular mass marker (Promega Corp., Madison, WI, USA). (equal loading of RNA was observed using ethidium bromide staining of the gel prior to transfer), and then blotted a nylon membrane (Hybond-N, Amersham Pharmacia Biotech, Aylesbury, UK) overnight at room temperature. The probes were labelled with [α-32P]dCTP using a random-primed DNA labelling kit (Megaprime, Amersham). Hybridization was performed according to the manufacturer's recommendations.
Protein extraction, immunoprecipitation, and kinase activity assay
The following steps were carried out at 4 °C unless stated otherwise. The plant tissues (3:1, buffer volume:fresh weight) were homogenized in a pestle and mortar with 100 mM TRIS-HCl buffer (pH 8.0) that contained 2 mM EDTA, 5 mM DL-dithiothreitol, 10% glycerol, 1 mM phenylmethylsulphonyl fluoride (PMSF), and 0.3 μM aprotinin. The homogenate was filtered through four layers of muslin cloth and centrifuged at 12 000 g for 40 min. The supernatant was desalted with a Sephadex G-25 column equilibrated with buffer suitable for individual enzymes. The desalted supernatant was stored in separate aliquots at –80 °C. The protein concentration was determined using the protein assay kit (Bio-Rad) with BSA as a standard.
Protein crude extracts (0.5 mg) were incubated with 50 μl of antibody at 4 °C overnight. Fifty microlitres of protein G-agarose beads was added and incubated for 2 h at 4 °C. The protein–antibody complex on the beads was collected and washed three times in ice-cold PBS and finally resuspended in protein sample buffer.
Kinase inactive GST–MPK4 fusion proteins were generated by exchanging a conserved lysine residue in the ATP binding domains to methionine and arginine using the Quick Change kit from Stratagene. The point mutations were performed as described by Markus et al. (2004). Two micrograms of inactive GST–MPK was incubated in 20 μl of kinase reaction buffer (50 mM TRIS, pH 7.5, 10 mM MgCl2, 1 mM DTT, 0.1 mM ATP, and 8 μCi of [32P] ATP) with immunoprecipitated GST fusion MEK1 from protoplasts. Kinase reactions were stopped after 30 min by adding 4 μl of SDS loading buffer and heating for 5 min at 95 °C Reaction products were analysed by SDS-PAGE, autoradiography and Coomassie Brilliant Blue R-250 staining.
Introduction of fusion genes into Arabidopsis protoplasts
The isolation of Arabidopsis protoplasts was according to the modified protocol from Abel and Theologis (1994). Leaves from 2-week-old Arabidopsis plants were washed in distilled water and incubated with enzyme solution containing 1% Cellulase R10, 0.25% Macerozyme R10 (both from Yakult, Tokyo, Japan), and 0.4 M mannitol for about 3–4 h at 23 °C with shaking (50 rev min−1). The protoplast suspension was filtered through a net with 150 μm pore diameter. After centrifugation (60 g, 2 min), the supernatant was discarded and the cells were resuspended in washing solution. The protoplasts were subsequently washed in washing buffer twice with Magma solution, respectively. The concentration of protoplasts was determined. The viability of the cells was verified by staining with FDA (fluorescein diacetate) and subsequent analysis with fluorescence microscopy (Zeiss Axioskop) (Nunberg and Thomas, 1993). Plasmid DNA solutions were adjusted to 0.5 M mannitol and mixed with 5×105 protoplasts. After the addition of an equal volume of PEG 6000 solution, the suspension was incubated at room temperature for 10 min and washed with washing and incubation (WI) buffer (0.5 M mannitol, 4 mM MES–KOH, pH 5.7, and 20 mM KCl). The pellets were resuspended in WI after centrifugation. The transfections were incubated in the dark (23 °C, 30 rev min−1, overnight). The suspensions was divided into different tubes, treated with ABA, NaCl solution, or PEG 6000 and incubated for different time-courses.
Assay for transient GUS activity
The suspension of protoplast was centrifuged (60 g, 2 min) and washed with 0.5 M mannitol. Pellets were dissolved in 105 μl of extraction buffer which is different according to the reporter gene. Extracts were vortexed and kept in –80 °C for more than 1 h or in liquid nitrogen for several minutes. Quantification of GUS activity was according to Fütterer et al. (1990) and a modified protocol for suppressing endogenous GUS activity (Kosugi et al., 1990). The protein concentration was measured using the protein assay kit (Bio-Rad) with BSA as a standard. GUS activity was measured in the supernatant after adding 4-methyl-umbelliferyl-β-D-glucuronide (MUG). The substrate MUG was hydrolysed by GUS-protein into 4-methyl-umbelliferone (4-MU) or 7-hydroxy-4-methyl-coumarin and glucuronide. GUS activity was determined on a dark micro-plate (Nunc GmbH & Co. KG) using the HTS 7000 plus Bioassay Reader (emission, 460 nm; excitation, 365 nm). GUS activity was normalized according to the expression derived from pHBT95-GUS in control experiments (average 204 pmol 4-MU produced min−1 μg−1 protein obtained from six independent experiments =1).
