-
PDF
- Split View
-
Views
-
Cite
Cite
Leana Van der Vliet, Carol Peterson, Beverley Hale, Cd accumulation in roots and shoots of durum wheat: the roles of transpiration rate and apoplastic bypass, Journal of Experimental Botany, Volume 58, Issue 11, August 2007, Pages 2939–2947, https://doi.org/10.1093/jxb/erm119
- Share Icon Share
Abstract
Cadmium is readily taken up from soils by plants, depending on soil chemistry, and variably among species and cultivars; altered transpiration and xylem transport and/or translocation in the phloem could cause this variation in Cd accumulation, some degree of which is heritable. Using Triticum turgidum var. durum cvs Kyle and Arcola (high and low grain Cd accumulating, respectively), the objectives of this study were to determine if low-concentration Cd exposure alters transpiration, to relate transpiration to accumulation of Cd in roots and shoots at several life stages, and to evaluate the role of apoplastic bypass in the accumulation of Cd in shoots. The low abundance isotope 106Cd was used to probe Cd translocation in plants which had been exposed to elemental Cd or were Cd-naïve; apoplastic bypass was monitored using the fluorescent dye PTS (8-hydroxy-1,3,6-pyrenetrisulphonate). Differential accumulation of Cd by ‘Kyle’ and ‘Arcola’ could be partially attributed to the effect of Cd on transpiration, as exposure to low concentrations of Cd increased mass flow and concomitant Cd accumulation in ‘Kyle’. Distinct from this, exposure of ‘Arcola’ to low concentrations of Cd reduced translocation of Cd from roots to shoots relative to root accumulation of Cd. It is possible, but not tested here, that sequestration mechanisms (such as phytochelatin production, as demonstrated by others) are the genetically controlled difference between these two cultivars that results in differential Cd accumulation. These results also suggest that apoplastic bypass was not a major pathway of Cd transport from the root to the shoot in these plants, and that most of the shoot Cd resulted from uptake into the stele of the root via the symplastic pathway.
Introduction
Concentrations of Cd in durum wheat (Triticum turgidum L. var. durum) grown on the North American prairies may exceed the proposed draft maximum concentration for cadmium in wheat grain and rice (0.2 mg kg−1) set by the Codex Committee (Garrett and Gawalko, 2000; Joint FAO/WHO Food Standards Programme Codex Alimentarius Committee, 2002). Agricultural practices (Grant et al., 1998) and plant genetics (Archambault et al., 2001) have both been shown to contribute to the variation in Cd concentration in grain observed among cultivars and fields. The physiological bases of these two sources of variation could be uptake by the root, as well as translocation from the root to the shoot, transpiration being the driver for bulk water flow in both of these processes. Accumulation of Cd by roots is positively related to the number of root tips (Berkelaar and Hale, 2000), supporting the observation that they are much more active in Cd uptake than are the distal sections of the root (Piñeros et al., 1998). Root accumulation of Cd is both passive (covalent or ionic bonding to functional groups on cell walls) and transmembrane (using transporters for Zn or Ca: Herren and Feller, 1977). Its fate after accumulation is species-dependent: the 109Cd sequestered in white lupin roots (either in the vacuole or in the cell wall) was soluble, and a very small amount of the accumulated Cd was transported to shoots from the roots to be distributed throughout the leaf tissue (Page et al., 2006). Tobacco (Nicotiana tabacum L.) transformed with various Arabidopsis CAX genes for cation transporters that are typically tonoplast-localized accumulated more Cd in roots of plants with high- than low-expressing genes (Koren'kov et al., 2007), suggesting a molecular basis for root loading of Cd. Water and ions can also enter roots directly via a low resistance, apoplastic pathway, bypassing the endodermal barrier at sites of emerging lateral roots or mechanical damage. Experiments on apoplastic flow through roots of red pine, Pinus resinosa Ait. (Hanson et al., 1985), and mangrove, Avicennia marina (Forsk.) Vierh. (Moon et al., 1986), have shown that in healthy roots, apoplastic bypass to the shoots was minimal, but that apoplastic bypass increased if the plant was subject to anaerobic stress (Hanson et al., 1985) or physical damage (Moon et al., 1986).
Transpiration as a controller of Cd accumulation in the shoot has been investigated in several species, either by manipulating transpiration (e.g. with humidity or exogenous abscisic acid) or by observing unperturbed transpiration rates (Hardiman and Jacoby, 1984; Florijn and Van Beusichem, 1993; Salt et al., 1995; Buckley et al., 2000). These investigations have led to equivocal conclusions, suggesting that reported results are quite situation dependent. Part of the equivocation may lie in several studies on a variety of plant species, which have shown that high concentrations of Cd (>0.1 mg l−1) in the nutrient solution supplied to roots induce stomatal closure in leaves and, hence, reduce transpiration (Barceló and Poschenrieder, 1990). These responses are probably a result of acute toxicity, and may not be reliably extrapolated to low-concentration Cd exposure. In some of these studies, the original dose–response curves show that at the lowest dose of Cd, transpiration rates and/or stomatal conductivity actually increased (Kirkham, 1978; Hagemeyer et al., 1986). Low-dose effects of Cd on transpiration and other plant processes that may contribute to variation in accumulation of Cd, such as growth (Wagner, 1993) and long-distance transport (Larsson et al., 2002), suggest that it would be useful to study plant accumulation of Cd further at low Cd exposures in particular, as very high Cd concentrations in soil are not typical of most food cropping systems (Mench, 1998). Finally, it is possible that translocation of Cd from root to shoot (and also its accumulation in roots) may not be closely related to bulk flow of water, as the inner surfaces of xylem vessels are active in ion exchange. The ease of Cd transfer from the root surface to the xylem, and subsequent transport through its conducting cells, can be quantified by calculating the ratio between expected (from transpiration) and observed total Cd in the plants; this ratio indicates whether the Cd accumulation in the plant is dominated by passive mass flow (ratio ≈1), or active accumulation or exclusion of Cd in addition to mass flow (ratio ≠ 1).
