-
PDF
- Split View
-
Views
-
Cite
Cite
Manabu Igarashi, Takatsugu Hirokawa, Ayato Takada, Structural and Energetic Basis for Differential Binding of Ebola and Marburg Virus Glycoproteins to a Bat-Derived Niemann-Pick C1 Protein, The Journal of Infectious Diseases, Volume 228, Issue Supplement_7, 15 November 2023, Pages S479–S487, https://doi.org/10.1093/infdis/jiad120
- Share Icon Share
Abstract
Our previous study demonstrated that the fruit bat (Yaeyama flying fox)-derived cell line FBKT1 showed preferential susceptibility to Ebola virus (EBOV), whereas the human cell line HEK293T was similarly susceptible to EBOV and Marburg virus (MARV). This was due to 3 amino acid differences of the endosomal receptor Niemann-Pick C1 (NPC1) between FBKT1 and HEK293T (ie, TET and SGA, respectively, at positions 425–427), as well as 2 amino acid differences at positions 87 and 142 of the viral glycoprotein (GP) between EBOV and MARV.
To understand the contribution of these amino acid differences to interactions between NPC1 and GP, we performed molecular dynamics simulations and binding free energy calculations. The average binding free energies of human NPC1 (hNPC1) and its mutant having TET at positions 425–427 (hNPC1/TET) were similar for the interaction with EBOV GP. In contrast, hNPC1/TET had a weaker interaction with MARV GP than wild-type hNPC1. As expected, substitutions of amino acid residues at 87 or 142 in EBOV and MARV GPs converted the binding affinity to hNPC1/TET.
Our data provide structural and energetic insights for understanding potential differences in the GP-NPC1 interaction, which could influence the host tropism of EBOV and MARV.
Most filoviruses in the genera Marburgvirus and Ebolavirus in the family Filoviridae are zoonotic pathogens that cause severe diseases in humans and nonhuman primates. The genus Marburgvirus includes a single species with 2 viruses: Marburg virus (MARV) and Ravn virus (RAVV), whereas the genus Ebolavirus includes 6 distinct species, namely, Ebola virus (EBOV), Sudan virus (SUDV), Taï forest virus (TAFV), Bundibugyo virus (BDBV), Reston virus (RESTV), and Bombali virus (BOMV) [1]. The 2 marburgviruses (MARV and RAVV) and 4 of the ebolaviruses (EBOV, SUDV, TAFV, and BDBV) are known to be human-pathogenic filoviruses [2]. Although filovirus disease outbreaks have been reported only in African countries, ecological and epidemiological studies suggest the occurrence of filovirus infection in humans and animals in nonendemic areas in Africa, and even in Asian and European countries [3–14].
Bats are considered to be natural reservoirs for some filoviruses. Many epidemiological studies have suggested that filoviruses infect various bat species, including frugivorous and insectivorous bats, both of which are widely distributed in African, European, and Asian countries [15]. It is interesting to note that an experimental study showed that the Egyptian fruit bat (Rousettus aegyptiacus), which is a natural reservoir for marburgviruses, was efficiently infected with MARV but not EBOV, SUDV, TAFV, BDBV, or RESTV, suggesting a differential host tropism between marburgvirus and ebolavirus [16]. Our previous study also demonstrated a difference between EBOV and MARV infectivities in a bat-derived cell line from the Yaeyama flying fox (Pteropus dasymallus yayeyamae), FBKT1, which showed preferential susceptibility to EBOV [17]. We found that this was due to the unique amino acid sequence of the endosomal filovirus receptor Niemann-Pick C1 (NPC1) of FBKT1 as well as a few specific amino acid differences of the viral surface glycoprotein (GP) between EBOV and MARV [17].