H2O2 measurement
An Amplex Red Hydrogen Peroxide/Peroxidase Assay Kit (Invitrogen, Carlsbad, CA) was used to measure H2O2 production in 2-week-old plants. Leaves were frozen in N2 and then ground. Then 500 μl of phosphate buffer (20 mM K2HPO4, pH 6.5) was added to 50 mg of ground frozen tissue. After centrifugation, 50 μl of the supernatant was incubated with 0.2 U ml−1 horseradish peroxidase and 100 μM Amplex Red reagent (10-acetyl-3,7-dihydrophenoxazine) at room temperature for 30 min under dark conditions. The fluorescence was quantified using FLUOStar Optima (excitation at 560 nm and emission at 590 nm).
Determination of O2·− production in Arabidopsis leaves
O2·− production in Arabidopsis leaves of treated and control plants was determined according to the method of Able et al. (1998) by monitoring the reduction of 3′-[1-(phenylamino-carbonyl)-3,4-tetrazolium]-bis(4-methoxy-6-nitro) benzenesulphonic acid hydrate (XTT) in the presence of O2·−. Leaves (1 g) were frozen in N2, ground, and then homogenized with 5 ml of 50 mM TRIS-HCl buffer (pH 7.5) and centrifuged at 5000 g for 10 min. The reaction mixture of 1 ml contained 50 μg of supernatant proteins, 50 mM TRIS-HCl buffer (pH 7.5), and 0.5 mM XTT. The reduction of XTT was determined at 470 nm for 5 min. Corrections were made for the background absorbance in the presence of 50 units SOD. O2·− production rate was calculated using an extinction coefficient of 2.16×104 M−1 cm−1.
Epidermal strip bioassay
Stomatal bioassay experiments were performed as described by Zhang et al. (2001) with slight modifications. To study the promotion of stomatal closure by ABA, freshly prepared abaxial epidermis was first incubated in 10 mM MES, pH 6.15, containing 50 mM KCl (MES–KCl) for 2 h under light to open the stomata (at 22–25 °C, under a photon flux density of 0.2–0.3 mmol m−2 s−1). The epidermal strips with open stomata were transferred to the above buffer MES-KCl in the presence of ABA (1 μM) for 4 h. Stomatal apertures were measured under the microscope at 1 h intervals for 50 randomly selected stomata. Each assay was repeated three times. The data were presented as mean ±SE (n=150).
Generation of AtMEK1 over-expressing plants
Full-length Arabidopsis AtMEK1 cDNA was obtained by using reverse transcription-PCR and cloned into pENTR-TOPO cloning vector (Invitrogen, Carlsbad, CA) and sequenced. After an LR reaction, AtMEK1 cDNA was inserted into pGWB5 vector (a gift from Professor Jiansheng Liang, Yangzhou University), named pGWB5-AtMEK1. Transgenic Arabidopsis expressing the GFP-tagged AtMEK1 from the cauliflower mosaic virus (CaMV) 35S promoter was generated using the floral dipping method (Clough and Bent, 1998) into Col-0 wild-type plants or mek1 null plants. Transformed plants were selected by growth on kanamycin-containing media. Plants of the second generation after transformation were used for the experiments. The same method was used to gain transgenic plants expressing the GFP-tagged CAT1, CAT2, and CAT3 promoters.
Drought and salt-tolerance analyses
For the drought assays, watering was withheld from 3-week-old soil-grown plants for specified times. To minimize experimental variations, the same number of plants in each comparison group was grown on the same tray. Watering was withheld from the plants for 20 d before photographs were taken and survival rates were counted. All experiments were repeated at least three times and results from one representative experiment are illustrated.
The effects of salt on seed germination were studied by determining the germination rates on half-strength Murashige and Skoog (MS) medium containing 150 mM NaCl. Germination rates were compared between seed lots that were produced, harvested, and stored under identical conditions. Before planting, seeds were surface-sterilized with 70% ethanol for 1 min, with 2% hypochlorite for 5 min, and rinsed five times with sterile deionized water. Fifty to 100 seeds from wild-type (Col-0), mek1, and MEK1-OE seeds were stratified at 4 °C for 48 h and planted in Petri dishes on half-strength MS 0.8% phytoagar medium at 22 °C under continuous white light.
Accession numbers
Sequence data from the article can be found in the GenBank data libraries or TIGR database (Arabidopsis thaliana Genome Project) under the following accession numbers: AtMEK1, At4g26070; AtMKK2, At4g29810; AtMKK3, At5g40440; AtMKK4, At1g51660; AtMKK5, At3g21220; AtMKK6, At5g56580; AtMKK7, At1g18350; AtMKK8, At3g06230; AtMKK9, At1g73500; AtMKK10, At1g32320; CAT1, At1g20630; CAT2, At4g35090; and CAT3, At1g20620.
