-
PDF
- Split View
-
Views
-
Cite
Cite
Minna Peltola, Teemu Kuosmanen, Hanna Sinkko, Niina Vesalainen, Martti Pulliainen, Päivi Korhonen, Kirsi Partti-Pellinen, Jari P Räsänen, Juha Rintala, Marko Kolari, Hannu Rita, Mirja Salkinoja-Salonen, Effects of polarization in the presence and absence of biocides on biofilms in a simulated paper machine water, Journal of Industrial Microbiology and Biotechnology, Volume 38, Issue 10, 1 October 2011, Page 1719, https://doi.org/10.1007/s10295-011-0961-4
- Share Icon Share
Abstract
The antifouling potential of electric polarization combined and not combined with biocides was studied in nonsaline warm water with high organic content. Deinococcus geothermalis is a bacterium known for forming colored biofilms in paper machines and for its persistence against cleaning and chemical treatments. When D. geothermalis biofilms grown for 24 h in simulated paper machine water were exposed to cathodic or cathodically weighted pulsed polarization at least 60% (P < 0.05) of the biofilms were removed from stainless steel (AISI 316L). Biofilm removal by 25 ppm (effective substances 5–25 ppm) of oxidizing biocides (bromochloro-5,5-dimethylhydantoin, 2,2-dibromo-2-cyanoacetamide, peracetic acid) increased to 70% when combined with cathodically weighted pulsed polarization. Using a novel instrument that allows real-time detection of reactive oxygen species (ROS) we showed that the polarization program effective in antifouling generated ROS in a pulsed manner on the steel surface. We thus suggest that the observed added value of oxidative biocides combined with polarization depended on ROS. This suggestion was supported by the finding that a reductive biocide, methylene bisthiocyanate, counteracted the antifouling effect of polarization.
Introduction
In warm water-intensive industries biofouling endangers product quality, causes equipment malfunction, corrosion, and economic loss. For biofilm prevention the paper industry depends on biocides and mechanical cleaning. Only a few biocides are approved by paper industries and their clients for the manufacture of sensitive paper products such as food packaging materials. Mechanical cleaning is needed as biocides are ineffective towards pre-existing biofilms and because biomass (dead or alive) provides surfaces and nutrients for the attachment and proliferation of microorganisms. Anodic generation of reactive oxygen species (ROS), shown to be effective in disinfecting planktonic cells [7–9], has gained interest because it leaves no toxic residue. Antifouling strategies beyond the conventional biocidal chemicals are needed.
In saline waters electrochemical polarization can generate biocidally active chlorine species (Cl2, HCl, OCl−, and ClO2) that are most likely responsible for the reported antimicrobial activity and antifouling in marine applications [16, 28]. We found that electrochemical polarization was effective also in brackish Baltic Sea water (3 g NaCl l−1) used as cooling water in heat exchangers of a power plant [13].
Electrochemical polarization was demonstrated to be antimicrobially active in inorganic buffers used to feed flow cells with strains of Escherichia coli, Pseudomonas spp., and Staphylococci adhered on surfaces [1, 2, 4, 6, 7, 26]. Electrochemical generation of ROS has not been reported in waters rich in organic matter, e.g., in water circuits in wet industry, possibly because organic matter may consume the ROS thus reducing its antimicrobial activity. We investigated the antifouling potential of polarization in freshwater-based paper machine water low in chloride but rich in organic matter. As the biofouler we used Deinococcus geothermalis because it forms firm biofilms in paper machine waters [11, 12, 17, 24]. This species is known for its resistance towards most industrial biocides and stressors like ROS generated by ionizing radiation, UV, and desiccation [3, 15, 24]. We measured the generation of ROS by polarization in real time. This was done using two novel purpose-designed instruments that are described in this paper.