Filovirus GP is responsible for viral entry into cells and the single envelope glycoprotein required for attachment and membrane fusion. The GP molecule exists as a homotrimer; each monomer consists of disulfide-linked subunits, GP1 and GP2. GP1 contains the receptor binding site (RBS), glycan cap, and mucin-like domain, whereas GP2 contains the fusion loop and transmembrane domain [18]. After attachment of GP to cell surface receptors (eg, C-type lectins), ebolavirus and maruburgvirus enter cells through macropinocytosis [19–21]. In the late endosome, GP is cleaved at multiple sites by host proteases (eg, cathepsins L and B), followed by the removal of the glycan cap and mucin-like domain [22]. The cleaved GP (GPcl) containing the exposed RBS binds to the endosomal receptor NPC1, leading to membrane fusion [23, 24]. The crystal structure of EBOV GPcl in complex with human NPC1 domain C (NPC1-C) revealed that the interaction between EBOV GPcl and NPC1-C was mediated by 2 protruding loops of NPC1-C (loop 1 and loop 2), which bind to the hydrophobic pocket in RBS on the head of GPcl (Figure 1A) [25].
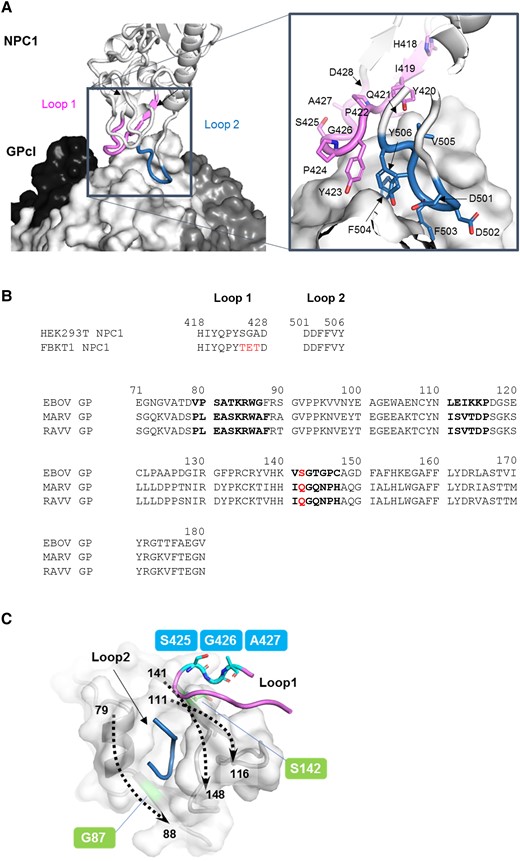
Interaction between glycoprotein (GP) and Niemann-Pick C1 (NPC1). (A) The 3-dimensional structures of the Ebola virus (EBOV) cleaved GP (GPcl) trimer and human NPC1 (hNPC1)-C (Protein Data Bank ID: 5F1B) are represented as a surface and a ribbon model, respectively. On the GPcl trimer, 1 monomer (center) is colored white and the others are colored black and dark gray. The GPcl binding interface including hNPC1 loop 1 and loop 2 (indicated in violet and sky blue, respectively) is shown in the boxed areas. Nitrogen and oxygen atoms are shown in blue and red, respectively. The amino acid residues of loop 1 and loop 2 in hNPC1 are displayed. (B) Amino acid sequences of NPC1 loops and GPs are shown. Different amino acids between hNPC1 and FBKT1 NPC1 are shown in red. Sequences of receptor binding site of EBOV, Marburg virus (MARV), and Ravn virus (RAVV) GPs are aligned using EBOV numbering. Amino acid residues that mainly interact with the NPC1 molecule are shown in bold face. Amino acids at position 142 are shown in red. (C) The edge of the NPC-binding pocket is formed by 3 regions (ie, amino acid positions 79–88, 111–116, and 141–148). The 3-dimensional structures of GP and hNPC1 in the EBOV GPcl-NPC1-C complex are represented as surface and cartoon models, respectively. Dashed arrows show the directions of amino acid residues, where the numbers indicate the amino acid positions of the cleft of the receptor binding pocket. Amino acid residues at positions 425–427 in NPC1 loop 1 are located close to the residue at position 142 of GP but away from the residue at position 87.