Results
Gene expressions of the catalase multigene family in response to ABA, drought, and salt stresses
In Arabidopsis, catalases are encoded by a multi-gene family consisting of CAT1, CAT2, and CAT3. Although the amino acid sequences of the three genes are highly homologous with identities more than 70%, their expressions in responses to different stresses are rather different. CAT1 expression was dramatically increased in response to ABA, drought, and salt stress (Fig. 1A). It seems that the baseline transcription levels of CAT2 and CAT3 are higher than that of CAT1 in the non-stressed condition. As shown in Fig. 1A and C, the transcript level of CAT3 apparently increased in response to ABA treatment, whereas the salt-responding capacity of the CAT3 transcript level did not significantly increase compared with the control. CAT2 responded strongly to drought and salt stresses (Fig. 1B, C). As both CAT2 and CAT3 are known to be regulated by circadian rhythm and their maximal mRNA abundances occur in a morning-specific phase for CAT2 and an evening-specific phase for CAT3 (Zhong et al., 1994; Zhong and McClung, 1996), a diurnal course was used to collect samples in each case in order to detect any possible interference with the natural rhythm. Figure 1D shows the circadian-regulated CAT1, CAT2, and CAT3 mRNA abundances. The experimental plants were grown in a 16/8 h of light/dark. Tissue was harvested at timed intervals. Stress treatment started from 12 h in the light period to 8 h after dark because the mRNA abundances of CAT2 and CAT3 were relatively low and stable. The result showed that no significant changes in the level of CAT1 expression were found during the light or the dark period, suggesting that the enhanced expression of CAT1 totally resulted from stress treatments (Fig. 1D). Although both CAT2 and CAT3 were regulated by the circadian clock, the selected time-course to start stress treatments helped to avoid error. The enhanced expression of transcript levels of CAT2 or CAT3 resulted from the stress treatment rather than from circadian regulation.

Accumulation of the CAT1, CAT2, and CAT3 transcripts in response to ABA, drought and salt. (A) Changes in CAT1, CAT2, and CAT3 transcript accumulation in the presence of ABA. (B, C) Expression of CAT1, CAT2, and CAT3 genes induced by drought and salt. (D) Circadian regulation of CAT1, CAT2, and CAT3 transcript accumulation. Arabidopsis tissues from the same time-course were used for RNA gel blot analysis. Total RNA was extracted from wild-type plants without stress treatment (control) or subjected to ABA (100 μM ABA), NaCl (300 mM), and drought (loss of 20% fresh weight and incubated for different time-courses) treatments. The Actin2 gene was used as a loading control. All experiments were repeated at least three times with similar results.
ATMEK1 mediates CAT1expression in response to ABA, drought, and salt stress
In the search for the MAPKK signal family, all ten T-DNA insert mutants of MAPKKs were collected and downstream signalling was screened. MAPK cascades minimally consist of a MAPKKK-MAPKK-MAPK module. There are at least 20 MAPKs, 10 MAPKKs, and 60 MAPKKKs in the Arabidopsis genome (www.arabidopsis.org; Nakagami et al., 2005). In the subfamily of MAPKKs, insertion mutation within each member is available from the Arabidopsis Biological Resource Center (ABRC)'s collection of T-DNA transformed Arabidopsis lines (Alonso et al., 2003). Because MAPKKs constitute the smallest subfamily with mutations available for each member and, more importantly, because increasing evidence suggests that many members of this subfamily, such as MEK1, MEK2, MEK4, and MEK5, are involved in various upstream stress signalling (Asai et al., 2002; Matsuoka et al., 2002; Ren et al., 2002; Teige et al., 2004), the T-DNA insertion mutants of MAPKKs were screened first in order to identify the signal component mediating CAT gene family expression. Interestingly, ABA-, drought-, and salt-activated CAT1 expression in wild-type plants was blocked in mek1 mutant plants. ABA-induced CAT3 expression was also arrested in mek1 mutant plants, while drought-induced CAT3 expression showed no significant changes between wild-type and mek1 plants. By contrast with drought- or salt-induced CAT1, the expression of CAT2 activated by drought or salt stress could still be detected in the mek1 null plants. This suggests that there are different signalling pathways in the CAT gene family: AtMEK1 was involved in the ABA-, drought-, and salt-induced CAT1 signalling pathway and was also involved in the regulation of ABA-induced CAT3 expression; drought- or salt-induced CAT2 or CAT3 expression was not under the signal pathway of AtMEK1 (Fig. 2A). Congruent with northern-blot assays (Fig. 1), RT-PCR results showed that the specific inhibitor of MAPK kinase, PD98059, markedly reduced stress-induced CAT1 expression (Fig. 2B). Moreover, ABA-induced CAT3 expression appeared to be reduced by PD98059. Surprisingly, the MAPK kinase inhibitor had no effect on the drought- and salt-induced gene expression of CAT2 and CAT3. All these results indicate that MAPK was involved in CAT1 expression in response to different stresses.
