-
PDF
- Split View
-
Views
-
Cite
Cite
Steven Footitt, Johanna E. Cornah, Itsara Pracharoenwattana, James H. Bryce, Steven M. Smith, The Arabidopsis 3-ketoacyl-CoA thiolase-2 (kat2-1) mutant exhibits increased flowering but reduced reproductive success, Journal of Experimental Botany, Volume 58, Issue 11, August 2007, Pages 2959–2968, https://doi.org/10.1093/jxb/erm146
- Share Icon Share
Abstract
The enzyme 3-ketoacyl-CoA thiolase (KAT) (EC 2.3.1.16) catalyses a key step in fatty acid β-oxidation. In Arabidopsis thaliana, expression of the KAT2 gene is known to be required for the efficient mobilization of triacylglycerol during germination and seedling establishment. Here, data from the Arabidopsis kat2-1 mutant are presented, showing that perturbation of β-oxidation also affects vegetative growth and reproductive success. In the wild type, the KAT2 protein was detected in all organs tested. In the kat2-1 mutant, rosette leaf area and dry weight, but not leaf number, were greatly increased relative to wild type. Global proliferative arrest of flowering was delayed, resulting in increased silique production in kat2-1 plants. However, total silique dry weight was not increased. kat2-1 siliques were smaller and had a reduced seed number caused by increased ovule abortion. In kat2-1 ovules, carbon flow into sugars via gluconeogeneis and respiration were both reduced in comparison to the wild type. In conclusion, these data indicate that a functional β-oxidation pathway is required to maintain the balance between silique development and the continued initiation of floral meristems.
Introduction
In oil seeds, lipid stored in the form of triacylglycerol (TAG) is the main source of energy and carbon skeletons during germination and seedling growth prior to the attainment of photosynthetic competence. The mobilization of TAG requires the action of lipases that release fatty acids for oxidation by the peroxisomal β-oxidation pathway (Lin et al., 1986). The β-oxidation of fatty acids is catalysed by three proteins: (i) acyl-CoA oxidase of which there are several isozymes in Arabidopsis acting on fatty acids of different chain lengths; (ii) the multifunctional protein of which there are two isoforms, which exhibit hydratase, dehydrogenase, epimerase, and isomerase activities; and (iii) 3-ketoacylCoA thiolase which comprises a three-gene family. This pathway is the central component of the TAG mobilization system, being able to reduce fatty acid chain length by successively cleaving two carbons at each turn of the cycle to yield acetyl-CoA (Baker et al., 2006, and references therein). The resulting acetyl-CoA units are utilized by the glyoxylate cycle to produce succinate. This is converted to malate or oxaloacetate in the mitochondrion before undergoing gluconeogenesis (Eastmond and Graham, 2001). The β-oxidation pathway is also active throughout the life cycle of the plant. It appears to fulfil a general house-keeping role in the turnover of membrane lipids, and of galactolipids during leaf senescence (Graham and Eastmond, 2002).
Forward and reverse genetic screens have successfully identified many of the genes encoding proteins that provide substrate for, facilitate transport to, or directly participate in the peroxisomal β-oxidation pathway (Hayashi and Nishimura, 2003). The resulting mutants are revealing that apart from its established role in lipid catabolism, β-oxidation also influences developmental decision making via a role in intracellular signalling (Titorenko and Rachubinski, 2004; Baker et al., 2006). Examples of fatty acid-derived signal molecules generated by β-oxidation are indol 3-acetic acid (derived from indol 3-butyric acid) (Woodward and Bartel, 2005) and jasmonic acid (JA) (Rubio et al., 2006) derived from oxylipins (Theodoulou et al., 2005). This makes β-oxidation, as a source of JA, vital in the signalling response to wounding (Cruz Castillo et al., 2004).
Analysis of β-oxidation mutants has revealed a number of seed germination and seedling establishment phenotypes (Baker et al., 2006; Footitt et al., 2006; Rylott et al., 2006). However, relatively little attention has been paid to phenotypes of such mutants at later stages of plant growth and reproduction. One notable exception is aim1 (abnormal inflorescence meristem-1), a mutant of the multifunctional protein, MFP1, which displays proliferation of flowering, with abnormal flowers and reduced fertility (Richmond and Bleecker, 1999). A more subtle phenotype is the reduced basal level of JA and wound-induced JA seen in the 3-ketoacyl-CoA thiolase-2 (KAT2) mutant ped1 (Afitlhile et al., 2005), the COMATOSE mutants cts1 and cts2 (Theodoulou et al., 2005), and in antisense plants of acylCoA oxidase1 (ACX1) and KAT2 (Cruz Castillo et al., 2004).