The loop 1 region of FBKT1 NPC1-C has the unique amino acid residues at positions 425–427, compared with a human cell line (HEK293T) and the other bat cell lines tested (Figure 1B) [17]. Unlike HEK293T and the other bat cell lines that showed similar susceptibilities to both EBOV and MARV, FBKT1 showed preference for EBOV, and the unique amino acid residues were responsible for the decreased susceptibility to MARV [17]. The cocrystal structure of human NPC1-C and EBOV GPcl showed that the amino acid residues at positions 425–427 in loop 1 could interact with S at position 142 of GP (Figure 1C), and, indeed, amino acid swapping at position 142 between EBOV and MARV GPs resulted in increased and decreased infectivities of vesicular stomatitis Indiana virus (VSIV) pseudotyped with MARV (VSIV-MARV) and EBOV (VSIV-EBOV) GPs, respectively, in FBKT1 cells [17]. These findings motivated us to investigate how these amino acid substitutions in NPC1 and GP affected the intermolecular interaction.
In this study, we computationally examined the effects of above-mentioned amino acid substitutions on the GP-NPC1 interaction by molecular modeling and molecular dynamics (MD) simulations, followed by binding free energy analysis.
METHODS
Simulation Setup
The structure of the complex formed by EBOV GPcl and NPC1-C was downloaded from the Protein Data Bank (PDB) (PDB code: 5F1B). The trimer structure of RAVV GP in complex with a neutralizing antibody was also downloaded from the PDB (PDB code: 5UQY). Based on the visible region of the EBOV GPcl-NPC1 complex (PDB code: 5F1B), the structural model of the free GPcl trimer for RAVV, in which constituent residues of the NPC1-binding pocket are 100% identical to MARV, was then obtained from the GP structure by deleting the coordinates of the antibody and glycan cap domains. After a single EBOV GPcl-NPC1 monomer in 5F1B was superimposed on a single monomer of RAVV GPcl trimer, the EBOV GPcl trimer and 2 of the 3 NPC1 were removed because the GPcl trimer may simply bind to 1 NPC1 [26]. Structures of the NPC1 and GP mutants were generated by replacing the corresponding amino acids, using Molecular Operating Environment (MOE) software (version 2018; Chemical Computing Group, Montreal, Canada).
Molecular Dynamics Simulations
The MD simulations were performed for the complexes of EBOV GPcl-NPC1-C and RAVV GPcl-NPC1-C and their mutants. The AMBER ff14SB force field was used for all the simulations [27]. The protonation states of the ionizable residues were assigned at pH 7.0 using the PDB2PQR web server [28]. Missing hydrogen atoms were added to the LEaP module in AMBER 16 [29]. The total charges were neutralized by the addition of either sodium or chloride counterions. The systems were then solvated in a truncated octahedral box of TIP3P water molecules with a distance of at least 10 Å around the proteins. Energy minimizations and MD simulations were performed using the pmemd.cuda program in AMBER 16, with a cutoff radius of 10 Å for the nonbonded interactions. All systems were energy-minimized in 4 steps: first, only hydrogen atoms; second, water and ions; third, the side chains; and fourth, all atoms. For energy minimization, the steepest descent method was used for 500 steps followed by the conjugate gradient method for 1500 steps. After energy minimization, the system was gradually heated from 0 to 310 K over 300 picoseconds (ps) with harmonic restraints (with a force constant of 1.0 kcal/mol·Å2). Two additional rounds of MD simulations (50 ps each at 310 K) were performed with decreasing restraint weight from 0.5 to 0.1 kcal/mol·Å2. Next, an unrestrained production run of 150 nanoseconds (ns) was performed, and the production trajectories were collected every 10 ps. All MD simulations were performed using the NPT ensemble and the Berendsen algorithm to control the temperature and pressure [30]. The time step was 2 femtoseconds (fs), and the SHAKE algorithm was used to constrain all bond lengths involving hydrogen atoms [31]. Long-range electrostatic interactions were treated using the particle mesh Ewald method [32]. For each GPcl-NPC1-C complex system, MD simulations were performed 3 times using different initial velocity distributions. Analysis of the trajectories was performed using the CPPTRAJ module of AmberTools16.