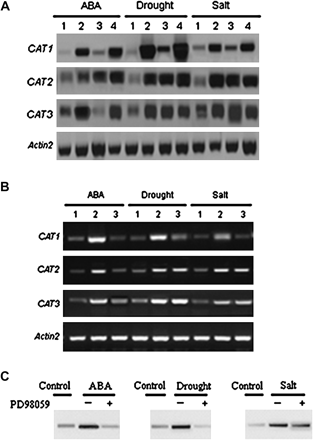
CAT1 expression in AtMEK1 mutant plants mek1 and the over-expressing lines and stress-induced AtMEK1 and CAT gene family activation and inhibition by PD98059. (A) Changes in the transcript levels of CAT, CAT2, and CAT3 induced by ABA, drought, or salt in wild-type, mutant, and over-expressing plants, mek1 and MEK1-OE by northern blot. 1, Control; 2, Col-0 lines; 3, AtMEK1 null mutant lines; 4, AtMEK1 over-expressing plants. The Actin2 gene was used as a loading control. (B) The transcript levels of ABA-, water deficit-, and salt-induced CAT1, CAT2, and CAT3 analysed by RT-PCR. Treatments were as follows: 1, control; 2, different treatments (0.1 mM ABA, drought stress for 4 h or 300 mM NaCl for 4 h); 3, 50 μM PD98059 (pretreated for 12 h) plus different treatments (0.1 mM ABA, drought stress for 4 h or 300 mM NaCl for 4 h). The Actin2 gene was used as a loading control. Experiments were repeated three times using samples from independent treatments. (C) Activation of AtMEK1 by ABA, drought, and salt stress and the effects of the MAPKK inhibitor PD98059 on different stresses. AtMEK1 activity was determined after transient expression in plant cells upon different stress treatments. GFP epitope-tagged AtMEK1 was immunoprecipitated from Arabidopsis protoplasts following stress treatments for 10 min: ABA (10 μM ABA), drought (30% PEG6000), salt (300 mM NaCl). AtMEK1 kinase activity was determined by in vitro kinase assays using kinase-inactive GST-MPK4 as a substrate. For the inhibitor experiment, protoplasts were pretreated with 0 μM (–) and 50 μM PD98059 (+) for 4 h and followed with the stress treatments. All experiments were repeated three times using samples from independent treatments.
In mek1 (SALK_015914), the T-DNA was located in the 5′ UTR near the start codon (Fig. 3A). Reverse-transcription PCR (RT-PCR) was used to determine the effect of T-DNA insertion on the messenger RNA levels of AtMEK1. While messenger RNA was detected in wild-type Columbia plants, no AtMEK1 message was detected in mek1 homozygous plants (Fig. 3B). To confirm further that stress-induced CAT gene family expression was mediated by AtMEK1, the full-length AtMEK1 sequence was placed under the control of CaMV-35S promoters and wild-type plants of Arabidopsis transformed. A total of 22 independent transgenic lines (T2) were generated and screened respectively for AtMEK1. Two or three lines for AtMEK1 transgenic plants were chosen for further analysis (Fig. 3C). ABA-, drought-, and salt-activated expression of CAT1 was increased in AtMEK1 over-expressing plants. The expressions of CAT2 and CAT3 induced by drought and salt stress were not significantly increased in the over-expressing plants (Fig. 2A).
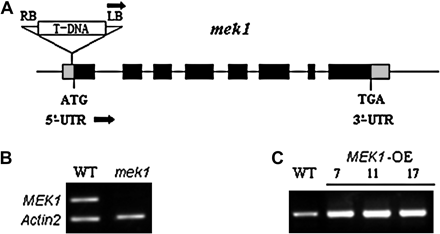
Verification of AtMEK1 mutants and RT-PCR analyses for AtMEK1 over-expressing plants. (A) LB, T-DNA left border; RB, T-DNA right border. Black boxes represent exons. The T-DNA insert is not drawn to scale. The black arrows at LB indicate the T-DNA left border primer, and the black arrows indicate the AtMEK1-specific primers used for mutant isolation. (B) Verification of AtMEK1 mutant, mek1. Reverse transcription-polymerase chain reaction (RT-PCR) analysis for AtMEK1 transcript. The AtMEK1 transcript was present in total RNA from wild-type Arabidopsis but absent in the AtMEK1 mutant. As a control, Actin2 primers that amplify a 500 bp product were added together with AtMEK1 primers in each PCR reaction. (C) The transcript levels of AtMEK1 in different over-expression lines analysed by RT-PCR. Total RNA was extracted from wild-type plants or AtMEK1 over-expressing plants.
In order to prove that AtMEK1 was indeed involved in ABA-, drought-, and salt-triggered signalling, the AtMEK1 kinase activities induced by ABA, drought, and salt stresses were examined using inactive MPK4 as the substrate. AtMEK1 of Arabidopsis have been proved to phosphorylate MPK4 (Matsuoka et al., 2002). The kinase activities were found to be activated by ABA, drought, and salt stresses. As shown in Fig. 2B, ABA, drought, and salt stresses all led to a significant increase in kinase activity, whereas the time-course of the MAPK activation was different in response to the different stresses. As expected, the MAPK kinase inhibitor, PD98059, substantially reduced the activation of the kinase activity induced by ABA, drought, and salt (Fig. 2C), which further proved that the inhibition of the stress-induced CAT1 expression by PD98059 was due to blocking of the activation of MAPK activity in response to various stresses.
