-
PDF
- Split View
-
Views
-
Cite
Cite
Shuhei Noda, Takaya Miyazaki, Takanori Miyoshi, Michiru Miyake, Naoko Okai, Tsutomu Tanaka, Chiaki Ogino, Akihiko Kondo, Cinnamic acid production using Streptomyces lividans expressing phenylalanine ammonia lyase, Journal of Industrial Microbiology and Biotechnology, Volume 38, Issue 5, 1 May 2011, Pages 643–648, https://doi.org/10.1007/s10295-011-0955-2
- Share Icon Share
Abstract
Cinnamic acid production was demonstrated using Streptomyces as a host. A gene encoding phenylalanine ammonia lyase (PAL) from Streptomyces maritimus was introduced into Streptomyces lividans, and its expression was confirmed by Western blot analysis. After 4 days cultivation using glucose as carbon source, the maximal level of cinnamic acid reached 210 mg/L. When glycerol (30 g/L) was used as carbon source, the maximal level of produced cinnamic acid reached 450 mg/L. In addition, using raw starch, xylose or xylan as carbon source, the maximal level of cinnamic acid reached 460, 300, and 130 mg/L, respectively. We demonstrated that S. lividans has great potential to produce cinnamic acid as well as other aromatic compounds.
Introduction
To construct a biomass-oriented sustainable society, it is necessary to produce chemicals and fuels from renewable resources such as biomass and industrial waste substances [3]. At present, polymer materials are made of building-block compounds usually made by chemical processes. Although various biomaterial production systems have been developed using a variety of microbes, such as ethanol production using yeasts [4, 12, 27, 28], lactic acid production using lactic acid bacteria [2, 13, 21], and amino acid production using Corynebacterium [9, 19, 22], there are few reports concerning production of aromatic building blocks from biomass.
Cinnamic acid is an aromatic compound widely used as a material for organic thin-film displays or as an antibacterial agent. In nature, cinnamic acid exists in plants as a precursor of various phenylpropanoids such as lignins, flavonoids, and coumarins [10]. Cinnamic acid is chemically produced via a condensation reaction of acetic anhydride and benzaldehyde in the presence of sodium acetate [10]; however, this process is energy intensive. Although cinnamic acid production systems using P. putida or E. coli have been reported previously [10, 23], cinnamic acid productivity still needs to be improved.
Here, we used Streptomyces, which are antibiotic producers having high tolerance for aromatic compounds, as a host for cinnamic acid production. Secondary metabolite pathways in Streptomyces have been widely studied, and Streptomyces have been used to produce various antibiotics [8, 20, 24]. Streptomyces have also been used as hosts for secretory production of heterologous proteins [11, 14, 17]. However, there are few reports concerning low-molecular-weight compound production using Streptomyces.
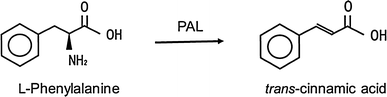
PAL-catalyzed conversion of l-phenylalanine to trans-cinnamic acid
In this work, we focused on S. lividans, which is known as a heterologous protein producer [11, 14, 17]. We introduced PAL from S. maritimus into S. lividans and evaluated cinnamic acid productivity using not only glucose, but also glycerol, which is a potential economical feedstock for fermentation [3]. We also demonstrated direct cinnamic acid production from several kinds of biomass, such as raw starch, xylose, and xylan. One of the advantages of S. lividans is its biomass-assimilating ability, which is suitable for building-block compound production from biomass. This is the first report showing building-block compound production directly from various biomass resources using Streptomyces.