The stability of the trajectories was assessed by monitoring the root mean square deviations (RMSDs) of the backbone Cα atoms from the initial structure of MD simulations. After confirming that RMSDs in all systems reached equilibrium within 100 ns, the trajectories extracted from the last 50 ns (ie, 100–150 ns) were used in subsequent analyses.
Binding Free Energy and Per-Residue Decomposition Analysis
Binding free energies and per-residue decomposition energy values were calculated using the script of the molecular mechanics/generalized Born surface area (MM/GBSA) method in AmberTools16 (MMPBSA.py), in which a single-trajectory approach was used. The conformational entropy was not considered due to the high computational cost and low prediction accuracy. The salt concentration was set to 0.20 M.
RESULTS
Effects of the TET Substitution in Human Niemann-Pick C1 on the Binding Free Energy to Interact With Glycoproteins
The NPC1 of FBKT1 has T, E, and T at positions 425, 426, and 427 in loop 1, respectively, whereas the corresponding residues of human NPC1 (hNPC1) are S, G, and A (Figure 1B). We previously demonstrated that cells expressing an hNPC1 mutant (hNPC1/TET) that had substituted amino acid residues TET at positions 425–427 instead of SGA were susceptible to VSIV-EBOV but much less susceptible to VSIV-MARV than those expressing wild-type hNPC1 [17]. We thus compared the binding potentials of the wild-type hNPC1 and its mutant (hNPC1/TET) to GPs (Table 1). Because there was no available structure of MARV GP in the PDB, we utilized the crystal structure of RAVV GP, which has amino acid residues identical to MARV GP in the NPC1-binding pocket (Figure 1B). The total average binding free energies (ΔG) of the 2 loops in hNPC1 and hNPC1/TET were similar (−30.4 and −32.9 kcal/mol, respectively) for EBOV GP. On the other hand, the ΔG value of hNPC1/TET for RAVV GP (−14.2 kcal/mol) increased +16.7 kcal/mol compared to that of hNPC1 (−30.9 kcal/mol), implying that hNPC1/TET had a weaker interaction with RAVV GP than wild-type hNPC1. In particular, the ΔG value of hNPC1 loop 1 was −13.3 kcal/mol, whereas that of hNPC1/TET loop 1 was only −0.7 kcal/mol. These results supported our experimental observations as mentioned above [17].
. | . | EBOV . | RAVV . |
---|---|---|---|
hNPC1 | Loop 1 | −11.2 ± 0.3 | −13.3 ± 1.3 |
Loop 2 | −19.2 ± 0.6 | −17.6 ± 0.0 | |
Total | −30.4 ± 0.4 | −30.9 ± 1.3 | |
hNPC1/TET | Loop 1 | −12.9 ± 1.5 | −0.7 ± 1.6 |
Loop 2 | −20.0 ± 0.3 | −13.5 ± 1.5 | |
Total | −32.9 ± 1.3 | −14.2 ± 2.0 |
. | . | EBOV . | RAVV . |
---|---|---|---|
hNPC1 | Loop 1 | −11.2 ± 0.3 | −13.3 ± 1.3 |
Loop 2 | −19.2 ± 0.6 | −17.6 ± 0.0 | |
Total | −30.4 ± 0.4 | −30.9 ± 1.3 | |
hNPC1/TET | Loop 1 | −12.9 ± 1.5 | −0.7 ± 1.6 |
Loop 2 | −20.0 ± 0.3 | −13.5 ± 1.5 | |
Total | −32.9 ± 1.3 | −14.2 ± 2.0 |
Abbreviations: EBOV, Ebola virus; GPcl, cleaved glycoprotein; hNPC1, human NPC1; NPC1, Niemann-Pick C1; RAVV, Ravn virus.
aAverage binding free energies (in kcal/mol) were calculated by adding the energies of each residue of the loops.