To gain a better understanding of the regulation of CAT gene family expression by AtMEK1 under different treatments, three vectors were constructed in which the β-glucuronidase (GUS) reporter gene was under the control of the CAT1, CAT2, or CAT3 promoter, respectively. The expression of the promoter of CAT1, CAT2, or CAT3 linked to the coding sequence of GUS was examined in protoplasts prepared from suspension-cultured cells after PEG-mediated DNA uptake. Figure 4 shows the results of transient GUS assays for Arabidopsis protoplasts transformed with HBT95–GUS and three pCAT–GUS fusions. Since the CaMV-35S promoter confers constitutive expression to a downstream reporter gene (Skriver et al., 1991), HBT95 was used as the positive control plasmid of PEG-mediated transformation and also for the negative standard of inducible or responsive gene expression. The four vectors were transformed into protoplasts of Arabidopsis wild-type, AtMEK1 mutant, and AtMEK1 over-expressing plants, respectively.
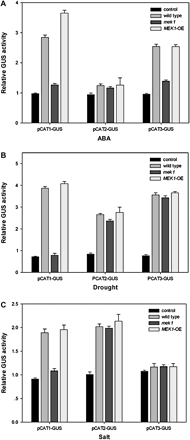
Transient GUS expression in Arabidopsis protoplasts transformed with three chimeric constructs. (A) The effects of ABA on transient GUS expression from pHBT95–GUS, pCAT1–GUS, pCAT2–GUS, and pCAT3–GUS in wild-type, mek1, and over-expressing plants. Three vectors containing the GUS reporter gene were constructed under the control of the promoter of CAT1, CAT2, and CAT3, respectively. The vectors were transformed into different Arabidopsis protoplasts (wild-type, mek1, and over-expressing) by incubating at 23 °C for 12 h, then treated with 100 μM ABA and incubated for another 16 h. GUS activities (pmol 4-MU produced min−1 μg−1 protein) were fluorimetrically determined and normalized by comparison with control experiments of pHBT95–GUS. Mean values from four independent experiments are shown. Vertical lines refer to standard errors. (B) The effects of drought on the transient GUS expression from pHBT95–GUS, pCAT1–GUS, pCAT2–GUS, and pCAT3–GUS in wild-type, mek1, and over-expressing plants. The experiments were repeated four times with similar results. (C) Changes in transient GUS expression from pHBT95–GUS, pCAT1–GUS, pCAT2–GUS, and pCAT3–GUS in wild-type, mek1, and over-expressing plants after treatment with 300 mM NaCl. Experiments were repeated three times using samples from independent treatments.
As shown in Fig. 4A, the application of 0.1 mM ABA to transformed protoplasts did not substantially change the pattern of GUS activities from the constitutive expression cassette. The reporter gene expression of pCAT1–GUS from wild-type plants was enhanced, with the average increase in enzyme activity being about 3.5-fold after treatment with ABA. It was shown that over-expression of AtMEK1 could promote stress-induced CAT1 expression when compared with the wild-type plants. Furthermore, in a transient expression system where the GUS gene was driven by the CAT1 promoter, it was shown that GUS activity could be activated in response to ABA, water deficit, and salt stress (Fig. 4) and again, such an activation of the GUS activity was either blocked or promoted, respectively, in mek1 or AtMEK1 over-expressing plants. All these results strongly demonstrate that AtMEK1 is involved in CAT1 expression in response to ABA, drought, and salt stress. Similar results were also observed in the ABA-induced CAT3 expression rather than the drought-activated expression of CAT3. For CAT2, there was a marked contrast according to the results from pCAT2–GUS, in which there was no apparent decrease in GUS activity in mek1 mutant plants, although both drought and salt activated the expression of CAT2 in wild-type Arabidopsis.
ATMEK1 mediates stress-induced H2O2 production
It is well known that the cellular ROS level is normally in homeostasis controlled by the antioxidative system. Thus, a change in activity of any antioxidative enzyme may cause a change in the cellular ROS level (Foyer and Noctor, 2005). AtMEK1, serving as a mediator in stress-induced CAT1 expression, should be expected to have some impact on H2O2 production. In order to reveal the particular roles of AtMEK1 in H2O2 production, the stress-induced changes in cellular H2O2 level were investigated further. As shown in Fig. 5, H2O2 production was very sensitive to ABA treatment, and ABA treatment led to an increase in H2O2 content by nearly 10-fold that in wild-type plants. Surprisingly, the AtMEK1 mutation did not promote ABA-induced H2O2 and, also, the over-expression of AtMEK1 did not inhibit H2O2 production as expected from the fact that AtMEK1 mediates ABA-induced gene expression of CAT1, whose activity is responsible for the release of H2O2. By contrast, while the ABA-induced increase in H2O2 production was almost completely blocked in mek1, it was significantly increased in plants with AtMEK1 over-expressed, indicating that AtMEK1 was involved in ABA-induced H2O2 accumulation. Drought and salt stress-induced H2O2 accumulation was also impaired to some extent in mek1, but, compared with the case of ABA treatment, such an impairment was much reduced (Fig. 5). Similar results were obtained with plants stained with 3, 3-diaminobenzidine (DAB), which also indicated that AtMEK1 was involved in ABA-induced H2O2 accumulation (unpublished results).