The objectives of this experiment were: (i) to determine if low-concentration Cd exposure alters transpiration rates; (ii) to explore whether there is a relationship between transpiration and accumulation of Cd in roots, and its long-distance transport to shoots at all life stages; and (iii) to evaluate the potential contribution of apoplastic bypass to the observed accumulation of Cd in wheat seedling shoots. The low abundance mass isotope 106Cd was used to quantify translocation in plants which had or had not received prior exposure to elemental Cd, i.e. Cd with an abundance of isotopes as typically found in geogenic sources. As Cd accumulation in grain is considered to be a significant entry point into the human food chain for this contaminant, the inclusion of several life stages including grainfill is a novel aspect of this study, as the data have relevance to field-grown crops. The second novel aspect of this study is the linking of transpiration and Cd transport in plants acclimated to chronic Cd concentrations (as field-grown plants would be), as phytochelatins, which are induced by chronic exposure to Cd, have been implicated in transport of Cd from soils to plant shoots. Apoplastic bypass was monitored using the non-toxic fluorescent dye PTS (8-hydroxy-1,3,6-pyrenetrisulphonate). For this study, two cultivars of Triticum turgidum var. durum with markedly different Cd accumulation patterns were used: at maturity, ‘Kyle’ accumulates more Cd in the grain as compared with ‘Arcola’ (Chan and Hale, 2004).
Materials and methods
Plant culture
Seeds were germinated on moistened filter paper in a Petri dish for 39–48 h, then placed in rock wool plugs (mineral fibre insulation; Grodan, Denmark), fitted into Styrofoam discs, which were floated on top of 2.5 l black plastic pots (high density polyethylene, Classic 300, Nursery Supplies) attached to a recirculating aerated hydroponic culture system. Four reservoirs containing 75% strength modified Hoagland's solution (automatically adjusted to maintain the pH between 5.8 and 6.2) each supplied a pair of seven-pot rows; two reservoirs were amended with 0 μg l−1 Cd and the other two with 10 μg l−1 elemental Cd (Chan and Hale, 2004). The composition of the Hoagland's solution was: 0.75 mM NH4H2PO4, 4.5 mM KNO3, 3.0 mM Ca(NO3)2.4H2O, 1.5 mM MgSO4, 34 mM H3BO3, 6.9 mM MnCl2.4H2O, 0.59 mM ZnSO4.H2O, 0.24 mM CuSO4.5H2O, 0.36 mM H2MoO4, 27 mM Fe(NO3)3.9H2O dissolved with 27 mM HEDTA (Hoagland and Arnon, 1950). Solutions were partially replaced daily, and fully replaced weekly. Border plants grown in peat-based commercial potting mix surrounded the hydroponics system. Because of gaps created during harvest at various life stages, border plants did not cover all possible edge effects during growth but, as plants were chosen randomly for harvesting, any edge effect was randomly distributed among the experimental units.
Transpiration and translocation measurements
‘Kyle’ and ‘Arcola’ were both at the tillering stage on the same calendar day (3 weeks after germination), and transpiration and translocation were evaluated in the greenhouse where average temperature and relative humidity were 22 °C and 50%, respectively. Using the criteria of Peterson (1965), each plant in the transpiration/translocation study was evaluated for true life stage, i.e. ‘heading’ meaning that no heads were in flower; and ‘grainfill’ meaning that the grains released milky white fluid when punctured. Heading and grainfill occurred on different days for the two cultivars, so transpiration and translocation were measured in a growth chamber (22 °C and 50% relative humidity) instead of the greenhouse to ensure uniform conditions for comparison of cultivars within heading and grainfill growth stages. For measurement of transpiration and translocation at each life stage, four or five replicate plants from 0 μg l−1 and 10 μg l−1 elemental Cd were removed from the recirculating system and placed in individual pots containing 75% Hoagland's solution with 10 μg l−1106Cd. Transpiration during the subsequent 9 h was measured by the weight difference method, evaporative loss from pots with no plants being measured as a control. At the end of this period, roots and shoots, as well as heads and grain at later life stages, were collected separately (roots were patted dry of exposure solution) and dried for 48 h at 70 °C, then weighed in preparation for elemental and 106Cd analyses. Following Cd determinations in tissues, the mass of 106Cd expected in the plant (if volume of mass flow was the only controller of Cd accumulation) was calculated as the product of volume of water transpired and concentration of 106Cd in the root solution. This value was compared with the observed (sum of 106Cd masses in the roots and shoot of each plant) as a ratio. Testing conditions of the tillering plants (greenhouse) versus heading and grainfill plants (growth chamber) may have been slightly different, and this variation could have influenced transpiration. To avoid this bias, most of the discussion of the transpiration data is of 106Cd accumulation relative to transpired volume, measured in the same plant.
Apoplastic bypass measurement
Apoplastic bypass experiments were conducted on seedling-stage plants only, under similar greenhouse conditions to those listed above. Instead of a recirculating system, these small plants were grown in cups that had nutrient solutions renewed at regular intervals. Six-day-old plants were exposed to Cd, the nutrient solution being replaced with 75% strength Hoagland's solution containing either 0 μg l−1 or 8.33 μg l−1106Cd (7.41×10−5 mM, measured value) for 21 h. All plants exposed to Cd were mechanically disturbed by transferring the seedlings to the Cd exposure solution without physical support. During the 21 h exposure, transpiration was measured by the weight difference method, as described earlier. Root systems of 12-d-old plants were exposed to PTS: nutrient solution was replaced with Hoagland's solution supplemented with 1.907 mM PTS (0.1%), with or without mechanical disturbance, for 24 h. For plants in the ‘undisturbed’ treatment, hydraulic support of the root system was maintained by removing only 14% of the total volume of the cup and adding concentrated PTS solution to achieve the desired final PTS concentration. Plants in the ‘disturbed’ treatment were lifted out of the nutrient solution and placed in cups containing 0.1% PTS. Control plants were mechanically disturbed, and not exposed to PTS. PTS was extracted from leaves using a procedure modified from Cholewa and Peterson (2001). Tissue was cut on wax paper into 1–2 mm segments using a razor blade, and segments transferred to a vial. Aliquots of 5 ml of 80% methanol were added, and the vial was boiled in a water bath for 10 min. When cooled, 10 ml of chloroform were added, and the mixture was shaken for 10 min. Then 5 ml of water were added, and the mixture was shaken and allowed to settle; the aqueous phase contained the PTS, which was quantified using a spectrofluorimeter (Photon Technology Inc., South Brunswick, NJ, USA) and Felix software (Photon Technology, Inc.), excitation λ=405 nm and emission λ=510 nm.