. | . | EBOV . | RAVV . |
---|---|---|---|
hNPC1 | Loop 1 | −11.2 ± 0.3 | −13.3 ± 1.3 |
Loop 2 | −19.2 ± 0.6 | −17.6 ± 0.0 | |
Total | −30.4 ± 0.4 | −30.9 ± 1.3 | |
hNPC1/TET | Loop 1 | −12.9 ± 1.5 | −0.7 ± 1.6 |
Loop 2 | −20.0 ± 0.3 | −13.5 ± 1.5 | |
Total | −32.9 ± 1.3 | −14.2 ± 2.0 |
. | . | EBOV . | RAVV . |
---|---|---|---|
hNPC1 | Loop 1 | −11.2 ± 0.3 | −13.3 ± 1.3 |
Loop 2 | −19.2 ± 0.6 | −17.6 ± 0.0 | |
Total | −30.4 ± 0.4 | −30.9 ± 1.3 | |
hNPC1/TET | Loop 1 | −12.9 ± 1.5 | −0.7 ± 1.6 |
Loop 2 | −20.0 ± 0.3 | −13.5 ± 1.5 | |
Total | −32.9 ± 1.3 | −14.2 ± 2.0 |
Abbreviations: EBOV, Ebola virus; GPcl, cleaved glycoprotein; hNPC1, human NPC1; NPC1, Niemann-Pick C1; RAVV, Ravn virus.
aAverage binding free energies (in kcal/mol) were calculated by adding the energies of each residue of the loops.
Per-Residue Energy Contributions of Niemann-Pick C1 Loops and Glycoprotein Receptor Binding Site to the Binding Potential
To clarify the contribution of each loop-constituent residue to the interactions with EBOV and RAVV GPs, we performed MM/GBSA free energy decomposition analysis for hNPC1 and hNPC1/TET (Figure 2A). The per-residue energy contribution patterns of the hNPC1 loops to EBOV GP binding were similar to those of the hNPC1/TET loops, except for 1 residue (Q421) that showed an energy difference of ≥1.0 kcal/mol between hNPC1 and hNPC1/TET (Figure 2A, left panel). In contrast, the TET substitution in hNPC1 destabilized the interaction with RAVV GP at all residues in loop 1 (Figure 1A, right panel). It is notable that E426 of the hNPC1/TET loop had an unfavorable energy contribution to RAVV GP binding compared with G426 of hNPC1.
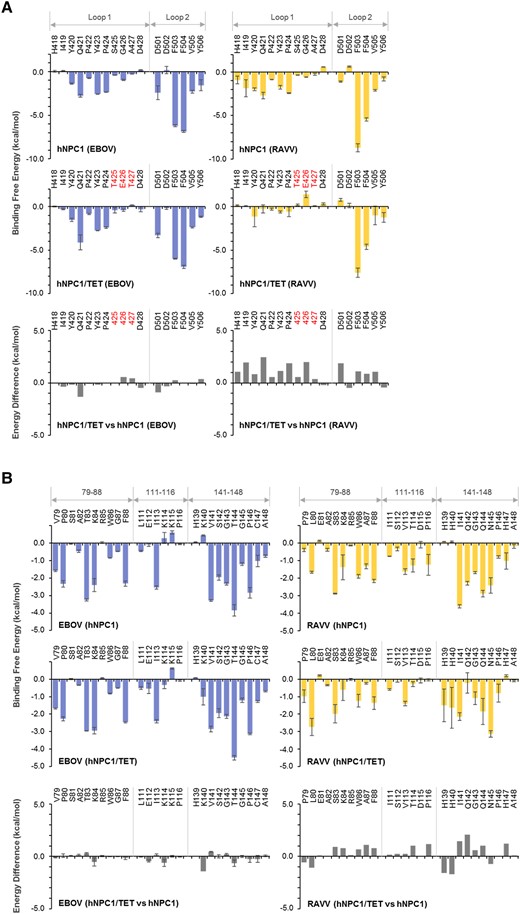
Per-residue decomposition analysis of human Niemann-Pick C1 (hNPC1) and hNPC1/TET for the interactions with Ebola virus (EBOV) and Ravn virus (RAVV) glycoproteins (GPs). (A) Per-residue contributions to the binding free energies of loop 1 and loop 2 in hNPC1 and hNPC1/TET for the interaction with EBOV or RAVV GPs were calculated using molecular mechanics/generalized Born surface area (MM/GBSA) free energy decomposition analysis. Each and molecular dynamics (MD) simulation was conducted 3 times, and averages and standard errors (SE) are shown. Amino acid residues that are distinct from those of hNPC1 (ie, TET at positions 426–428) are shown in red. Differences in binding free energies were calculated by subtraction of the values of hNPC1 from those of hNPC1/TET. (B) Per-residue contributions to the binding free energies of EBOV and RAVV GPs for the interactions with hNPC1 and hNPC1/TET were calculated using MM/GBSA free energy decomposition analysis. The numbering scheme for GP was adapted from EBOV. Each MD simulation was conducted 3 times, and averages and SE are shown. Differences of binding free energies were calculated by subtraction of the values of hNPC1 from those of hNPC1/TET.