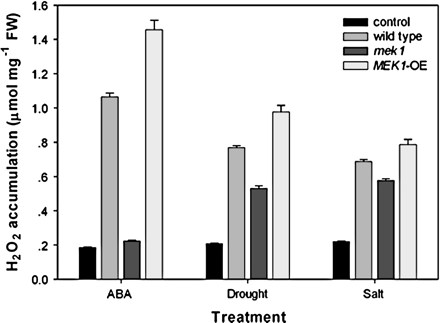
AtMEK1 mediates stress-induced H2O2 production and might be a mediator in ABA-triggered H2O2 production in guard cells. ABA-, water deficit-, and salt-induced H2O2 accumulation in wild-type and AtMEK1 mutant plants. Wild-type, AtMEK1 mutant, and AtMEK1 over-expressing seedlings were treated with 100 μM ABA, 300 mM NaCl, or drought and then H2O2 accumulation was assayed (detailed in the Materials and methods). All experiments were repeated three times using samples from independent treatments.
H2O2 is involved in AtMEK1-mediated CAT1 expression
As shown above (Fig. 5), stress-induced CAT1 expression was arrested in mek1 and stress-induced H2O2 was also arrested in mek1 and, equally, stress-induced CAT1 expression was promoted in AtMEK1 over-expressing plants and stress-induced H2O2 was also promoted in AtMEK1 over-expressing plants. This observation appeared to indicate that H2O2 was involved in AtMEK1-mediated CAT1 expression. To provide further evidence for this, the effect of H2O2 on the gene expression of the catalase family was investigated (Fig. 6). As speculated, CAT1 expression was dramatically induced in response to H2O2 treatment in a concentration-dependent manner. While CAT2 expression seemed to be slightly responsive to H2O2 at some concentrations, H2O2 seemed to have no effect on CAT3 expression. This result further indicates that H2O2 is involved in AtMEK1-mediated CAT1 expression.
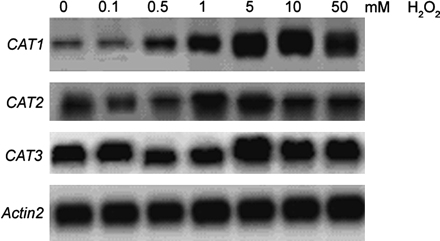
Response of the Arabidopsis CAT genes to 12 h treatment with H2O2. Seedlings were treated with increasing concentrations of H2O2 and CAT gene transcripts in leaves were detected after hybridization with 32P-labelled CAT1, 2, and 3 gene-specific probes, respectively. Equal loading of the gels was confirmed by Actin2.
AtMEK1 is involved in ABA-regulated stomatal movement
It has been established that H2O2 can act as a second messenger mediating ABA-induced stomatal closure (Pei et al., 2000; Schroeder et al., 2001). Hence, the present finding that AtMEK1 could mediate ABA-triggered H2O2 production in guard cells prompted an experiment to see whether AtMEK1 might possibly modulate ABA-regulated stomatal movement. In normal conditions, not much difference was found in the stomatal apertures among the wild-type, mek1, and AtMEK1 over-expressing plants (Fig. 7). ABA treatment significantly reduced stomatal aperture in both wild-type plants and plants over-expressing AtMEK1, but little difference in stomatal aperture was found between wild-type plants and the plants over-expressing AtMEK1, although it did seem that the decrease in stomatal aperture in AtMEK1 over-expressing plants was a little more sensitive than the wild-type plants during the initial phase (Fig. 7). However, while ABA treatment significantly reduced stomatal aperture in wild-type plants, the same treatment of ABA only led to a minor decrease in the stomatal aperture in mek1, which strongly indicates that AtMEK1 is involved in ABA-induced stomatal closing.
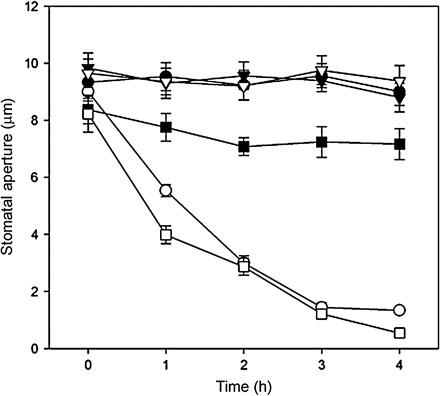
The effects of ABA on stomatal closure in guard cells of wild-type, mek1 null, and over-expressing Arabidopsis plants. Isolated epidermis of Arabidopsis was incubated in MES–KCl for 3 h under conditions promoting stomatal opening and were then transferred to fresh MES-KCl containing no ABA (open circles) and 1 μM ABA (filled circles) in the wild type; no ABA (open triangles) and 1 μM ABA (filled triangles) in mutants mek1; no ABA (open squares) and 1 μM ABA (filled squares) in over-expressing plants for only another 4 h. Stomatal apertures were determined with a project microscope at 1 h intervals during the 4 h incubation. Each assay was repeated three times. The data were presented as means of 150 measurements ±SE.