Plant digestion and Cd analysis
Plant tissues were digested using hot nitric acid (trace metal grade) and closed Teflon vessels (Topper and Kotuby-Amacher, 1990), either whole (tillering plants) or after grinding in a Wiley-Mill (mesh #40) and subsampling, for heading and grainfill plants. The mass of dry tissue digested never exceeded 1 g, to which 10 ml of acid were added, and the final volume of which (after dilution with water) was 25 ml. For smaller masses of tissue, the volumes of acid and water were scaled accordingly. A standard reference material (SRM) [either 1547 (peach leaves) or 1570a (spinach leaves), National Institute of Standards and Technology] was included with each digestion run to quantify recovery of Cd, and a method blank was included to quantify background Cd. Recovery of Cd from the SRM was routinely >90%. 106Cd was quantified by inductively coupled plasma mass spectroscopy (ICP-MS) with a quadrupole mass analyser (ICP-MS; Elan 6100, Perkin Elmer SCIEX). ICP-MS multielement standards (ICP Analytical Mixture 3 and plasmCal) were analysed with each run of the experimental samples to evaluate analytical interference, none of which was detected. Quality control and instrument drift were monitored using a yttrium internal standard measured at regular intervals throughout sample analysis. To determine elemental Cd concentration, 111Cd was measured as an individual isotope, and then scaled up to its relative abundance (12.80%). All 106Cd data were corrected for the 106 isotope contribution from elemental Cd (1.25%) by subtracting the mass of elemental Cd×0.0125 from the measured mass of 106Cd in that sample.
Experimental design and statistical analysis
The translocation/transpiration experiment was a full factorial, with cultivar (‘Kyle’ or ‘Arcola’), whole-life elemental Cd exposure (0 μg l−1 or 10 μg l−1), and life stage (tillering, heading, or grainfill) as the factors; for each of these factorial combinations, there were four or five experimental units. Plants were grown in a randomized complete block design in the hydroponics system, with elemental Cd concentration blocked on row. For transpiration/translocation measurements, the plants were arranged in a completely randomized design. The apoplastic bypass experiment was an incomplete factorial in a completely randomized design; only the PTS-treated plants were either disturbed or undisturbed, whereas the control (i.e. no PTS, no Cd) plants were disturbed, as were the plants exposed to Cd only. For each experiment reported in this study, there was significant heterogeneity of variance that could not be remedied by transformation of the data; thus, a t-test for unequal variances was used to detect differences between pairs of treatments. In all statistical tests, the critical P-value was 0.05; throughout this report, identification of an effect is made only when the P-value for its test was ≤0.05.
Results
Transpiration and translocation
‘Arcola’ had similar root dry weights, but greater shoot dry weight than ‘Kyle’ at tillering and grainfill; head dry weight was greater in ‘Arcola’ than in ‘Kyle’, and none of these variables was influenced by lifetime exposure to Cd (Table 1). At tillering, the transpiration rate of ‘Kyle’, but not ‘Arcola’, was increased by continuous exposure to elemental (10 μg l−1) Cd in the nutrient solution (Table 1). Apparent transpiration rates were higher in tillering plants than in plants at heading or grainfill (Table 1), probably reflecting the increasing proportion of stems and heads in shoot dry weight at the latter life stages.
Plant biomass (mg plant−1), transpiration rate (ml H2O g−1 shoot DW h−1), and coefficient of variation (%, calculated as the ratio of standard deviation to its mean) for means of tissue Cd and 106Cd concentrations (μg g−1 shoot DW)
Biomass | ‘Kyle’ | ‘Arcola’ | ||||||||||
0 μg l−1 Cd | 10 μg l−1 Cd | 0 μg l−1 Cd | 10 μg l−1 Cd | |||||||||
t | h | g | t | h | g | t | h | g | t | h | g | |
Root DW | 0.45 (0.05) | 3.0 (0.41) | 5.0 (1.3) | 0.4 (0.08) | 2.31 (0.46) | 4.76 (1.3) | 0.43 (0.04) | 2.9 (0.47) | 4.9 (2.4) | 0.37 (0.02) | 3.2 (0.75) | 4.7 (1.6) |
Shoot W | 2.58 (0.45) | 44.7 (3.2) | 74.6 (19) | 2.56 (0.39) | 40.7 (9.1) | 66.8 (13) | 3.0 (0.19) | 72.6 (11.4) | 96.3 (22) | 2.8 (0.33) | 76.9 (19) | 85.6 (14) |