Mutations in the Arabidopsis 3-ketoacyl-CoA thiolase gene, KAT2, were previously shown to result in the retention of oil bodies, peroxisome deformation, resistance to the pro-auxin herbicide 2,4-dichlorophenoxy butyric acid, and a requirement for exogenous sucrose for seedling establishment (Hayashi et al., 1998; Germain et al., 2001). A search of the Arabidopsis genome identified two other KAT genes, KAT1 and KAT5, on chromosomes 1 and 5, respectively (Germain et al., 2001). Inspection of publicly held Arabidopsis transcriptome data shows that KAT2 expression is several-fold higher than that of its two paralogues in most tissues and treatments (Zimmermann et al., 2004). KAT5 is only able to partially complement kat2-1 when driven by the 35S promoter (Germain et al., 2001). Furthermore co-expression analysis indicates that, unlike KAT2, KAT5 is not co-expressed with other β-oxidation genes but with those associated with flavonoid biosynthesis (Carrie et al., 2007). These data suggest that KAT2 is the primary thiolase of β-oxidation in Arabidopsis. To further our knowledge of the role of 3-ketoacyl-CoA thiolase-2 in plant development, vegetative and reproductive growth was investigated in the kat2-1 mutant. Data showing the role of KAT2 in vegetative growth and reproductive success are presented. The kat2-1 mutation is shown to alter both the vegetative and reproductive phase of the life cycle, indicating that β-oxidation has a potential role in resource allocation between (i) vegetative and reproductive organs and (ii) between developing embryos and floral meristems.
Materials and methods
Plant material and growth conditions
The kat2-1 mutant was isolated from an Arabidopsis thaliana T-DNA insertion population by Germain et al. (2001). Seeds of the kat2-1 mutant and the parent ecotype, Ws-4, were surface-sterilized in 10% (v/v) bleach for 10 min, then washed three times in sterile water. Seeds of kat2-1 were plated onto 0.8% (w/v) agar supplemented with half-strength MS salts (pH 5.7) and stratified in the dark at 4 °C for 3 d before being transferred to 24 h light (50 μmol m−2 s−1)/20 °C for 7 d. Seeds that had germinated but not established were then transferred to half-strength MS medium supplemented with 20 mM sucrose to enable establishment. At this point, wild-type seeds stratified as above were transferred to germination conditions. After a further 7 d, wild-type and mutant seedlings were transplanted to soil and plants grown to maturity in growth cabinets (16 h light/8 h dark at a constant temperature of 20 °C). During the light phase the incident photosynthetically active radiation was 50 μmol m−2 s−1 at soil level. The position of plant trays was systematically rotated to minimize light effects.
Western blot analysis of KAT2-1 expression
Total protein was extracted from the leaves, stems, buds, flowers from the primary inflorescence, and siliques of wild-type and kat2-1 plants. Total protein was also extracted from wild-type petals, sepals, stamens, carpels, and developing ovules and siliques. Western blot analysis used 10 μg protein/lane; equal loading was verified by pre-staining membranes with Ponceau S. The polyclonal KAT2 antibody used was raised against recombinant KAT2 protein as described previously (Germain et al., 2001).
Vegetative phenotype
Plants of kat2-1 and Ws-4 were harvested, commencing 10 d following transfer to soil and at 5 d intervals until siliques began to shatter. At each harvest 10 plants were taken and analysed individually for rosette and cauline leaf number, area, and dry weight; silique number and dry weight were also determined, as was the dry weight of the remainder of the aerial plant parts (inflorescences). Dry weights were determined after 24 h at 90 °C. Leaf area was determined from the weight of photocopied leaf images by linear regression to a weight/area calibration for paper. All data are presented as the mean ± standard error.
Reproductive phenotype
Flowering:
At the onset of flowering on the main raceme of the primary inflorescence, the total number of flowers opening was recorded daily from a population (n=14). Flowering buds were marked by applying acrylic paint to the pedicel. Flowering was denoted as first appearance of petals from within the enclosing sepals (stage 13) (Smyth et al., 1990). Data are presented as the cumulative population mean. At stage 13, flowers were dissected to expose stamens and pistils for determination of the long stamen:pistil ratio.
Silique and ovule development:
Following tagging of flowers, single samples of 10 siliques of known age were taken from 5 to 17 d after flowering (DAF) and their respiration rate, size, and dry weight determined. Respiration rate was measured as oxygen consumption using a Clark cell oxygen electrode at 20 °C. Total protein was extracted from developing ovules from 5 to 17 DAF for western analysis. The incidence of seed filling and abortion was determined at 15–17 DAF. The thousand-seed weight was determined for fully matured seeds stored at 20 °C for 5 d following harvest to allow maturation drying.