AtMEK1 over-expression gave Arabidopsis enhanced drought and salt tolerance
AtMEK1 over-expressing and mek1 null plants had no obvious phenotypic differences under normal conditions, but showed significant differences under drought stress. As shown in Fig. 8A, AtMEK1-overexpressing plants displayed increased drought tolerance compared with wild-type and mek1 null plants, whereas mek1 null plants exhibited a hypersensitivity to drought. While nearly 90% of the mek1 null plants died due to the drought treatment, more than 70% plants over-expressing AtMEK1 were still alive, showing that the AtMEK1 over-expressing lines had a strong tolerance to drought (Fig. 8A). Besides drought tolerance, AtMEK1 was also found to play a role in salt tolerance. Figure 8B shows the germination of seeds on salt-containing MS media. While little difference in germination rate was found between wild-type and AtMEK1 over-expressing plants, the germination of mek1 null plants was very sensitive to salt stress compared with wild-type or AtMEK1 over-expressing plants. As shown in Fig. 8C, while the germination rate of either wild-type or AtMEK1 over-expressing plants reached more than 90%, the germination rate of mek1 was only about 20%, suggesting a crucial role of AtMEK1 in the salt tolerance of Arabidopsis.
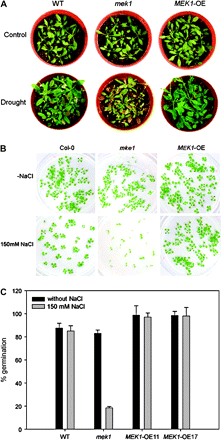
Phenotypic analysis of mek1 null and over-expressing lines. (A) Different drought tolerance. Watering was withheld from 3-week-old plants for 23 d before the photographs were taken. (B) Different salt tolerance. Germination of wild-type (Col-0), mek1 null, and AtMEK1 over-expressing plants was tested on agar plates without and with 300 mM NaCl. Germination was visually scored 10 d after putting plants into the light. (C) Statistical analysis of germination of wild-type (Col-0), mek1 null, and AtMEK1 over-expressing plants OE11 and OE17 on salt-containing media.
ABA, water deficit, salt-induced superoxide production in mek1, wild-type, and AtMEK1 over-expressing plants
To find out the possible reasons why AtMEK1 can render stress-tolerance to Arabidopsis, the effects of AtMEK1 on superoxide production were assessed. As shown in Fig. 9, and contrary to the change in H2O2 level, stress-induced superoxide production was significantly promoted in mek1, and, congruent with mek1, it was considerably reduced in AtMEK1 over-expressing plants. This result indicates that the stress-tolerance given by AtMEK1 results from the relief of oxidative damage and, therefore, AtMEK1 should hence play a crucial role in the regulation of the anti-oxidative system. It is not known how AtMEK1 can regulate superoxide production while it serves as a mediator of H2O2 production and CAT1 expression. Nevertheless, these findings indicate that AtMEK1 is involved in multiple signalling processes controlling the cellular ROS level.
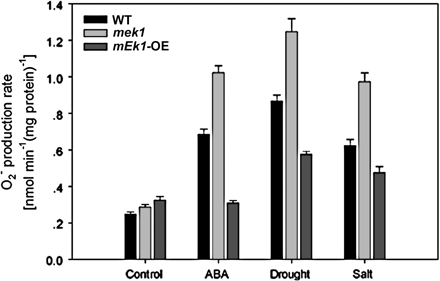
O2·− production rate after ABA, drought, and salt treatment in wild-type, mek1 null, and AtMEK1 over-expressing plants.
Discussion
Since H2O2 was identified as a key messenger more than 10 years ago (Levine et al., 1994), a large number of investigations have suggested that H2O2 can serve as a crucial signal in a wide range of cellular functions, such as stomatal movement, root hair development, programmed cell death, plant–pathogen interactions, and various responses to abiotic stresses (Pei et al., 2000; Bethke and Jones, 2001; Neill et al., 2002; Bais et al., 2003; Foreman et al., 2003; Apel and Hirt, 2004; Desikan et al., 2004; Foyer and Noctor, 2005; Vanderauwera et al., 2005; Gadjev et al., 2006; Pitzschke and Hirt, 2006). While the importance of H2O2 in cellular signalling has been established and roles of H2O2 in various aspects of cellular function have increasingly been identified, relatively little is known about the precise mechanism for the H2O2-mediated signalling. On the one hand, little is known about how H2O2 is perceived and finally transduced into specific cellular responses, and on the other hand, not much is known about how an initial stimulus is perceived by the cell and finally triggers H2O2 signal production (Pitzschke and Hirt, 2006).
Under normal condition, cellular ROS are constantly produced and maintained in homeostasis tightly governed by the antioxidant system (Gadjev et al., 2006; Pitzschke and Hirt, 2006,). Catalase is an iron porphyrin protein present in all aerobic organisms. As a releaser or door-keeper of H2O2, catalase plays crucial roles not only in protecting cells from oxidative damage but also in governing cellular redox status, thereby regulating cellular signalling (Baker and Orlandi, 1995; Neuenschwander et al., 1995; Low et al., 1996). Clearly, the mechanism for catalase gene expression in response to a stimulus is of particular importance that needs to be explored.