Materials and methods
DBA instrument
The double biofilm analyzer (DBA) has a platform mounted with 12 detachable stainless steel vials (AISI 316L, depth 30 mm, diameter 45 mm, holding volume 40 cm3) of which six were individually polarizable. The electric current into each vial was fed via a control unit and Pt-coated Nb-wire electrodes, one for each vial (Savcor Group Ltd, Mikkeli, Finland). The wire electrode and a reference electrode (Savcor model 1) were implanted in the platform lid and became immersed inside each of the six vials when the lid was closed. Each vial itself worked as the counter electrode. The vials were cleaned before each experiment by soaking in an ultrasonic bath of 1.0% (w/v) of an enzyme-containing laundry detergent (Bioluvil, Lever Fabergé, Finland B01/247636), 60 min, 60°C, rinsed with drinking water. For passivation the cups were soaked for 1–2 h in 0.1% NaOH at 50°C and rinsed with drinking water, and then immersed in the passivation liquid (AvestaPolarit 302, AvestaPolarit, Finland) for 20 min, rinsed with drinking water, and autoclaved. The polarization programs used in the DBA instrument were commercial tradeware delivered by Savcor Group Ltd (Mikkeli, Finland).
To screen for the antifouling effect of electrochemical polarization, biofilms of Deinococcus geothermalis strain E50051 (HAMBI 2411) were grown in each of the DBA vials filled with 8.5 ml of the synthetic paper machine water (SPW) amended with 10 vol% of modified R2 broth (Table 1) and 100 ppm of a silicon-based antifoam agent (Kemira Aerotech 1510, Kemira Chemicals, Finland) for 24 h, 87 rpm at 45°C (flow speed 0.3 m s−1). The vials were then emptied, rinsed, and refilled with 20.5 ml per vial of the same medium, so that the previously grown biofilm became submerged. At this stage biocide was added (or not) and the vials were polarized (or not) at 45°C, and rotated at 87 rpm for the indicated times. The applied polarization potentials were below the limits for corrosion of the steel. The vials were emptied, rinsed with sterile drinking water, and the amounts of biofilms were measured from each vial by the crystal violet staining method as described elsewhere [10]. In brief, biomass in the vials was stained with crystal violet (4 g l−1 in 20 vol% methanol) for 3 min, rinsed with drinking water, and allowed to air-dry. The stain retained by the biofilm was dissolved into 10 ml 70 vol% ethanol (30 min, 150 rpm, 35°C) and A 595 nm was measured using an iEMS photometric plate reader (Thermo Fisher, Vantaa Finland).
Compositions of synthetic (SPW with or without 10% R2) and of authentic paper machine white waters from two mills (A, B)
Analytes . | SPW + 10% R2 . | SPW . | White water of two paper machines . | |
---|---|---|---|---|
A . | B . | |||
pH, adjusted with NaOH or HCl | 7.0 or 3.0 | 7.0 or 3.0 | 8.5 | 7.6 |
Conductivity (μS cm−1) | 700 | 700 | 377 | 2,290 |
Al (mg l−1) | 16 | 16 | ND | ND |
Cl (mg l−1) | 65 | 65 | 30 | 23 |
Ca (mg l−1) | 36 | 36 | 360 | 480 |
K (mg l−1) | 16 | 3.4 | ND | ND |
Mg (mg l−1) | 0.9 | 0 | 7 | 8.6 |
Fe (mg l−1) | 1 | 1 | ND | ND |
Mn (mg l−1) | 0.9 | 0.9 | ND | ND |
Na (mg l−1) | 53 | 53 | 58 | 470 |