The receptor binding pocket of GP mainly consists of 3 regions (ie, amino acid positions 79–88, 111–116, and 141–148) (Figure 1C). To examine the contribution of each amino acid residue in RBS to the interaction with hNPC1 and hNPC1/TET, we then conducted decomposition analysis for the GP molecules of EBOV and RAVV. Consistent with the results for NPC1 loops, little difference was found in the per-residue energy contributions of EBOV GP between the interactions with hNPC1 and hNPC1/TET (Figure 2B, left panel). In contrast, 10 amino acid positions (80, 87, 114, 116, 139, 140, 141, 142, 144, and 147) in RAVV GP displayed energy differences of ≥1.0 kcal/mol between hNPC and hNPC1/TET (Figure 2B, right panel). Of these, amino acid residues A87, T114, P116, I141, Q142, Q144, and H147 in RAVV GP had more unfavorable energy contributions to the interaction with hNPC1/TET than with hNPC1. Notably, the biggest difference in free-energy contribution between hNPC1 and hNPC1/TET occurred at Q142.
Effects of Amino Acid Substitutions in Glycoproteins on the Binding Free Energy to Interact With Human Niemann-Pick C1/TET
Next we examined the effects of amino acid substitutions at positions 87 and 142 of EBOV and RAVV GPs on the binding free energy to interact with hNPC1/TET (Table 2). The total average ΔG values of the hNPC1/TET loops were −22.0, −27.7, −30.2, and −28.0 kcal/mol for EBOV S142Q, RAVV Q142S, EBOV G87A, and RAVV A87G GP mutants, respectively. The ΔG value of EBOV S142Q (−22.0 kcal/mol) was 10.9 kcal/mol higher than that of wild-type EBOV (−32.9 kcal/mol), whereas the ΔG value of RAVV Q142S (−27.7 kcal/mol) was 13.5 kcal/mol lower than that of wild-type RAVV (−14.2 kcal/mol) (Tables 1 and 2). These results suggested that EBOV S142Q and RAVV Q142S substitutions led to decreased and increased binding affinities to hNPC1/TET, respectively. Although the ΔG value of EBOV G87A (−30.2 kcal/mol) was almost equal to that of wild-type EBOV (−32.9 kcal/mol), the value of RAVV A87G (−28.0 kcal/mol) was lower than that of wild-type RAVV (14.2 kcal/mol), suggesting that the RAVV A87G substitution resulted in an increased binding affinity to hNPC1/TET. It is interesting to note, however, that the amino acid at position 87 of GP was located on the opposite side of position 142 away from loop 1 in the NPC1-binding pocket (Figure 1C) [33].