In Arabidopsis, catalase is encoded by a small multigene family consisting of three members, i.e. CAT1, CAT2, and CAT3 (Frugoli et al., 1996). It has been known that both CAT2 and CAT3 are regulated by the circadian clock (Zhong et al., 1994; Zhong and McClung, 1996). Unlike CAT2 and CAT3, CAT1 expression is not regulated by the circadian clock (Zhong et al., 1994; Zhong and McClung, 1996). In this study, a diurnal investigation was performed and it was demonstrated that CAT1 expression is strongly regulated by ABA, drought, and salt stress. By avoiding the effect of circadian regulation and taking into consideration the low and not very stable baseline mRNA abundances of CAT2 or CAT3, the trends of stress-induced expression of CAT2 or CAT3 mRNA abundance were significant and reliable (Fig. 1).
The members of the CAT gene family responded differently to different stresses. Thus, the present study has provided a more complex module for the expression of the catalase gene family, in which the signalling pathways mediating expression of a particular gene are intercrossed with each other, thus forming a complex network. In the signalling network, the pathway controlling CAT1 expression is a major cascade responsive to various abiotic stresses, which is different from the pathways controlling CAT2 or CAT3 expression although they are intercrossed at unknown steps. Interestingly, the difference in the signalling pathway between CAT1 and CAT2 or CAT3 is congruent with the difference in their spatial expression patterns (Frugoli et al., 1996). Hence, whether signalling leading to the gene expression of catalase is spatially modulated deserves to be investigated further.
Catalase serves as the releaser of H2O2. Hence, its gene expression should lead to a reduction in H2O2 level. However, while the gene expression of catalase was induced in response to stresses, especially to ABA treatment (Fig. 1), the H2O2 level was dramatically increased (Fig. 5). One possibility for this is that the cellular H2O2 level is actually controlled by many redox-controlling factors, and under ABA treatment the effect of the factor producing H2O2 is powerful enough to overwhelm the clearing of H2O2 by catalase. Another possibility is that the regulation sites for catalase gene expression and H2O2 production might be spatially or temporally separated, thus avoiding the rapid decomposing of H2O2 by catalase. In addition, the activation of catalase gene expression in response to stresses might actually be a feedback effect preventing a possible over-accumulation of H2O2, thus playing a crucial role in stress tolerance. Surprisingly, while H2O2 generation and accumulation have been demonstrated to be mediated by AtMEK1, mek1 was found to exhibit a hypersensitivity to drought and salt stresses, and correspondingly, AtMEK1 over-expressing plants were found to be more tolerant to the drought and salt stresses. From this, it is speculated that AtMEK1-mediated H2O2 generation might just be a messenger-triggering event, where H2O2 only serves as a messenger mediating the downstream stress-tolerant responses and actually has no obvious effect of damage to the cell function.
The roles of regulation of stomatal movement in stress tolerance have been well established (McAinsh et al., 1996; Torsethaugen et al., 1999; Burnett et al., 2000; Pei et al., 2000; Zhang et al., 2001; Gomi et al., 2005). Interestingly, in addition to the roles of AtMEK1 in H2O2 production and CAT1 expression, AtMEK1 was also found to be involved in ABA-induced stomatal closure in this study. A possible mechanism is that AtMEK1 is a mediator of H2O2 production, and H2O2 has been proved to be a second messenger in the signalling process of ABA-induced stomatal closure. Since H2O2 has been recognized as a second messenger for ABA-regulated stomatal movement, the signalling cascade upstream H2O2 production has been an attractive research topic. It is not known whether AtMEK1 is just the missing link between ABA perception and H2O2 production in guard cells. Hence, the precise mechanisms for AtMEK1-regulated stomatal movement deserve to be further investigated.
In summary, the data presented here demonstrate that stress-induced gene expression of the Arabidopsis catalase family consists of two major signalling pathways, i.e. the signalling pathway leading to CAT1 expression and the pathway leading to CAT2 or CAT3 expression (Fig. 10). AtMEK1 plays crucial roles in both H2O2 production and CAT1 expression, and H2O2 is involved in CAT1 expression. Thus, AtMEK1–H2O2 comprises a major signalling cascade mediating ABA-, drought-, and salt stress-induced CAT1 expression. Although drought can also induce CAT2 and CAT3 expression, the drought-induced CAT2 and CAT3 expression is mediated by an unknown signalling pathway in which AtMEK1 is not involved. There may be signalling crosstalk between the two pathways, because ABA was shown to induce CAT3, and, moreover, mek1 appeared to impair ABA-induced CAT3 expression. In conclusion, it has been demonstrated that AtMEK1 is a crucial signal mediating the regulation of the antioxidative system under stress conditions, and thereby plays important roles in both drought and salt-tolerance in Arabidopsis.

Proposed working model for the ABA-, drought-, and salt stress-triggered CAT pathway involving AtMEK1 as an upstream activator of the downstream CAT gene family.
This work was supported by the Hong Kong Research Grants Council (HKBU2465/05M), the University Grants Committee of Hong Kong (AoE/B-07/99), and a Hong Kong Baptist University Research Studentship (to Y Xing).
Comments