SO4 (mg l−1) | 161 | 160 | 17 | 630 |
PO4 (mg l−1) | 14 | 4.2 | ND | ND |
NH4 (mg l−1) | 2.5 | 2.5 | ND | ND |
Sum of inorganic | 366 | 342 | 472 | 1,612 |
Pyruvate | 110 | 80 | 30 | 30 |
Starch (potato) | 400 | 300 | ||
Yeast extract | 200 | 150 | ||
Peptone and amino acids | 100 | |||
Total solids | 3,110 | 4,510 | ||
Ash residue (550°C) | 2,050 | 720 | ||
Volatile solids | 298a | 288a | 1,060 | 3,790 |
Acetone extract (gravimetric) | 4 | 322 | ||
Total organic matter | 810 | 530b | 955a | 2,667a |
Analytes . | SPW + 10% R2 . | SPW . | White water of two paper machines . | |
---|---|---|---|---|
A . | B . | |||
pH, adjusted with NaOH or HCl | 7.0 or 3.0 | 7.0 or 3.0 | 8.5 | 7.6 |
Conductivity (μS cm−1) | 700 | 700 | 377 | 2,290 |
Al (mg l−1) | 16 | 16 | ND | ND |
Cl (mg l−1) | 65 | 65 | 30 | 23 |
Ca (mg l−1) | 36 | 36 | 360 | 480 |
K (mg l−1) | 16 | 3.4 | ND | ND |
Mg (mg l−1) | 0.9 | 0 | 7 | 8.6 |
Fe (mg l−1) | 1 | 1 | ND | ND |
Mn (mg l−1) | 0.9 | 0.9 | ND | ND |
Na (mg l−1) | 53 | 53 | 58 | 470 |
SO4 (mg l−1) | 161 | 160 | 17 | 630 |
PO4 (mg l−1) | 14 | 4.2 | ND | ND |
NH4 (mg l−1) | 2.5 | 2.5 | ND | ND |
Sum of inorganic | 366 | 342 | 472 | 1,612 |
Pyruvate | 110 | 80 | 30 | 30 |
Starch (potato) | 400 | 300 | ||
Yeast extract | 200 | 150 | ||
Peptone and amino acids | 100 | |||
Total solids | 3,110 | 4,510 | ||
Ash residue (550°C) | 2,050 | 720 | ||
Volatile solids | 298a | 288a | 1,060 | 3,790 |
Acetone extract (gravimetric) | 4 | 322 | ||
Total organic matter | 810 | 530b | 955a | 2,667a |
ND not determined
aCalculated from the composition
bExcluding the low-melting agarose used to reduce liquid flow in the RadBox cuvette
Compositions of synthetic (SPW with or without 10% R2) and of authentic paper machine white waters from two mills (A, B)
Analytes . | SPW + 10% R2 . | SPW . | White water of two paper machines . | |
---|---|---|---|---|
A . | B . | |||
pH, adjusted with NaOH or HCl | 7.0 or 3.0 | 7.0 or 3.0 | 8.5 | 7.6 |
Conductivity (μS cm−1) | 700 | 700 | 377 | 2,290 |
Al (mg l−1) | 16 | 16 | ND | ND |
Cl (mg l−1) | 65 | 65 | 30 | 23 |
Ca (mg l−1) | 36 | 36 | 360 | 480 |
K (mg l−1) | 16 | 3.4 | ND | ND |
Mg (mg l−1) | 0.9 | 0 | 7 | 8.6 |
Fe (mg l−1) | 1 | 1 | ND | ND |
Mn (mg l−1) | 0.9 | 0.9 | ND | ND |
Na (mg l−1) | 53 | 53 | 58 | 470 |
SO4 (mg l−1) | 161 | 160 | 17 | 630 |
PO4 (mg l−1) | 14 | 4.2 | ND | ND |
NH4 (mg l−1) | 2.5 | 2.5 | ND | ND |
Sum of inorganic | 366 | 342 | 472 | 1,612 |
Pyruvate | 110 | 80 | 30 | 30 |
Starch (potato) | 400 | 300 | ||
Yeast extract | 200 | 150 | ||
Peptone and amino acids | 100 | |||
Total solids | 3,110 | 4,510 | ||
Ash residue (550°C) | 2,050 | 720 | ||
Volatile solids | 298a | 288a | 1,060 | 3,790 |
Acetone extract (gravimetric) | 4 | 322 | ||
Total organic matter | 810 | 530b | 955a | 2,667a |
Analytes . | SPW + 10% R2 . | SPW . | White water of two paper machines . | |
---|---|---|---|---|
A . | B . | |||
pH, adjusted with NaOH or HCl | 7.0 or 3.0 | 7.0 or 3.0 | 8.5 | 7.6 |
Conductivity (μS cm−1) | 700 | 700 | 377 | 2,290 |
Al (mg l−1) | 16 | 16 | ND | ND |
Cl (mg l−1) | 65 | 65 | 30 | 23 |
Ca (mg l−1) | 36 | 36 | 360 | 480 |
K (mg l−1) | 16 | 3.4 | ND | ND |