Head W | n/a | 1.75 (0.48) | n/a | n/a | 1.20 (0.62) | n/a | n/a | 3.7 (1.8) | n/a | n/a | 8.0 (3.5) | n/a |
Transpiration | 3.55 (0.14) | 1.29 (0.06) | 1.01 (0.11) | 4.3 (0.18) | 1.5 (0.05) | 1.1 (0.06) | 3.7 (0.14) | 1.01 (0.02) | 0.69 (0.07) | 3.44 (0.21) | 0.94 (0.11) | 0.71 (0.06) |
CV | ||||||||||||
Root 106Cd | 34 | 8.8 | 6.4 | 63 | 27 | 38 | 18 | 5.7 | 11 | 13 | 20 | 17 |
Shoot 106Cd | 27 | 34 | 62 | 11 | 36 | 32 | 13 | 23 | 50 | 23 | 59 | 52 |
Root Cd | 20 | 10 | 18 | 6.4 | 21 | 21 | 5.3 | 15 | 36 | 14 | 31 | 45 |
Shoot Cd | 26 | 15 | 16 | 10 | 14 | 14 | 66 | 19 | 14 | 12 | 8.5 | 19 |
Biomass | ‘Kyle’ | ‘Arcola’ | ||||||||||
0 μg l−1 Cd | 10 μg l−1 Cd | 0 μg l−1 Cd | 10 μg l−1 Cd | |||||||||
t | h | g | t | h | g | t | h | g | t | h | g | |
Root DW | 0.45 (0.05) | 3.0 (0.41) | 5.0 (1.3) | 0.4 (0.08) | 2.31 (0.46) | 4.76 (1.3) | 0.43 (0.04) | 2.9 (0.47) | 4.9 (2.4) | 0.37 (0.02) | 3.2 (0.75) | 4.7 (1.6) |
Shoot W | 2.58 (0.45) | 44.7 (3.2) | 74.6 (19) | 2.56 (0.39) | 40.7 (9.1) | 66.8 (13) | 3.0 (0.19) | 72.6 (11.4) | 96.3 (22) | 2.8 (0.33) | 76.9 (19) | 85.6 (14) |
Head W | n/a | 1.75 (0.48) | n/a | n/a | 1.20 (0.62) | n/a | n/a | 3.7 (1.8) | n/a | n/a | 8.0 (3.5) | n/a |
Transpiration | 3.55 (0.14) | 1.29 (0.06) | 1.01 (0.11) | 4.3 (0.18) | 1.5 (0.05) | 1.1 (0.06) | 3.7 (0.14) | 1.01 (0.02) | 0.69 (0.07) | 3.44 (0.21) | 0.94 (0.11) | 0.71 (0.06) |
CV | ||||||||||||
Root 106Cd | 34 | 8.8 | 6.4 | 63 | 27 | 38 | 18 | 5.7 | 11 | 13 | 20 | 17 |
Shoot 106Cd | 27 | 34 | 62 | 11 | 36 | 32 | 13 | 23 | 50 | 23 | 59 | 52 |
Root Cd | 20 | 10 | 18 | 6.4 | 21 | 21 | 5.3 | 15 | 36 | 14 | 31 | 45 |
Shoot Cd | 26 | 15 | 16 | 10 | 14 | 14 | 66 | 19 | 14 | 12 | 8.5 | 19 |
Values in parentheses are the standard deviation. t, tillering; h, heading; g, grainfill.
Plant biomass (mg plant−1), transpiration rate (ml H2O g−1 shoot DW h−1), and coefficient of variation (%, calculated as the ratio of standard deviation to its mean) for means of tissue Cd and 106Cd concentrations (μg g−1 shoot DW)
Biomass | ‘Kyle’ | ‘Arcola’ | ||||||||||
0 μg l−1 Cd | 10 μg l−1 Cd | 0 μg l−1 Cd | 10 μg l−1 Cd | |||||||||
t | h | g | t | h | g | t | h | g | t | h | g | |
Root DW | 0.45 (0.05) | 3.0 (0.41) | 5.0 (1.3) | 0.4 (0.08) | 2.31 (0.46) | 4.76 (1.3) | 0.43 (0.04) | 2.9 (0.47) | 4.9 (2.4) | 0.37 (0.02) | 3.2 (0.75) | 4.7 (1.6) |
Shoot W | 2.58 (0.45) | 44.7 (3.2) | 74.6 (19) | 2.56 (0.39) | 40.7 (9.1) | 66.8 (13) | 3.0 (0.19) | 72.6 (11.4) | 96.3 (22) | 2.8 (0.33) | 76.9 (19) | 85.6 (14) |
Head W | n/a | 1.75 (0.48) | n/a | n/a | 1.20 (0.62) | n/a | n/a | 3.7 (1.8) | n/a | n/a | 8.0 (3.5) | n/a |
Transpiration | 3.55 (0.14) | 1.29 (0.06) | 1.01 (0.11) | 4.3 (0.18) | 1.5 (0.05) | 1.1 (0.06) | 3.7 (0.14) | 1.01 (0.02) | 0.69 (0.07) | 3.44 (0.21) | 0.94 (0.11) | 0.71 (0.06) |
CV | ||||||||||||
Root 106Cd | 34 | 8.8 | 6.4 | 63 | 27 | 38 | 18 | 5.7 | 11 | 13 | 20 | 17 |
Shoot 106Cd | 27 | 34 | 62 | 11 | 36 | 32 | 13 | 23 | 50 | 23 | 59 | 52 |
Root Cd | 20 | 10 | 18 | 6.4 | 21 | 21 | 5.3 | 15 | 36 | 14 | 31 | 45 |
Shoot Cd | 26 | 15 | 16 | 10 | 14 | 14 | 66 | 19 | 14 | 12 | 8.5 | 19 |
Biomass | ‘Kyle’ | ‘Arcola’ | ||||||||||
0 μg l−1 Cd | 10 μg l−1 Cd | 0 μg l−1 Cd | 10 μg l−1 Cd | |||||||||
t | h | g | t | h | g | t | h | g | t | h | g | |
Root DW | 0.45 (0.05) | 3.0 (0.41) | 5.0 (1.3) | 0.4 (0.08) | 2.31 (0.46) | 4.76 (1.3) | 0.43 (0.04) | 2.9 (0.47) | 4.9 (2.4) | 0.37 (0.02) | 3.2 (0.75) | 4.7 (1.6) |
Shoot W | 2.58 (0.45) | 44.7 (3.2) | 74.6 (19) | 2.56 (0.39) | 40.7 (9.1) | 66.8 (13) | 3.0 (0.19) | 72.6 (11.4) | 96.3 (22) | 2.8 (0.33) | 76.9 (19) | 85.6 (14) |
Head W | n/a | 1.75 (0.48) | n/a | n/a | 1.20 (0.62) | n/a | n/a | 3.7 (1.8) | n/a | n/a | 8.0 (3.5) | n/a |
Transpiration | 3.55 (0.14) | 1.29 (0.06) | 1.01 (0.11) | 4.3 (0.18) | 1.5 (0.05) | 1.1 (0.06) | 3.7 (0.14) | 1.01 (0.02) | 0.69 (0.07) | 3.44 (0.21) | 0.94 (0.11) | 0.71 (0.06) |
CV | ||||||||||||
Root 106Cd | 34 | 8.8 | 6.4 | 63 | 27 | 38 | 18 | 5.7 | 11 | 13 | 20 | 17 |
Shoot 106Cd | 27 | 34 | 62 | 11 | 36 | 32 | 13 | 23 | 50 | 23 | 59 | 52 |
Root Cd | 20 | 10 | 18 | 6.4 | 21 | 21 | 5.3 | 15 | 36 | 14 | 31 | 45 |
Shoot Cd | 26 | 15 | 16 | 10 | 14 | 14 | 66 | 19 | 14 | 12 | 8.5 | 19 |
Values in parentheses are the standard deviation. t, tillering; h, heading; g, grainfill.