Developing ovules were collected from siliques from 5 to 17 DAF; those showing signs of abortion were excluded. Twenty ovules were transferred to 3.5 ml screw-top vials and labelled in triplicate with 0.2 μCi [14C]1-acetate in 1 mM sodium acetate buffered with 0.1 M HEPES (pH 7.4) (total volume 0.2 ml) for 18 h/20 °C in the dark on a shaker at 60 rpm. Labelled components were separated as follows. TAG was extracted in 200 μl heptane/isopropanol (3/2, v/v), then 66 μl of 0.1 M phosphate buffer (pH 2.5) and 26 μl 0.1 M phosphoric acid were added. The phases were then separated and the top heptane layer removed to a fresh tube containing 200 μl of 0.1 M phosphoric acid and mixed. The pellet and remaining aqueous phase were then re-extracted twice with 200 μl of heptane/isopropanol, then twice with heptane. The combined heptane phase was then mixed to partition the hydrophilic fraction back into the phosphate buffer. The combined heptane fraction was then separated by thin layer chromatography on LK6D silica gel 60A plates (Whatman) with hexane/diethyl ether/acetic acid (60/40/1 by vol.). Plates were stained with iodine vapour and 0.5% starch solution. The TAG fraction was removed to scintillation vials containing 10 ml Optiphase Hisafe scintillant (Perkin Elmer) and incorporation counted. The remaining two aqueous phases were combined, vacuum dried, resuspended in 50 mM acetic acid, and again vacuum dried to remove unincorporated acetate (Runquist and Kruger, 1999). The aqueous fraction was then resuspended in 500 μl H2O and separated into the neutral sugar, organic acid, and amino acid fractions after Harley and Beevers (1963) except the organic acid fraction was eluted with 2 N HCOOH and the amino acid fraction with 2 N NH4OH. Label incorporation was determined as above.
Results
Vegetative growth phenotype
In the parent ecotype, Ws-4, the KAT2 protein was detected in all vegetative and reproductive organs tested (Fig. 1) as well as in seedlings (Germain et al., 2001). The polyclonal antibody used here was previously shown to react strongly with recombinant KAT2 and KAT1, but weakly with KAT5 (Germain et al., 2001). This polyclonal antibody did not detect any of the KAT proteins in any of the kat2-1 organs tested (Fig. 1). This is consistent with a previous report that the antibody detected no protein in kat2-1 seedlings (Germain et al., 2001). Furthermore, KAT2 gene expression is several-fold higher than either KAT1 or KAT5 (Zimmermann et al., 2004). From the above expression data it is concluded that the protein detected here in flowering plants (Fig. 1) is predominantly KAT2. These observations imply that KAT2 and β-oxidation play a role throughout plant growth and development.
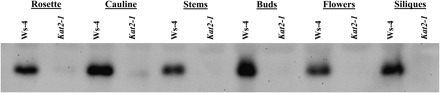
Western blot analysis for KAT2 protein expression in wild-type and kat2-1 organs. Protein extracts were prepared from rosette and cauline leaves, stems, buds, primary flowers, and young siliques. For each sample, 10 μg of total protein was used for SDS–PAGE and immunoblot analysis using antibodies raised against KAT2.
To determine whether the absence of a functional KAT2 had consequences for plant growth, plants of the kat2-1 knockout and Ws-4 were grown together under controlled environment conditions with a 16 h day to promote flowering. Total plant dry weight was similar in the wild type and mutant until 30 d after transfer to soil when it increased in kat2-1 above that of the wild type (Fig. 2). The experiment was stopped on day 40 to avoid seed loss through shattering of siliques. Analysis of leaf phenotypes showed that the number of rosette leaves arising during plant growth did not differ between the mutant and wild type (Fig. 3A). However, rosette leaf area and dry matter accumulation were greater in kat2-1 but declined to wild-type levels by day 40 (Fig. 3B, C). The fact that rosette leaf number does not differ between kat2-1 and wild-type plants, and their final leaf area and dry matter are the same, indicates that development is not delayed in the mutant. The kat2-1 mutant produced more cauline leaves, and continued producing them beyond the point when wild-type plants had stopped (Fig. 3D). Cauline leaf area and dry matter accumulation only differed after 30 d when it increased in the still-flowering mutant (Fig. 3E, F). In Arabidopsis, cauline leaves arise at sites of secondary branching. The increase observed in cauline leaf number was reflected by an increase in the formation of secondary racemes (number not determined) on each inflorescence which gave kat2-1 plants a more bushy appearance than the wild type. This increase in branching was reflected in an increase in dry weight of inflorescence tissue remaining after removal of leaves and siliques above that of the wild type. In the wild-type inflorescence, dry weight stabilized beyond day 26 but increased to double the wild-type value in kat2-1 (Fig. 4).