Materials and methods
Plasmid construction
The basal expression vector for the PAL protein in S. lividans as a host was constructed by polymerase chain reaction (PCR) using PrimeSTAR HS (Takara, Shiga, Japan). The gene fragment encoding the promoter region of the PLD open reading frame from Streptoverticillium cinnamoneum [11, 14] was amplified by PCR using the Stv. cinnamoneum genome (NBRC 12852) as a template with the following two primers: 5′-aaaagcttacgtcatggcgggtctctctcgtc-3′ (HindIII-promoter/Fw) and 5′-ttaagcttcccccgtcatgcgccccggaacgtccgggcgctcagtcgtcagatcttgtgcatccttaaacgaagtaacgattccg-3′ (HindIII-terminator-BglII-promoter/Rv). The amplified fragment was subcloned into the HindIII site of pUC18. The vector was named pUC18-prom-BglII-term.
The gene fragment encoding encP from S. maritimus was amplified by PCR using the S. maritimus genome (DSMZ 41777) as a template with the following two primers: 5′-aaagaattcagatctccgtcgagtccaccgattctccggccgcaaagg-3′ (EcoRI-BglII-encP/Fw) and 5′-tttaagcttagatctgcggccgcctcgagaggccttcagtgcgccgccacggcagc-3′ (HindIII-BglII-NotI-XhoI-StuI-encP/Rv). The amplified fragment was subcloned into the HindIII and EcoRI sites of pUC18. The vector was named pUC18-encP. pUC18-encP was digested with BglII, and the digested fragment encoding encP was subcloned into the BglII site of pUC18-prom-BglII-term. The vector was named pUC18-prom-encP-term. Finally, pUC18-prom-encP-term was digested with HindIII, and the digested fragment encoding prom-encP-term was subcloned into the HindIII site of pUC702. The vector was named pUC702-p-encP. The vector for expression of His-tagged encP was constructed as follows. The gene fragment encoding encP-(His)6 was amplified by PCR using pUC18-encP as a template with the flowing primers: 5′-aaaagatctatgaccttcgtcatagagctcgaca-3′ (BglII-encP-(His)6/Fw) and 5′-tttagatcttcagtggtggtggtggtggtggtgcgccgccacggcagcgtcgacg-3′ (BglII-encP-(His)6/Rv). The resultant plasmid was named pUC702-p-encP-(His)6.
Bacterial strain, transformation, and cultivation
Protoplasts of S. lividans 1326 were prepared according to the method of Hopwood et al. [5]. Briefly, mycelium of S. lividans 1326 was treated with 1 mg/mL lysozyme solution (Wako, Osaka, Japan), and the suspended mycelium was used as protoplasts. The plasmids pUC702-p-encP and pUC702-p-encP-(His)6 were introduced by the polyethylene glycol (PEG) method. Selection of transformants was carried out by overlaying soft agar containing 50 μg/mL thiostrepton as antibiotic. After cultivation for 5 days, selected transformants were named S. lividans/p-encP and S. lividans/p-encP-(His)6, respectively.
One spore of S. lividans/p-encP was inoculated in a test-tube containing 5 ml TSB medium [17 g/L pancreatic digest of casein, 3 g/L papaic digest of soybean meal, 2.5 g/L glucose, 5.0 g/L sodium chloride, 2.5 g/L dipotassium phosphate (BD Diagnostic Systems, Sparks, MD, USA)] supplemented with 5 μg/mL thiostrepton (Sigma, St. Louis, MO), followed by cultivation at 28°C for 3 days. Then, 5 ml of the preculture medium was seeded into a shake flask with a baffle containing 100 ml TSB medium with 5 μg/mL thiostrepton, 15 g/L glucose, 15–30 g/L glycerol, 15 g/L raw starch, 15 g/L xylose, 15 g/L xylan from birch wood (Sigma) as carbon source, and 15–50 g/L tryptone as nitrogen source, and incubated at 28°C for 4–8 days.