Elemental Cd concentration in roots was similar for ‘Arcola’ and ‘Kyle’, and was higher at tillering than at heading and grainfill (Fig. 1a). Elemental Cd concentrations in shoots of ‘Arcola’ were approximately one-half of those in ‘Kyle’ (Fig, 1b). At tillering, pre-exposure to elemental Cd enhanced accumulation of 106Cd in roots of ‘Arcola’ approximately 2-fold during the 9 h exposure (Fig. 2a). However, the accumulation of 106Cd in the roots of ‘Kyle’ was not different for pre-exposed plants (Fig. 2b). Transpiration rates of the pre- and non-pre-exposed plants during this 9 h exposure (x-axes of Figs 1 and 2) were more similar for ‘Arcola’ than for ‘Kyle’, confirming that Cd accumulation in roots is a result of diffusion into the root from the soil solution more than a result of bulk flow. In contrast to the accumulation of 106Cd in roots, during the same period there was very little translocation to the shoots in ‘Arcola’ (as indicated by 106Cd accumulation) and no difference between pre- and non-pre-exposed plants (Fig. 3a). In contrast to ‘Arcola’, shoots of ‘Kyle’ plants that had been pre-exposed to elemental Cd accumulated 106Cd during the 9 h exposure, whereas shoots of the näive plants did not (Fig. 3b).
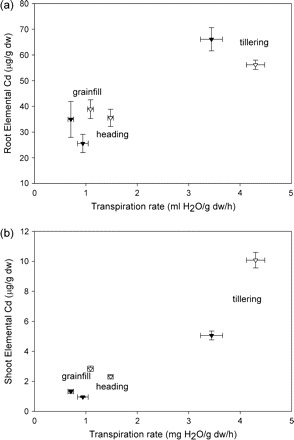
Elemental Cd in roots (a) and shoots (b) of Triticum turgidum cv. Arcola (filled triangles) and Kyle (open triangles) that had been exposed continuously to elemental Cd prior to the study. Each symbol is a mean value of the observed transpiration rate and the concomitant Cd concentration; the horizontal standard error (SE) bar is for transpiration, and the vertical SE bar is for Cd concentration.
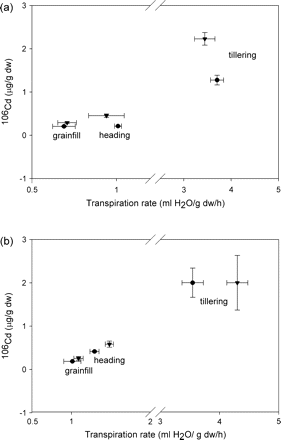
106Cd concentrations in roots of Triticum turgidum cv. Arcola (a) and Kyle (b) at tillering, heading, and grainfill life stages for plants that had been pre-exposed (triangles) or not pre-exposed (circles) continuously to elemental Cd prior to the study. Each symbol is a mean value of the observed transpiration rate and the concomitant Cd concentration; the horizontal standard error (SE) bar is for transpiration, and the vertical SE bar is for Cd concentration.
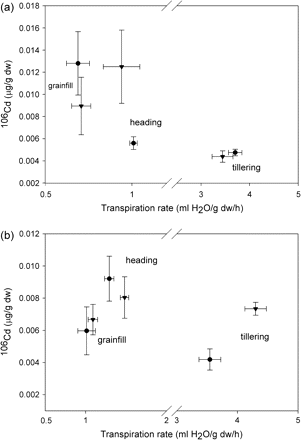
106Cd concentrations in shoots of Triticum turgidum cv. Arcola (a) and Kyle (b) at tillering, heading, and grainfill life stages for plants that had been pre-exposed (triangles) or not pre-exposed (circles) continuously to elemental Cd prior to the study. Each symbol is a mean value of the observed transpiration rate and the concomitant Cd concentration; the horizontal standard error (SE) bar is for transpiration, and the vertical SE bar is for Cd concentration.
Root 106Cd concentration at heading and grainfill was increased by pre-exposure in ‘Arcola’ (Fig. 2a) but not ‘Kyle’ (Fig. 2b). Concentrations of 106Cd in shoots at heading and grainfill after the 9 h exposure period were not different between cultivars or pre-exposures. The standard deviation of these means tended to be greater relative to the mean than at the tillering stage, and much more variable than for root 106Cd concentrations, thus differences among treatments were difficult to detect. This is attributed in part to the lower concentrations of 106Cd in shoots than roots, i.e. a reduced signal:noise ratio, and probably in part to greater asynchrony of plants within these two later development stages, as only approximately half of the plants in each group were at the specific developmental stage (Table 2).