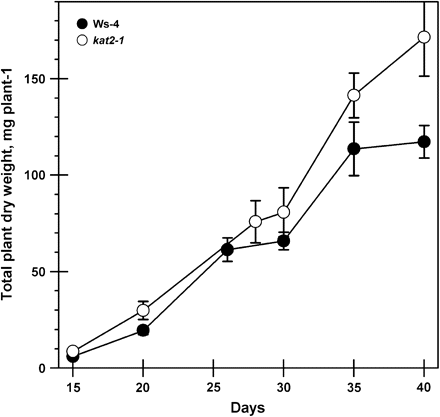
Total plant dry weight accumulation during plant development. The dry weights of all components from individual plants were combined at each harvest for both wild-type and kat2-1 plants. All data represent the mean ±SE (n=10).
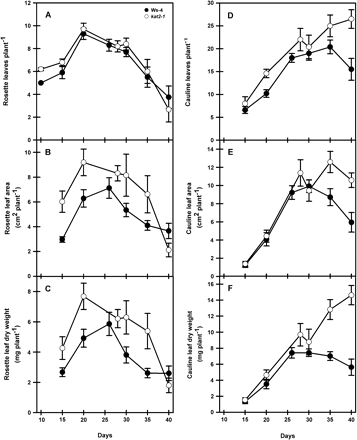
The development of rosette and cauline leaves during whole plant growth. At each harvest, rosette and cauline leaves from individual plants were collected, and leaf number, area, and dry weight were recorded. All data represent the mean ±SE (n=10).
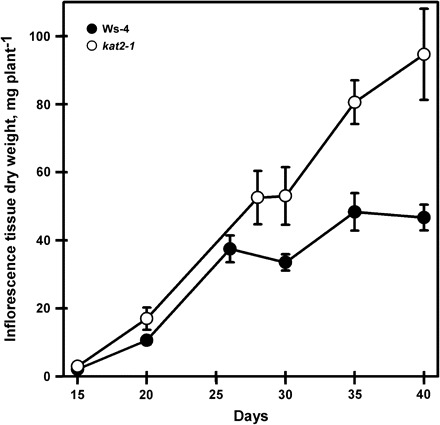
Dry weight of inflorescence tissue produced during plant development. The dry weight of inflorescence tissue from individual plants was determined after removal of leaves and siliques at each harvest for both wild-type and kat2-1 plants. All data represent the mean ±SE (n=10).
Reproductive growth phenotype
Floral phenotype:
The expression of KAT2 seen in wild-type flowers was found to occur in all floral organs (Fig. 5A). Overall flower morphology appeared normal except at flower stage 13 when the long stamen:pistil ratio was lower in kat2-1 (0.74±0.01, n=50) than in Ws-4 (0.82±0.01, n=58). Stamen length subsequently recovered to allow pollination. Further analysis of flowering on the primary raceme of the primary inflorescence showed that in kat2-1 the production of flowers commenced later than in the wild-type but continued for at least 6 d longer such that, when measurements ceased, kat2-1 had borne appreciably more flowers than the wild type. The time from the onset of flowering to its cessation was 15 d in the wild type and at least 18 d in kat2-1 (Fig. 5B).
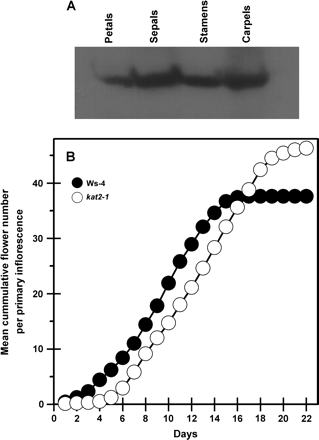
KAT2 protein expression in wild-type flowers and flower production on the primary inflorescence. (A) Expression of the KAT2 protein in wild-type floral organs. For each sample, 10 μg of total protein was used for SDS–PAGE and immunoblot analysis using antibodies raised against KAT2. (B) Cumulative flowering on the primary inflorescence. At the onset of flowering, the total number of flowers to open each day in a population of 14 plants was recorded for the wild type and kat2-1. All data represent the population mean (n=14).