Western blotting
S. lividans/p-encP was cultured at 28°C for 72 h in 100 ml TSB medium. After cultivation, cells were centrifuged at 21,880×g for 10 min at 4°C. Cells were washed and resuspended in 700 μl phosphate-buffered saline (pH 7.2) containing 1% (vol/vol) Protease Inhibitor Cocktail (Nacalai Tesque, Kyoto, Japan). Cells were then disrupted using a Multi Beads Shocker (Yasuikikai, Osaka, Japan) according to a previously described protocol [22]. The insoluble fraction and glass beads were removed by centrifugation at 21,880×g for 10 min at 4°C. Sodium dodecyl sulfate polyacrylamide gel electrophoresis (SDS–PAGE) loading buffer was added to the supernatant, followed by boiling at 100°C for 5 min. Proteins were analyzed by SDS–PAGE using an SDS–polyacrylamide gel (15%: w/v), after which proteins were electroblotted onto a polyvinylidene difluoride membrane (Millipore Co., Boston, MA, USA) and were allowed to react with primary rabbit anti-(His)6 and secondary goat anti-rabbit immunoglobulin G alkaline-phosphatase-conjugated antibodies (Promega Co., Madison, WI, USA). The membrane was then stained with nitroblue tetrazolium (Promega) and 5-bromo-4-chloro-3-indolylphosphate (Promega) according to the manufacturer’s protocol.
Analytical methods
Cinnamic acid concentration was simultaneously determined by high-performance liquid chromatography (HPLC; Shimadzu, Kyoto, Japan) using a Cholester column (Nacalai Tesque, Kyoto, Japan). The operating conditions were 30°C, acetonitrile:phosphate buffer (50 mM, pH 2.5) (30:70) mobile phase, and flow rate 1.2 ml/min; cinnamic acid concentration was determined using a ultraviolet absorbance detector (Shimadzu SPD-20AV). Culture supernatant was separated from the culture broth by centrifugation at 21,880×g for 20 min and then analyzed by HPLC.
Results and discussion
Expression of encP using S. lividans

Western blot analysis of PAL-(His)6: lane 1: S. lividans/pUC702; lane 2: S. lividans/p-encP-(His)6
Production of cinnamic acid from glucose using S. lividans/p-encP, and optimization of the supplied nitrogen source
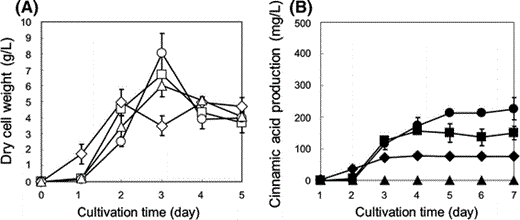
a Time-courses of dry cell weight using glucose as sole carbon source: S. lividans/pUC702 in TSB medium with 15 g/L glucose and 15 g/L tryptone (open triangles); S. lividans/p-encP in TSB medium with 15 g/L glucose, and 15 g/L tryptone (diamonds), 30 g/L tryptone (open squares), and 50 g/L tryptone (open circles). b Time-courses of produced cinnamic acid in culture: S. lividans/pUC702 in TSB medium with 15 g/L glucose and tryptone (closed triangles); S. lividans/p-encP in TSB medium with 15 g/L glucose, and 15 g/L tryptone (closed diamonds), 30 g/L tryptone (closed squares), and 50 g/L tryptone (closed circles). Each data point shows the average of three independent experiments, and error bars represent standard deviation
Figure 3b shows the amount of cinnamic acid produced. S. lividans/p-encP successfully produced about 80 mg/L cinnamic acid from 15 g/L glucose, whereas S. lividans/pUC702 did not produce cinnamic acid at all. Increasing the initial glucose concentration led to low cell growth and lower cinnamic acid productivity (data not shown), and hence 15 g/L glucose was suitable for efficient cinnamic acid production using S. lividans/p-encP.