Percentage plants satisfying Peterson's (1965) criteria for developmental stage (n=4 or 5 for each mean)
‘Arcola’ | ‘Kyle’ | |||
106Cd | 106Cd+elemental Cd | 106Cd | 106Cd+elemental Cd | |
Tillering | 100 | 100 | 100 | 100 |
Heading | 79 | 54 | 87 | 66 |
Grainfill | 53 | 74 | 77 | 71 |
‘Arcola’ | ‘Kyle’ | |||
106Cd | 106Cd+elemental Cd | 106Cd | 106Cd+elemental Cd | |
Tillering | 100 | 100 | 100 | 100 |
Heading | 79 | 54 | 87 | 66 |
Grainfill | 53 | 74 | 77 | 71 |
Percentage plants satisfying Peterson's (1965) criteria for developmental stage (n=4 or 5 for each mean)
‘Arcola’ | ‘Kyle’ | |||
106Cd | 106Cd+elemental Cd | 106Cd | 106Cd+elemental Cd | |
Tillering | 100 | 100 | 100 | 100 |
Heading | 79 | 54 | 87 | 66 |
Grainfill | 53 | 74 | 77 | 71 |
‘Arcola’ | ‘Kyle’ | |||
106Cd | 106Cd+elemental Cd | 106Cd | 106Cd+elemental Cd | |
Tillering | 100 | 100 | 100 | 100 |
Heading | 79 | 54 | 87 | 66 |
Grainfill | 53 | 74 | 77 | 71 |
In roots, higher transpiration rates (i.e. greater volume of mass flow) were positively related to higher lifetime elemental Cd accumulation, and, with higher 106Cd accumulation over the 9 h period of transpiration measurement (Figs 1, 2). Shoot data were dissimilar to those for roots: while the transpiration rate during the 9 h exposure to 106Cd was consistent with lifetime elemental Cd accumulation in shoots for both ‘Kyle’ and ‘Arcola’, it was not consistent with 106Cd accumulation in shoots during that same period (Figs 1, 3). The ratio between expected (from transpiration) and observed total 106Cd was mostly higher for heading than for tillering, and pre-exposure to elemental Cd reduced the ratio in ‘Arcola’ at the heading stage, but not in Kyle (Table 3). For ‘Arcola’ at heading, transpiration overpredicted accumulation of 106Cd in non-pre-exposed plants 4-fold, whereas in the pre-exposed plants, transpiration predicted accumulation very well (Table 3). At heading and grainfill, uptake of 106Cd into roots and transpiration rates were both much lower than at tillering, suggesting that Cd uptake and transpiration are correlated, although there were too few (and bunched) pairs of observations for calculating the coefficient of determination. However, the effects of pre-exposure did not support this correlation: pre-exposure sometimes enhanced Cd accumulation or transpiration, but not in a pattern that could be attributed to cause and effect.
‘Kyle’ | ‘Arcola’ | |||||||
0 μg l−1 Cd | 10 μg l−1 Cd | 0 μg l−1 Cd | 10 μg l−1 Cd | |||||
t | h | t | h | t | h | t | h | |
Ratio | 0.67 (0.17) | 2.32 (0.43) | 0.99 (0.53) | 1.86 (0.50) | 1.30 (0.13) | 4.7 (0.51) | 0.76 (0.33) | 1.08 (0.70) |
‘Kyle’ | ‘Arcola’ | |||||||
0 μg l−1 Cd | 10 μg l−1 Cd | 0 μg l−1 Cd | 10 μg l−1 Cd | |||||
t | h | t | h | t | h | t | h | |
Ratio | 0.67 (0.17) | 2.32 (0.43) | 0.99 (0.53) | 1.86 (0.50) | 1.30 (0.13) | 4.7 (0.51) | 0.76 (0.33) | 1.08 (0.70) |
Values in parentheses are the standard deviation. t, tillering; h, heading.
‘Kyle’ | ‘Arcola’ | |||||||
0 μg l−1 Cd | 10 μg l−1 Cd | 0 μg l−1 Cd | 10 μg l−1 Cd | |||||
t | h | t | h | t | h | t | h | |
Ratio | 0.67 (0.17) | 2.32 (0.43) | 0.99 (0.53) | 1.86 (0.50) | 1.30 (0.13) | 4.7 (0.51) | 0.76 (0.33) | 1.08 (0.70) |
‘Kyle’ | ‘Arcola’ | |||||||
0 μg l−1 Cd | 10 μg l−1 Cd | 0 μg l−1 Cd | 10 μg l−1 Cd | |||||
t | h | t | h | t | h | t | h | |
Ratio | 0.67 (0.17) | 2.32 (0.43) | 0.99 (0.53) | 1.86 (0.50) | 1.30 (0.13) | 4.7 (0.51) | 0.76 (0.33) | 1.08 (0.70) |
Values in parentheses are the standard deviation. t, tillering; h, heading.
PTS and transpiration
The Cd and PTS experiments were performed at different times, probably under slightly different environmental conditions; thus, accumulations of Cd and PTS were normalized for transpiration volume and external exposure concentration, by calculation of the transpiration stream concentration factor (TSCF) for each. Using spectrofluorimetry, PTS was detected in five of the eight plants exposed to PTS, and was similar in both number of plants and concentration in plant tissue for ‘disturbed’ and ‘undisturbed’ plants (Table 4); for the remaining three plants, no spectrofluorimetry peak typical of PTS (at 510 nm) was observed (Table 4). The calculated TSCF was not different for ‘undisturbed’ and ‘disturbed’ plants (Table 4). The TSCF values for 106Cd in the disturbed plants (7.0×10−2) as compared with those for PTS (3.4–4.1×10−4) indicate that apoplastic bypass would account for <1% of the Cd in the transpiration stream.
PTS or Cd concentration in leaf tissue and transpiration stream, and transpiration stream concentration factor (TSCF) for PTS or Cd.
No. of plants with detectable PTS or Cd | Concentration of PTS or Cd in leaves (nmol g FW−1) | Concentration of PTS or Cd in transpiration stream (nmol l−1) | TSCF | |
Undisturbed+PTS | 3/4 | 13.5 (0.6) | 7.8(0.5)×10−2 | 4.1 (0.3)×10−4 |
Disturbed+PTS | 2/4 | 12.2(0.9) | 6.5 (1.5)×10−2 | 3.4 (0.5)×10−4 |
Disturbed+106Cd | 4/4 | 29.0 (5.0)×10−3 | 5.18 (0.79) | 7.0 (0.1)×10−2 |
No. of plants with detectable PTS or Cd | Concentration of PTS or Cd in leaves (nmol g FW−1) | Concentration of PTS or Cd in transpiration stream (nmol l−1) | TSCF | |
Undisturbed+PTS | 3/4 | 13.5 (0.6) | 7.8(0.5)×10−2 | 4.1 (0.3)×10−4 |
Disturbed+PTS | 2/4 | 12.2(0.9) | 6.5 (1.5)×10−2 | 3.4 (0.5)×10−4 |
Disturbed+106Cd | 4/4 | 29.0 (5.0)×10−3 | 5.18 (0.79) | 7.0 (0.1)×10−2 |
Values in parentheses are the standard error. The TSCF for PTS or Cd was calculated by dividing the calculated transpiration stream concentration by the concentration in the external solution (1.907 mM PTS or 7.41×10−5 mM 106Cd).