Silique phenotype:
The greater number of cauline leaves in kat2-1 relative to Ws-4 (Fig. 3D) is a reflection of an increased number of secondary racemes arising from each inflorescence. The consequences of increased flowering in kat2-1 on subsequent silique development was analysed in both the whole plant and on siliques harvested on specific days after flowering. In whole plants, silique production had stopped in the wild type by day 35 but continued in kat2-1 plants, which by day 40 had produced 50% more siliques than the wild type (Fig. 6A). However, silique dry matter accumulation was the same in kat2-1 and the wild type at day 40 (Fig. 6B). Subsequent seed yield of whole plants was substantially reduced in kat2-1 (Table 1).
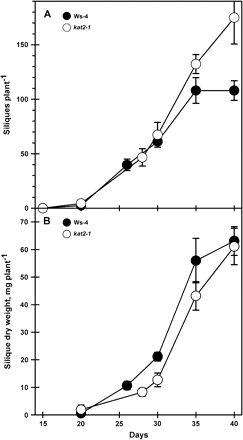
Total silique production during whole plant growth. (A) The total number of siliques on each plant was recorded at harvest. (B) The total dry weight of siliques on each plant was determined at harvest. All data represent the mean ±SE (n=10).
Full seeds (n=20 siliques) | Aborted seeds | Seed yield mg plant−1 (n=6) | 1000 seed wt (mg) (n=5) | |
Ws-4 | 42.3±2.1 | 2.5±1.7 | 41.4±2.0 | 14.3±0.2 |
kat2-1 | 16.9±1.7 | 19.3±1.5 | 25.5±1.5 | 13.7±0.1 |
Full seeds (n=20 siliques) | Aborted seeds | Seed yield mg plant−1 (n=6) | 1000 seed wt (mg) (n=5) | |
Ws-4 | 42.3±2.1 | 2.5±1.7 | 41.4±2.0 | 14.3±0.2 |
kat2-1 | 16.9±1.7 | 19.3±1.5 | 25.5±1.5 | 13.7±0.1 |
Mature siliques were dissected and the number of full and aborted seeds determined. The thousand-seed weight was determined using seeds from the same plants. The total seed yield was determined in six individual plants of the wild type and mutant. All data represent the mean ±SE.
Full seeds (n=20 siliques) | Aborted seeds | Seed yield mg plant−1 (n=6) | 1000 seed wt (mg) (n=5) | |
Ws-4 | 42.3±2.1 | 2.5±1.7 | 41.4±2.0 | 14.3±0.2 |
kat2-1 | 16.9±1.7 | 19.3±1.5 | 25.5±1.5 | 13.7±0.1 |
Full seeds (n=20 siliques) | Aborted seeds | Seed yield mg plant−1 (n=6) | 1000 seed wt (mg) (n=5) | |
Ws-4 | 42.3±2.1 | 2.5±1.7 | 41.4±2.0 | 14.3±0.2 |
kat2-1 | 16.9±1.7 | 19.3±1.5 | 25.5±1.5 | 13.7±0.1 |
Mature siliques were dissected and the number of full and aborted seeds determined. The thousand-seed weight was determined using seeds from the same plants. The total seed yield was determined in six individual plants of the wild type and mutant. All data represent the mean ±SE.
Wild-type siliques harvested on specific days after flowering from the primary inflorescence showed KAT2 expression throughout their development (Fig. 7A). Further analysis showed that kat2-1 siliques were smaller. This was reflected in the reduced length (Fig. 7B) and dry matter accumulation (data not shown) of kat2-1 siliques. Respiration rates of whole siliques in both kat2-1 and the wild type was highest at 9 DAF and, on a dry weight basis, respiration did not differ between the two (data not shown).
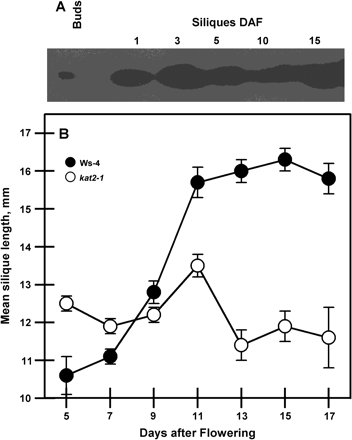
Silique development. (A) Expression of KAT2 protein in buds and developing siliques at increasing days after flowering (DAF). For each sample, 10 μg of total protein was used for SDS–PAGE and immunoblot analysis using antibodies raised against KAT2. (B) Changes in silique length during their development on the primary inflorescence. All data represent the mean ±SE (n=10).