Mg (mg l−1) | 0.9 | 0 | 7 | 8.6 |
Fe (mg l−1) | 1 | 1 | ND | ND |
Mn (mg l−1) | 0.9 | 0.9 | ND | ND |
Na (mg l−1) | 53 | 53 | 58 | 470 |
SO4 (mg l−1) | 161 | 160 | 17 | 630 |
PO4 (mg l−1) | 14 | 4.2 | ND | ND |
NH4 (mg l−1) | 2.5 | 2.5 | ND | ND |
Sum of inorganic | 366 | 342 | 472 | 1,612 |
Pyruvate | 110 | 80 | 30 | 30 |
Starch (potato) | 400 | 300 | ||
Yeast extract | 200 | 150 | ||
Peptone and amino acids | 100 | |||
Total solids | 3,110 | 4,510 | ||
Ash residue (550°C) | 2,050 | 720 | ||
Volatile solids | 298a | 288a | 1,060 | 3,790 |
Acetone extract (gravimetric) | 4 | 322 | ||
Total organic matter | 810 | 530b | 955a | 2,667a |
ND not determined
aCalculated from the composition
bExcluding the low-melting agarose used to reduce liquid flow in the RadBox cuvette
The RadBox instrument for real-time ROS detection
The radical detection cuvette, RadBox (10 mm × 25 mm, holding volume 4 ml; made of stainless steel, was equipped with a working electrode (∅ 2 mm steel wire of AISI 316L), longitudinally inserted into the cuvette. The two side walls (23 mm × 18 mm, AISI 316L) of the cuvette functioned as the counter electrodes. The potential of the working electrode was measured against a reference electrode (Savcor model 2). Before each experiment the wire electrode and the cuvette were cleaned similarly to the DBA vials (see above) and the working electrode was passivated for 20 min. The cuvette content (SPW, Table 1) was fortified with low-melting agarose (0.25% w/v, Sigma–Aldrich, St. Louis, USA) to attenuate the liquid flow. ROS was kinetically recorded as the fluorescence output, ex 355 and em 425 nm, by Tempo-9-Ac (50 μM) (Molecular Probes, Eugene, Oregon USA), continuously recorded for 300 s from 72 locations inside the cuvette (one round of 72 locations each 7.5 s) with a microplate reader (Fluoroskan Ascent, Thermo Fisher, Finland). The readings obtained were integrated for six blocks of longitudinal sections (12 locations each). Fluorescence acquisition was calibrated with hydrogen peroxide (Riedel-de Haen, Germany) with 1 mM FeSO4 Fenton’s reagent dissolved in synthetic paper machine water (SPW, acidified to pH 3.0). The fluorescence response to ROS generated by the Fenton reaction in SPW was linear when measured with 5–40 μM of Tempo-9-Ac. Fluorescence of 23 RFU (relative fluorescence unit) was obtained with 40 μM of Tempo-9-Ac. The calibration experiment as carried out using a microtiter plate.
Media and reagents
The composition of SPW (Table 1) aims to simulate authentic paper mill white waters. It contained (mg l−1): Na2SO4 100, K2HPO4·3H2O 13.4, FeSO4·7H2O 5.1, Al2(SO4)3·18H2O 194, CaCl2·2H2O 133, MnCl2·4H2O 3.1, yeast extract 150, soluble starch 300, Na-pyruvate 100, and (NH4)2SO4 10. pH was adjusted to 7.0 with 1 M NaOH or to 3.0 with 1 M HCl. Where glucose was replaced by soluble potato starch, 10 vol% of R2 broth [5] was added to SPW in DBA experiments to allow biofilm growth of D. geothermalis. Tempo-9-Ac (4-((9-acridinecarbonyl) amino)-2,2,6,6-tetramethylpiperidin-1-oxyl) from Molecular Probes (Invitrogen Eugene, Oregon USA) 20 mM was dissolved in dimethyl sulfoxide (J.T.Baker, Deventer, Holland).