To enhance cinnamic acid production using S. lividans, we tried to optimize the initial nitrogen source. S. lividans/p-encP was grown in TSB medium with additional 15, 30 or 50 g/L tryptone as nitrogen source. As shown in Fig. 3b, increasing the initial tryptone concentration enhanced cinnamic acid productivity, and the cinnamic acid concentration reached up to 210 mg/L after 5 days cultivation of S. lividans/p-encP with 15 g/L glucose and 50 g/L tryptone. Cinnamic acid productivity was not enhanced when larger amounts of tryptone were used (data not shown). Although initial cell growth and cinnamic acid production rates become slower with increasing initial tryptone concentrations, the maximum dry cell weight and produced cinnamic acid were improved. These results demonstrated that the nitrogen source is a key factor to enhance the production rate and maximal concentration of cinnamic acid.
Production of cinnamic acid from biomass in S. lividans/p-encP, and optimization of the nutrient source concentration
According to our finding that increasing the initial glucose concentration leads to low cell growth and low cinnamic acid productivity, we tried to use glycerol, a potentially economical feedstock for fermentation, as carbon source [3]. Some Streptomyces can utilize glycerol more effectively than glucose [18]; however, the glycerol-assimilating ability of S. lividans had not previously been tested.
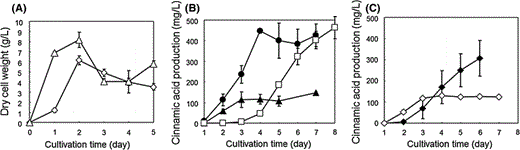
a Time-courses of dry cell weight using glycerol as sole carbon source: S. lividans in TSB medium with 15 g/L glycerol and 15 g/L tryptone (open triangles); S. lividans/p-encP in TSB medium with 15 g/L glycerol and 15 g/L tryptone (open diamonds). b Time-courses of produced cinnamic acid in culture: S. lividans/p-encP in TSB medium with 15 g/L glycerol and 15 g/L tryptone (closed diamonds), 30 g/L glycerol and 50 g/L tryptone (closed circles), and 15 g/L raw starch and 50 g/L tryptone (open squares) c 15 g/L xylose and 50 g/L tryptone (closed diamonds), and 15 g/L xylan and 50 g/L tryptone (open diamonds). Each data point shows the average of three independent experiments, and error bars represent standard deviation
Encouraged by these findings, we tried to carry out cinnamic acid production from xylose or xylan, which represents the most abundant hemicellulosic polysaccharide, using S. lividans/p-encP. Although the initial rate of cinnamic acid production was lower than that with 15 g/L glucose, the maximal amount of produced cinnamic acid from 15 g/L xylose was 300 mg/L, which was 1.5-fold higher than that using glucose. When arabinose was used as sole carbon source, the amount of cinnamic acid produced was lower compared with other carbon sources (data not shown). This result implies that S. lividans also has an only xylose-assimilating pathway, like other Streptomyces species [1, 7, 15]. In addition, the amount of cinnamic acid produced from 15 g/L xylan reached 130 mg/L after 4 days cultivation, due to active-form xylanases produced by S. lividans [6]. This is the first report concerning production of biocompounds from various types of biomass using Streptomyces, and we also demonstrated that S. lividans has a great advantage for as a host for production of biocompounds from various carbon sources due to its glycerol-, xylose-, xylan-, and starch-assimilating abilities. In addition, S. lividans can grow in the presence of more than 1 g/L cinnamic acid, whereas E. coli cannot grow in only 0.1 g/L cinnamic acid (data not shown). This result implies that S. lividans has tolerance to cinnamic acid and is a promising host for cinnamic acid overproduction.
In conclusion, we successfully demonstrated cinnamic acid production using engineered S. lividans along with optimization of the carbon and nitrogen source. To improve cinnamic acid productivity, l-phenylalanine availability in the cell should be enhanced, because addition of small amounts of l-phenylalanine improved cinnamic acid productivity (data not shown). S. lividans can potentially be used as a host to produce various aromatic building-block compounds.
Acknowledgments
This work was supported by the Special Coordination Funds for Promoting Science and Technology, Creation of Innovation Centers for Advanced Interdisciplinary Research Areas (Innovation Bioproduction Kobe), MEXT, Japan.