Ovule phenotype:
Western analysis of developing ovules from the wild type showed KAT2 expression throughout development (Fig. 8). Feeding of labelled acetate to developing ovules from 5 to 17 DAF showed that incorporation of label into TAG was unaffected, indicating that uptake was not compromised. However, incorporation of label into neutral sugars and organic acids was reduced in kat2-1 in comparison to the wild type. Incorporation of label by kat2-1 into amino acids was barely detectable (Fig. 9). Developing kat2-1 ovules were smaller than the wild type and ovule abortion was seen by 9 DAF. At maturity a high incidence of abortion and a reduced seed weight were recorded (Table 1), which may be causally related to the compromised respiration rate (data not shown). The normal respiration rate of siliques may reflect the fact that most of the silique is photoautotrophic while the ovules exhibit heterotrophic metabolism.
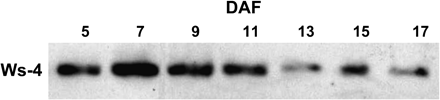
Expression of KAT2 during ovule development. Ovules were removed from siliques of known developmental age at increasing days after flowering (DAF). For each sample, 10 μg of total protein was used for SDS–PAGE and immunoblot analysis using antibodies raised against KAT2.
![The cumulative incorporation of [14C]1-acetate by developing seeds into TAG, neutral sugars, organic acids, and amino acids. Developing seeds were labelled for 18 h and then labelled metabolites were extracted and fractionated. All data represent the mean ±SE (n=3).](https://oup.silverchair-cdn.com/oup/backfile/Content_public/Journal/jxb/58/11/10.1093/jxb/erm146/2/m_jexboterm146f09_lw.gif?Expires=1748046992&Signature=3FnpC9-6KLVCJPbnv~ZLd6nyq5zlHy9wLDtb4PNdYnCHvutdztohEskDSEX3qqqgNFtXGx~cICdqJpjL90~EKH-yd8FYsKn550Cy4cCd8pif7CbKjYptwO80-ori0Wf9O5ZfbMYBzaEX10nfrZQqMnoyNxx0xN3g0SokZY95dqTwnoDS3DHUSD8s0H7jeFWIAhhnuVhC6c9BvLhg6qCegFixv~gKf9jMrwFEY4a2HDVFfXhBYbSFU6Yt2DSNBCvqKQYDga8ctmbf7fE66pg6xSFf3qaM7DNyq7Y3gy-bLadOLW6q-aYfz3RTT7nDjwLCCAhq5PHIF3QLHaTl0HGetw__&Key-Pair-Id=APKAIE5G5CRDK6RD3PGA)
The cumulative incorporation of [14C]1-acetate by developing seeds into TAG, neutral sugars, organic acids, and amino acids. Developing seeds were labelled for 18 h and then labelled metabolites were extracted and fractionated. All data represent the mean ±SE (n=3).
Discussion
This study was conducted to investigate the role of KAT2 and, by implication, β-oxidation in overall plant development using the kat2-1 knockout mutant. The KAT2 mutants, kat2-1 and ped1, have previously been reported to exhibit reduced germination potential, a requirement for exogenous sucrose for seedling establishment, retention of TAG, abnormal peroxisome morphology, and reduced jasmonate levels (Hayashi et al., 1998; Germain et al., 2001; Cruz Castillo et al., 2004; Pinfield-Wells et al., 2005; Footitt et al., 2006). Here widespread phenotypic changes during the reproductive growth phase as a consequence of the kat2-1 mutation are reported. These changes were not the result of delayed vegetative development as indicated by rosette leaf number or delayed transition to the reproductive growth phase as indicated by the first appearance of cauline leaves and increased production in inflorescence tissue in the mutant.
The KAT2 protein was expressed in all wild-type organs tested indicating a continuing requirement for β-oxidation during the plant's life cycle, consistent with previous observations (Charlton et al., 2005). Both vegetative and reproductive growth phases exhibited increased rosette (vegetative) and cauline (reproductive) leaf area and dry matter accumulation in the mutant. By contrast, the β-oxidation mutant aim1 showed a reduction in rosette leaf size (Richmond and Bleecker, 1999). In kat2-1, this increased leaf area would be expected to increase the growth potential of the mutant. This was reflected in increased dry matter accumulation resulting from an increase in inflorescence tissue. Interestingly, total silique dry matter in kat2-1 was consistent with its wild type despite the large increase in silique number. The increase in inflorescence tissue and overall reduction in silique size is consistent with that seen in aim1 and could imply that during the prolonged flowering period energetic constraints govern subsequent silique and ovule development.