The biocides were technical products (Kemira Chemicals, Vaasa, Finland) and used as received (concentration of the effective substance): 2-dibromo-2-cyanoacetamide (20%) (Fennosan R20 V); bromochloro-5,5-dimethylhydantoin (99% w/w) (Fennosan Br99); peracetic acid (15% with 13–16% H2O2 w/w) (Fennosan PAA), and methylene bisthiocyanate (9%) (Fennosan M9).
Statistical analysis
Statistical significance was estimated by the contrast factorial analysis using the SAS Program (version 6.12, SAS Institute, Cary, USA) and by the Student t test analysis of variance (ANOVA).
Results
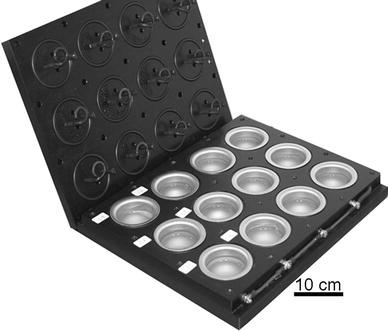
Double biofilm analyzer (DBA) instrument with the lid open. The instrument consists of a platform with holders for 12 stainless steel vials of which six were individually polarizable. Each vial was provided with a wire electrode and a lid-mounted reference electrode so that they reached the vial when closing the lid. The vial operated as the counter electrode. The DBA instrument was operated in a rotating thermostatic incubator (45°C) that provided a liquid flow of 0.3 m s−1 in each vial
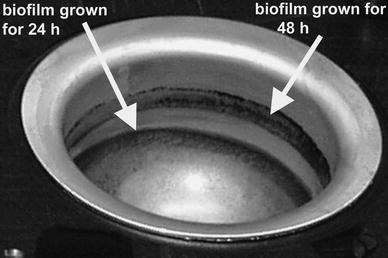
Biofilms of Deinococcus geothermalis accumulated on air–liquid interfaces of the stainless steel vials in the DBA instrument. The lowest biofilm in the vials resulted from growth in 8.5 ml of SPW medium seeded with D. geothermalis. The vial was then emptied, rinsed, and filled with 20.5 ml of fresh SPW. The previous biofilm thus became submerged and a new biofilm developed above it at the air–liquid interface
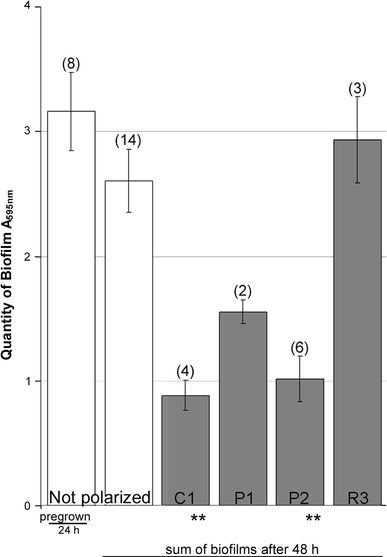
Effect of polarization on biofilms of D. geothermalis on stainless steel. Biofilms were grown in SPW in stainless steel vials for 24 h, then emptied, rinsed, and refilled as described in Fig. 2 and subsequently polarized for 48 h. The y-axis indicates the total amount of biofilm resulting from the 24 h + 48 h of growth on nonpolarized (white columns) and polarized surfaces (shaded columns) of the steel vials. The used polarization programs were cathodic (C1), cathodic weighted pulsed (P1 and P2), and pulsed at random anodic and cathodic (R3). The error bars were calculated from independent experiments. Significant difference is indicated P < 0.05 **
Polarization enhances D. geothermalis biofilm removal by biocides
. | Polarization program biofilm remaining, % of nontreated . | |||||
---|---|---|---|---|---|---|
None . | P2 . | C1 . | ||||
Oxidative biocide (ppm)a . | Mean . | SD . | Mean . | SD . | Mean . | SD . |
0 | 100 | 9.6 | 39 | 6.4 | 33.9 | 8.5 |
5 | 80.1 | 21.2 | 47.3 | 17.4 | ||
25 | 53.5 | 15.5 | 27.4 | 10 |
. | Polarization program biofilm remaining, % of nontreated . | |||||
---|---|---|---|---|---|---|
None . | P2 . | C1 . | ||||
Oxidative biocide (ppm)a . | Mean . | SD . | Mean . | SD . | Mean . | SD . |