PTS or Cd concentration in leaf tissue and transpiration stream, and transpiration stream concentration factor (TSCF) for PTS or Cd.
No. of plants with detectable PTS or Cd | Concentration of PTS or Cd in leaves (nmol g FW−1) | Concentration of PTS or Cd in transpiration stream (nmol l−1) | TSCF | |
Undisturbed+PTS | 3/4 | 13.5 (0.6) | 7.8(0.5)×10−2 | 4.1 (0.3)×10−4 |
Disturbed+PTS | 2/4 | 12.2(0.9) | 6.5 (1.5)×10−2 | 3.4 (0.5)×10−4 |
Disturbed+106Cd | 4/4 | 29.0 (5.0)×10−3 | 5.18 (0.79) | 7.0 (0.1)×10−2 |
No. of plants with detectable PTS or Cd | Concentration of PTS or Cd in leaves (nmol g FW−1) | Concentration of PTS or Cd in transpiration stream (nmol l−1) | TSCF | |
Undisturbed+PTS | 3/4 | 13.5 (0.6) | 7.8(0.5)×10−2 | 4.1 (0.3)×10−4 |
Disturbed+PTS | 2/4 | 12.2(0.9) | 6.5 (1.5)×10−2 | 3.4 (0.5)×10−4 |
Disturbed+106Cd | 4/4 | 29.0 (5.0)×10−3 | 5.18 (0.79) | 7.0 (0.1)×10−2 |
Values in parentheses are the standard error. The TSCF for PTS or Cd was calculated by dividing the calculated transpiration stream concentration by the concentration in the external solution (1.907 mM PTS or 7.41×10−5 mM 106Cd).
Discussion
The main purpose of this study was to identify the role of transpiration in differential accumulation of Cd among cultivars of durum wheat which are known to differ in accumulation of Cd in grain, and to determine how this role was influenced by pre-exposure to low concentrations of Cd. Barceló and Poschenrieder (1990) suggest that the mechanism of stimulated transpiration could be that toxicity to the roots but not shoots causes increased sucrose in leaves due to reduced root growth (thus reduced root sink). The increased leaf sucrose would lead to higher turgor, and thus lower stomatal resistance and higher transpiration; this mechanism is consistent with the present study, which suggests that ‘Kyle’ (but not ‘Arcola’) is sensitive to low-concentration Cd exposure as indicated by increased transpiration, but not growth reduction. The associated enhanced Cd accumulation in ‘Kyle’ might be the result of toxicity in the form of perturbed transpiration, at solution Cd concentrations similar to those which might be experienced in field soil solutions (Ingwersen and Streck, 2005). Kirkham (1978) similarly demonstrated that 10 μg l−1 Cd increased the transpiration rate of chrysanthemum (Chrysanthemum morifolium Ramat. ‘Indianapolis White’), whereas concentrations greater than this suppressed transpiration. These authors linked increased transpiration with increased turgor and decreased stomatal resistance, and suggested that such physiological changes might be the bases for the occasional report of growth stimulation by a non-essential element, for example, in the study of Miscanthus sinensis L. var. Giganteus (Arduini et al., 2004). At higher exposure concentrations of Cd, the response of transpiration is reversed: Hagemeyer et al. (1986) demonstrated marked reduction in transpiration of Fagus sylvatica L. (beech) exposed to 5, 25, or 75 mg l−1 Cd, at pH 3.5, concentrations (as well as percentage of total Cd in the cationic, thus bioavailable, form) which are far greater than those used in the present study. Similarly, Haag-Kerwer et al. (1999) reported that transpiration of Brassica juncea was reduced when plants were exposed to 25 μM CdNO3, a very high concentration relative to those shown to stimulate transpiration. This study further showed that while exposure to this concentration of Cd did not result in reduced photosynthesis, reduced transpiration was correlated with reduced Cd accumulation, and a reduced leaf expansion rate. Another possible explanation of the variation in transpiration rates in response to Cd treatment that has, to date, not been tested is that this metal influences the quantity or activity of aquaporins in the roots, thus regulating the resistance to water flow in the plant. (For further information, see review by Tyerman et al., 2002.)
The second objective of the present study was to examine the relationship between transpiration and translocation of Cd in plants acclimated to Cd through pre-exposure; in this study, this relationship was complex (Table 5). Numerous studies have demonstrated the induction of phytochelatins in response to Cd exposure, and their role in detoxification is considered a key adaptative strategy (Rauser, 1995, and citations therein; Haag-Kerwer et al., 1999). Larsson et al. (2002) demonstrated that a phytochelatin-deficient mutant of Arabidopsis thaliana translocated less 109Cd from root to shoot than the wild type, and that exposure to elemental Cd (0.25–1.0 μM) during growth increased translocation of 109Cd in the wild type in comparison with 0 μM elemental Cd during growth. These authors suggest that induction of phytochelatin plays a key role in translocation of Cd from roots to shoots. This mechanism could co-exist with the toxicity/water relations hypothesis of Barceló and Poschenrieder (1990). The existence of multiple controllers for Cd accumulation by plants is suggested by the work of Ingwersen and Streck (2005) who modelled Cd accumulation in field crops relative to Cd concentration in the soil solution, and explained between 66% and 87% of this relationship by the amount of water transpired. This hypothesis of disjunct between transpiration volume and Cd accumulation was noted earlier by Hardiman and Jacoby (1984), who measured the accumulation of 109Cd from nutrient solution in Phaseolus vulgaris under low and high humidities, and found that the concentrations of Cd in the transpiration stream were similar regardless of the transpiration rate. Also, Cd is known to be phloem mobile (a process not directly dependent on transpiration) which is the mechanism by which it moves to the grain (Jalil et al., 1994; Hart et al., 1998; Harris and Taylor, 2001). The data in the present study well support that more than one physiological process controls Cd translocation from soil solution to plant tissues: scrutiny of the ratio between expected and observed 106Cd accumulation suggested that any effects of pre-exposure to elemental Cd on transpiration and 106Cd accumulation were more consistent with each other for ‘Kyle’ than for ‘Arcola’. In complete contrast to the apparent enhancement of transpiration and concomitant root to shoot translocation of Cd by pre-exposure to Cd in ‘Kyle’, the capacity of ‘Arcola’ roots to sequester Cd, and limit root to shoot translocation, was enhanced by pre-exposure to Cd (Table 5).