Developing kat2-1 siliques exhibited an increased incidence of embryo abortion which reduced overall seed yield. Reduced seed yield is also seen in other β-oxidation mutants such as the acx3acx4 double mutant and aim1 (Richmond and Bleecker, 1999; Rylott et al., 2003). It is likely that these mutations result in substrate limitation, particularly in availability of fatty-acyl CoA units for β-oxidation, in the case of the acx3acx4 double mutant. β-Oxidation itself would be compromised in aim1, a mutant of the multifunctional protein of β-oxidation. In kat2-1, substrate limitation would be downstream of this β-oxidation mutant. However, further effects of the kat2-1 mutation have been observed in the reduced number of, but increased size of, peroxisomes in cotlyledons potentially caused by the accumulation of fatty acyl-CoAs within the peroxisome (Germain et al., 2001); a similar effect is seen in the citrate synthase double mutant csy2-1csy3-1 (Pracharoenwattana et al., 2005). Such perturbations may have significant consequences regarding resource allocation. This may be especially so in peroxisome-rich tissues such as flowers and particularly the pistil/young silique (Schilmiller et al., 2007). This reduction in the overall size of the silique metabolic sink results in an alteration in resource allocation which underlies the observed continuation in the initiation of floral meristems, prolongation of the flowering period, and increased secondary branching.
The delay in the cessation of flowering or global proliferative arrest (GPA) in kat2-1 suggests that β-oxidation plays a role not only in ovule development but in metabolic signalling between developing ovules and floral meristems. That GPA is rapid and global in the wild type suggests a threshold response to a putative signal and appears to be a function of ovule development rather than fertilization (Hensel et al., 1994). Whether GPA is brought about by an increase in the overall size of the ovule metabolic sink beyond a critical point where floral meristems cannot be maintained or by a developmental signal is unclear. However, energetic events occurring during ovule development potentially dominate any perturbation of signalling. The KAT2 protein was shown to be strongly expressed in developing wild-type ovules consistent with the observations of Charlton et al. (2005). The acyl-CoA oxidase genes ACOX3 and ACOX4 specific for short- and medium-chain acyl CoA esters are also strongly expressed during embryogenesis, and the double mutant is embryo lethal (Rylott et al., 2003). Overall, embryo development appears to be highly dependent on a functional β-oxidation pathway.
Energetic consequences of the kat2-1 mutation
In developing kat2-1 ovules the reduced incorporation of labelled acetate into neutral sugars indicated that gluconeogensis was compromised. This was also reflected in a reduction in label appearing in organic acids. This indicates that carbon flow into the glyoxylate cycle was reduced. That even less label was detected in the amino acid fraction suggests carbon was being diverted to energy provision. A further consequence of the kat2-1 lesion was a decrease in ovule respiration (data not shown). By contrast, ovule respiration in the glyoxylate cycle mutants, mls1 and icl2 did not differ from the wild type (S Footitt, SM Smith, unpublished results). This decrease in respiration in kat2-1 may be a consequence of two related events. (i) The accumulation of long-chain acyl CoA esters as reported previously for this and other β-oxidation mutants (aim1, mfp2, cts1, and cts2) (Richmond and Bleecker, 1999; Germain et al., 2001; Footitt et al., 2002; Rylott et al., 2006), which is observed to result in perturbation of peroxisome form in kat2-l and csy2-1csy3-1 (Germain et al., 2001; Pracharoenwattana et al., 2005). Such perturbations may result in altered transport of di- and tricarboxylic acids from the peroxisome as seen in rat mitochondria where long-chain acyl CoA esters inhibit respiration via inhibition of the dicarboxylate transporter and the ATP/ADP carrier when succinate or glutamate are the major respective respiratory substrates (Ventura et al., 2005). Although, no peroxisomal transporters for di- and tricarboxylic acids have been identified in Arabidopsis it is possible that the comparable mitochondrial transporters are dually localized (Kunze et al., 2006). (ii) The expected downstream effect of kat2-1 is a decreased flow of carbon skeletons into the glyoxylate cycle reducing the pool of citrate and succinate available for respiration. Citrate derived from TAG via β-oxidation has been demonstrated to be an important source of respiratory substrate (Pracharoenwattana et al., 2005). This may have added importance as there is some evidence that the glyoxylate cycle may be limiting in Arabidopsis reproductive organs. Inspection of publicly held Arabidopsis transcriptome data shows expression of the key enzymes of the glyoxylate cycle, isocitrate lyase and malate synthase, to be negligible in reproductive organs, while that of citrate synthase2 and 3 is many-fold higher (Table 2) (Zimmermann et al., 2004). Furthermore, malate synthase could not be detected by RT-PCR or by use of an MLS::LUC construct in Arabidopsis reproductive organs with expression only increasing during senescence or starvation of photosynthate (Charlton et al., 2005). This suggests citrate rather than succinate is the predominant lipid-derived respiratory substrate in reproductive organs. As developing embryos appear to be highly dependent on β-oxidation, a reduction in attendant respiration may be a contributing factor to embryo abortion if the size of metabolic sinks between competing sibling embryos within a silique becomes critical.