0 | 100 | 9.6 | 39 | 6.4 | 33.9 | 8.5 |
5 | 80.1 | 21.2 | 47.3 | 17.4 | ||
25 | 53.5 | 15.5 | 27.4 | 10 |
Polarization enhances D. geothermalis biofilm removal by biocides
. | Polarization program biofilm remaining, % of nontreated . | |||||
---|---|---|---|---|---|---|
None . | P2 . | C1 . | ||||
Oxidative biocide (ppm)a . | Mean . | SD . | Mean . | SD . | Mean . | SD . |
0 | 100 | 9.6 | 39 | 6.4 | 33.9 | 8.5 |
5 | 80.1 | 21.2 | 47.3 | 17.4 | ||
25 | 53.5 | 15.5 | 27.4 | 10 |
. | Polarization program biofilm remaining, % of nontreated . | |||||
---|---|---|---|---|---|---|
None . | P2 . | C1 . | ||||
Oxidative biocide (ppm)a . | Mean . | SD . | Mean . | SD . | Mean . | SD . |
0 | 100 | 9.6 | 39 | 6.4 | 33.9 | 8.5 |
5 | 80.1 | 21.2 | 47.3 | 17.4 | ||
25 | 53.5 | 15.5 | 27.4 | 10 |
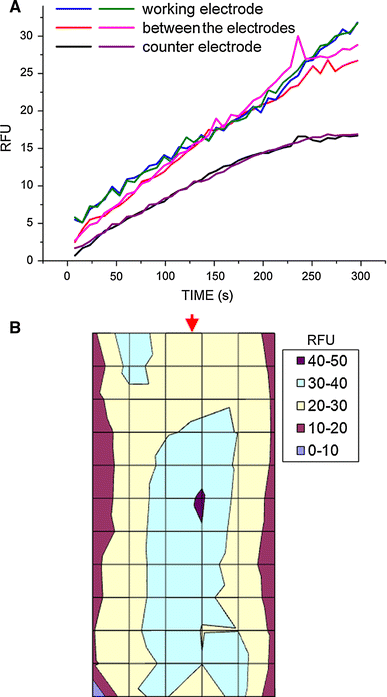
a The kinetics of fluorescence emission from the RadBox cuvette by Tempo-9-ac (50 μM) during pulsed polarization (P2) in SPW medium. The fluorescence (RFU) emitted by Tempo-9-Ac (50 μM) excited by the ROS generated during the polarization was recorded from 72 locations of the RadBox cuvette (7.5 s per each round, 300 s in total). The readings were integrated for blocks of six longitudinal sectors (12 locations in each). The lines show the averaged RFU from these sectors shown in b. b Map of the cumulative (300 s) fluorescence output from the different sectors in the cuvette. The arrowhead points to the location of the working electrode in the cuvette. The left and the right sides of b represent the walls of the cuvette that functioned as the counter electrodes
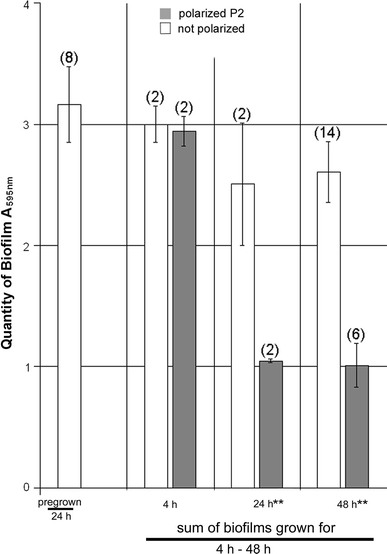
Biofilm removal from stainless steel vials in the DBA instrument during pulsed cathodically weighted polarization (P2). The biofilms were grown from inocula of D. geothermalis in 8.5 ml medium for 24 h as described for Fig. 2. The vials were then emptied, rinsed, and refilled with 20.5 ml medium and polarized (or not) for 4 h, 24 h, or 48 h. The columns show the summed amount of biofilms per vial and the error bars (n is given in parenthesis). Significant difference is indicated P < 0.05 **
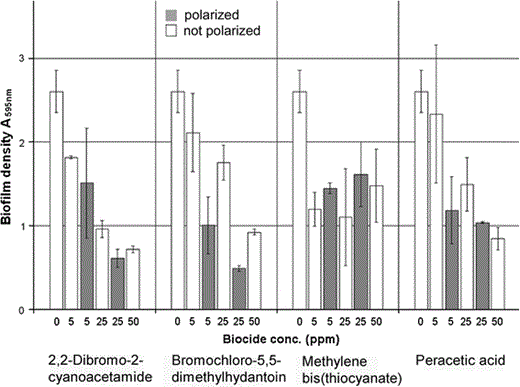
Combining industrial biocides with polarization. Biofilms were grown (8.5 ml of the SPW medium) in vials of stainless steel in the DBA instrument as described for Fig. 2. The vials were emptied and refilled (20.5 ml) with SPW containing (0 ppm, 5 ppm, 25 ppm, or 50 ppm) of the indicated biocide (technical product). Biocides with concentrations of 5–25 ppm were combined with polarization. The technical products contained the effective substance 2-dibromo-2-cyanoacetamide (20%), bromochloro-5,5-dimethylhydantoin (99% w/w), or peracetic acid (15% peracetic acid with 13–16% H2O2 w/w). The columns indicate the total amount of biofilm found per vial 48 h later (45°C, 87 rpm). The columns show averages of two independent experiments with the error bars