Summary of changes in physiological parameters resulting from pre-exposure to Cd
‘Kyle’ | ‘Arcola’ | |
Transpiration | ↑ at tillering | 0 |
106Cd accumulation in roots | 0 | ↑ |
106Cd accumulation in shoots | ↑ at tillering | 0 |
Expected versus observed total plant 106Cd | 0 | ↓ at heading |
Root dry weight | 0 | 0 |
Shoot dry weight | 0 | 0 |
‘Kyle’ | ‘Arcola’ | |
Transpiration | ↑ at tillering | 0 |
106Cd accumulation in roots | 0 | ↑ |
106Cd accumulation in shoots | ↑ at tillering | 0 |
Expected versus observed total plant 106Cd | 0 | ↓ at heading |
Root dry weight | 0 | 0 |
Shoot dry weight | 0 | 0 |
Summary of changes in physiological parameters resulting from pre-exposure to Cd
‘Kyle’ | ‘Arcola’ | |
Transpiration | ↑ at tillering | 0 |
106Cd accumulation in roots | 0 | ↑ |
106Cd accumulation in shoots | ↑ at tillering | 0 |
Expected versus observed total plant 106Cd | 0 | ↓ at heading |
Root dry weight | 0 | 0 |
Shoot dry weight | 0 | 0 |
‘Kyle’ | ‘Arcola’ | |
Transpiration | ↑ at tillering | 0 |
106Cd accumulation in roots | 0 | ↑ |
106Cd accumulation in shoots | ↑ at tillering | 0 |
Expected versus observed total plant 106Cd | 0 | ↓ at heading |
Root dry weight | 0 | 0 |
Shoot dry weight | 0 | 0 |
The third objective of this study was to quantify the potential contribution of apoplastic bypass to Cd accumulation in the shoots. The present study demonstrates that an apoplastic bypass exists in most durum wheat seedlings grown in hydroponic culture. However, comparison of TSCF for PTS and Cd suggests that such a bypass would make a very small contribution to the accumulation of Cd in plant shoots, and that it was not enhanced by the mechanical disturbance. This simple comparison of TSCFs for Cd and PTS assumes that PTS movement in the apoplastic bypass is the same as that of Cd. However, PTS is much larger than atomic Cd; the formula weights of PTS and Cd are 524 amu and 112 amu, respectively, and the diameters are 1.1 nm (Moon et al., 1986) and 0.19 nm (Henderson, 1982), respectively. It can be assumed that a pathway with channels of sufficient size to allow the passage of a large dye molecule would be accessible to smaller, physiologically relevant molecules (Peterson et al., 1981). The size difference suggests that PTS apoplastic flow could be an underestimation of Cd apoplastic flow. If PTS apoplastic flow underestimates this bypass by 5-fold or 10-fold, for example, the contribution of apoplastic Cd would still remain minimal (2.7% or 5.4%, respectively). It is also possible that negatively charged PTS overestimates the role of apoplastic flow in shoot accumulation of Cd, as cations are known to interact with negative charges in cell walls, including those of the xylem vessels, whereas anions do not. Lack of any significant apoplastic flow indicates that the majority of Cd follows a symplastic pathway into the stele of the root, and that cellular influx and efflux of Cd would be the main control points for Cd transport. Supporting this conclusion is a study in which Brassica juncea was exposed to EDTA, Cd, and PTS, and ratios of xylem sap concentration:exposure solution concentration of Cd-EDTA and PTS were compared (Schaider et al., 2006). Both ratios were >1, and the ratio for Cd-EDTA was at least 2-fold that for PTS; the authors concluded that apoplastic bypass was not the route by which metal–EDTA complexes were taken up by plants. These studies and the present one confirm, as Marschner (1995) suggested, that small bypasses occur widely and under a variety of plant culture regimes. The disturbance imposed in the present study, while representative of that which occurs in other experiments as part of routine hydroponic culture, was not large enough to increase apoplastic bypass beyond that which occurs normally in roots.
Conclusions
The data in this study demonstrate that differential accumulation of Cd by ‘Kyle’ and ‘Arcola’ could be partially attributed to effects on transpiration: exposure to low concentrations of Cd increased mass flow and concomitant Cd accumulation in ‘Kyle’. Distinct from this, exposure of ‘Arcola’ to low concentrations of Cd reduced translocation of Cd from roots to shoots relative to root accumulation of Cd. It is possible, but not tested here, that sequestration mechanisms (such as phytochelatin production, as demonstrated by others) are the genetically controlled difference between these two cultivars that results in differential Cd accumulation. These results also suggest that apoplastic bypass was not a major pathway of Cd transport from the root to the shoot in these plants, and that most of the Cd ultimately present in the shoot moved into the stele of the root via the symplastic pathway. Movement into the cytoplasm (across the plasma membrane) of epidermal and cortical cells, and out of the cytoplasm (again across the plasma membrane) in the root stele are two additional potential control points for delivery of Cd to the shoot.
The authors thank Ewa Cholewa, Fengshan Ma, and Pamela Collins for assistance with PTS extraction and spectrofluorimetry, fluorescence microscopy, and ICP-MS, respectively.
Comments