Gene expression levels of ISOCITRATE LYASE, MALATE SYNTHASE, and CITRATE SYNTHASE2 and 3 in Arabidopsis reproductive organs
Gene | Gene expression levels in specific organs | ||||
Flower | Carpel | Ovary | Stigma | Silique | |
ICL (At3g21720) | 33±6 | 11±2 | 9±1 | 7±1 | 335±144 |
MLS (At5g03860) | 53±5 | 54±15 | 14±5 | 47±10 | 174±37 |
CSY2 (At3g58750) | 2622±201 | 1199±87 | 826±83 | 954±16 | 2143±113 |
CSY3 (At2g42790) | 1881±157 | 929±89 | 491±33 | 779±86 | 2491±262 |
Gene | Gene expression levels in specific organs | ||||
Flower | Carpel | Ovary | Stigma | Silique | |
ICL (At3g21720) | 33±6 | 11±2 | 9±1 | 7±1 | 335±144 |
MLS (At5g03860) | 53±5 | 54±15 | 14±5 | 47±10 | 174±37 |
CSY2 (At3g58750) | 2622±201 | 1199±87 | 826±83 | 954±16 | 2143±113 |
CSY3 (At2g42790) | 1881±157 | 929±89 | 491±33 | 779±86 | 2491±262 |
Data represents mean ±SE. Data are reproduced from Geneinvestigator (Zimmermann et al., 2004).
Gene expression levels of ISOCITRATE LYASE, MALATE SYNTHASE, and CITRATE SYNTHASE2 and 3 in Arabidopsis reproductive organs
Gene | Gene expression levels in specific organs | ||||
Flower | Carpel | Ovary | Stigma | Silique | |
ICL (At3g21720) | 33±6 | 11±2 | 9±1 | 7±1 | 335±144 |
MLS (At5g03860) | 53±5 | 54±15 | 14±5 | 47±10 | 174±37 |
CSY2 (At3g58750) | 2622±201 | 1199±87 | 826±83 | 954±16 | 2143±113 |
CSY3 (At2g42790) | 1881±157 | 929±89 | 491±33 | 779±86 | 2491±262 |
Gene | Gene expression levels in specific organs | ||||
Flower | Carpel | Ovary | Stigma | Silique | |
ICL (At3g21720) | 33±6 | 11±2 | 9±1 | 7±1 | 335±144 |
MLS (At5g03860) | 53±5 | 54±15 | 14±5 | 47±10 | 174±37 |
CSY2 (At3g58750) | 2622±201 | 1199±87 | 826±83 | 954±16 | 2143±113 |
CSY3 (At2g42790) | 1881±157 | 929±89 | 491±33 | 779±86 | 2491±262 |
Data represents mean ±SE. Data are reproduced from Geneinvestigator (Zimmermann et al., 2004).
Signalling consequences of kat2-1 mutation
As our Knowledge of peroxisome function increases (Titorenko and Rachubinski, 2004; Baker et al., 2006) the role of β-oxidation as a focal point in signal processing becomes more apparent. Signal molecules derived via β-oxidation include JA (Creelman and Mulpuri, 2002; Rubio et al., 2006) and auxin via indole butyric acid (Bartel et al., 2001). Both JA and auxin are involved in filament elongation prior to pollination (Stintzi and Browse, 2000; Feng et al., 2006). This may explain the reduced long anther length seen in kat2-1. Although the kat2-1/ped1 mutants have reduced JA levels (Cruz Castillo et al., 2004; Afitlhile et al., 2005) it is not presently known what effect these lesions have on auxin levels.
The main conclusion of this study is that, in the developing ovule, KAT2 has an important role in the determination of reproductive success. These findings and those on studies of other β-oxidation mutants such as aim1 and the acx3acx4 double mutant (Richmond and Bleecker, 1999; Rylott et al., 2003) indicate that reproductive growth is dependent on peroxisomal β-oxidation. The disruption of carbon flow through β-oxidation results in reduced ovule sink size leading to alterations in resource allocation in favour of floral meristems.
The authors wish to thank Frederica Theodoulou for helpful comments on the manuscript. This research was funded by BBSRC grant RSP07677 to SMS and JHB. SMS acknowledges the award of an Australian Research Council Federation Fellowship. We also acknowledge financial support from the Thai Government Development and Promotion of Science and Technology Talents Project PhD studentship awarded to IP.
References
Author notes
Present address: Warwick HRI, University of Warwick, Wellesbourne, Warwick CV35 9EF, UK.
Comments