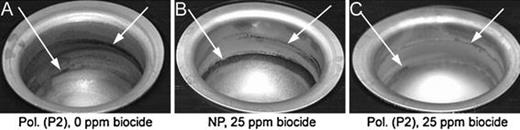
Examples of DBA vials with biofilms remaining after polarization treatments with or without biocide. The biofilms were grown for 24 h in biocide-free medium (8.5 ml of SPW) followed by 48 h of growth (20.5 ml of SPW) with 0 ppm (a) or 25 ppm (b, c) of 2-dibromo-2-cyanoacetamide containing product. The vials in a and c were polarized with a cathodically weighted pulsed program (P2); b shows a vial that was not polarized. Biofilms were grown (24 h, left arrow) before and (48 h, right arrow) after the polarization. Submerged biofilm (left arrow) is absent in a and c and the splash area biofilm is absent in b and c. Similar results were seen with the other oxidative biocides
Discussion
This study is the first to show the antifouling potency of electrochemical polarization towards biofilms of an industrial biofouler microorganism resistant towards oxidative biocides. We also show that ROS were generated when the stainless steel surfaces were polarized in nonsaline waters with high organic content resembling those in paper machines (Table 1). We reduced the calcium content in SPW compared to authentic paper mill waters to enable optical transparency for the fluorescence measurements.
D. geothermalis is a suitable test organism for studying biofilm removal because it is exceptionally resistant towards ROS generated by irradiation [3] and one of the most biocide-resistant biofilm formers found in the paper industry [11, 17]. Suboptimal biocide dosing even stimulated most D. geothermalis strains to form biofilm [10]. The firm attachment of D. geothermalis is mediated by extracellular polymeric substance (EPS) glycoconjugates [18] and adhesion threads consisting of glycoproteins of type IV pili [22]. The deinococcal biofilm possesses a tightly sealed colony matrix consisting of several different glycoconjugates [18] and acts as a pedestal for less effective biofilm formers such as Bacillus species to adhere on nonliving surfaces [11, 17].
We showed here that cathodically weighted pulsed polarization removed the submerged biofilms of D. geothermalis from stainless steel where the biocides were ineffective. The antifouling effect of cathodic polarization has been postulated to depend on the electro-repulsive interactions between the negatively charged bacteria and the substratum [2, 6, 21, 25–27]. Hong et al. [6] showed that an anodic current inactivated 85% of Pseudomonas aeruginosa cells persisting on a metal oxide surface whereas a cathodic current did not kill them. However, a direct anodic current is not usable as an antifoulant because it clusters the negatively charged bacteria to the surface and thus hinders electric conductivity reactions on the electrode surface [6, 20].
Electrochemical ROS generation has been shown to occur both anodically and cathodically [7–9, 29]. A pulsed current may be superior to a direct current for antifouling because it avoids accumulation of ions that could result into insoluble deposits with low electric conductivity of the surface thus decreasing the impact of the electric power [27]. We showed that the same cathodically weighted pulsed polarization program that had an antifouling effect also produced ROS, indicating a role of ROS in the antifouling. The pulsating pattern of ROS generation, detected during the cathodically weighted pulsed polarization, indicates that ROS were formed during the pulse only, cathodic or anodic. At the cathode oxygen reduction can indirectly produce superoxide anion and hydroxyl radicals [23, 29]. Recently Pérez-Roa et al. [19] showed that during pulsed electric polarization oxygen was reduced to hydrogen peroxide at a cathode immersed in seawater. Using a fluorogenic dye, BODIPY, they also detected hydroxyl radicals at the cathode [19]. In this study an ample amount of ROS was detected, using the fluorogenic dye Tempo-9-Ac, in the SPW medium containing a high amount (>500 mg l−1) of organic substance. On the basis of the data obtained with the radical-measuring cuvette (RadBox) we suggest that the ROS connected to antifouling was produced at the cathodic electrode.
The air–liquid interface is often a source of biofilms, and D. geothermalis favors such areas. The ineffectivity of polarization against biofilms on air–liquid interfaces is obviously due to lack of electric conductivity. In systems where water levels periodically change, such as in paper machines, the biofilms can be removed by polarization during the periods when the water level will be raised. Alternatively, oxidative biocides can be combined with pulsed cathodic polarization to keep the air–liquid interfaces clean.
To our knowledge the present study is the first evidence for the role of ROS in removal of actively growing, macroscopically visible (Figs 2 and 7) biofilm from steel surfaces. It has earlier been shown [1] that Pseudomonas aeruginosa on stainless steel surface was killed by low biocide concentrations combined with electric polarization. That study was done with nonoxidizing biocides (Kathon, quaternary, and glutaraldehyde) in a flow cell (modified Robbins device) fed with buffer solution. We showed that removal of D. geothermalis biofilm was enhanced by cathodically weighted pulsed polarization in the presence of low concentrations of oxidizing biocides (bromochloro-5,5-dimethylhydantoin, 2,2-dibromo-2-cyanoacetamide, or peracetic acid). In industrial applications it is important to remove biofilm (dead or alive) and deposits from process surfaces, whereas medical applications aim to kill infectious organisms. It has been suggested that block current or pulsed polarization could inactivate cells by disrupting the integrity of the cellular membranes by rapid reversals of the anodic and cathodic currents [14, 26] but removal of mature biofilms has not been reported.
The fact that the tenacious biofilm former Deinococcus geothermalis was effectively controlled, under conditions simulating paper machines, by combining pulsed polarization with an oxidative biocide, suggests positive prospects for the control of other biofoulers as well. As a spin-off effect, the secondary biofilm-formers whose adherence is promoted by a surface colonized by D. geothermalis could also be controlled.
Acknowledgments
This work was supported by the technology program PINTA of the Finnish Funding Agency for Technology and Innovation (Tekes). Additional support was obtained from Kemira Oyj, Savcor Forest Oyj, StoraEnso Oyj, UPM-Kymmene Oyj and the Center of Excellence (Photobiomics, grant no. 118637) of the Academy of Finland. We thank the Helsinki University Viikki Science Library for excellent information service, the Faculty of Agriculture and Forestry Instrument Centre for technical support, and Leena Steininger, Hannele Tukiainen, and Tuula Suortti for many kinds of help.
References
Peltola M, Neu TR, Raulio M, Kolari M, Salkinoja-Salonen MS (2008) Architecture of Deinococcus geothermalis biofilms on glass and steel: A lectin study. Environ Microbiol. doi:10.1111/j.1462-2920.2008.01596.x