Calculated Average Binding Free Energies Between NPC1 Loops and GPcl Mutantsa
. | . | EBOV S142Q . | RAVV Q142S . | EBOV G87A . | RAVV A87G . |
---|---|---|---|---|---|
hNPC1/TET | Loop 1 | −5.4 ± 2.1 | −11.7 ± 1.7 | −11.2 ± 0.2 | −12.4 ± 0.6 |
Loop 2 | −16.6 ± 1.8 | −16.0 ± 0.8 | −19.0 ± 0.5 | −15.6 ± 1.5 | |
Total | −22.0 ± 3.8 | −27.7 ± 1.8 | −30.2 ± 0.7 | −28.0 ± 2.2 |
. | . | EBOV S142Q . | RAVV Q142S . | EBOV G87A . | RAVV A87G . |
---|---|---|---|---|---|
hNPC1/TET | Loop 1 | −5.4 ± 2.1 | −11.7 ± 1.7 | −11.2 ± 0.2 | −12.4 ± 0.6 |
Loop 2 | −16.6 ± 1.8 | −16.0 ± 0.8 | −19.0 ± 0.5 | −15.6 ± 1.5 | |
Total | −22.0 ± 3.8 | −27.7 ± 1.8 | −30.2 ± 0.7 | −28.0 ± 2.2 |
Abbreviations: EBOV, Ebola virus; Gpcl, cleaved glycoprotein; hNPC1, human NPC1; NPC1, Niemann-Pick C1; RAVV, Ravn virus.
aAverage binding free energies (in kcal/mol) were calculated by adding the energies of each residue of the loops.
Calculated Average Binding Free Energies Between NPC1 Loops and GPcl Mutantsa
. | . | EBOV S142Q . | RAVV Q142S . | EBOV G87A . | RAVV A87G . |
---|---|---|---|---|---|
hNPC1/TET | Loop 1 | −5.4 ± 2.1 | −11.7 ± 1.7 | −11.2 ± 0.2 | −12.4 ± 0.6 |
Loop 2 | −16.6 ± 1.8 | −16.0 ± 0.8 | −19.0 ± 0.5 | −15.6 ± 1.5 | |
Total | −22.0 ± 3.8 | −27.7 ± 1.8 | −30.2 ± 0.7 | −28.0 ± 2.2 |
. | . | EBOV S142Q . | RAVV Q142S . | EBOV G87A . | RAVV A87G . |
---|---|---|---|---|---|
hNPC1/TET | Loop 1 | −5.4 ± 2.1 | −11.7 ± 1.7 | −11.2 ± 0.2 | −12.4 ± 0.6 |
Loop 2 | −16.6 ± 1.8 | −16.0 ± 0.8 | −19.0 ± 0.5 | −15.6 ± 1.5 | |
Total | −22.0 ± 3.8 | −27.7 ± 1.8 | −30.2 ± 0.7 | −28.0 ± 2.2 |
Abbreviations: EBOV, Ebola virus; Gpcl, cleaved glycoprotein; hNPC1, human NPC1; NPC1, Niemann-Pick C1; RAVV, Ravn virus.
aAverage binding free energies (in kcal/mol) were calculated by adding the energies of each residue of the loops.
Per-Residue Energy Contributions of Niemann-Pick C1 Loops and Mutant Glycoprotein Receptor Binding Site to the Binding Potential
The binding energy difference between GPs of EBOV and EBOV S142Q for the interaction with hNPC1/TET showed that the S142Q substitution caused unfavorable binding for most of the amino acid residues in both NPC1 loop 1 and loop 2 (Figure 3A, left panel). On the other hand, the RAVV Q142S substitution resulted in favorable binding for almost all the amino acid residues of the hNPC1/TET loops, and E426 and D501 had especially big energy differences of ≤ −2.0 kcal/mol (Figure 3A, middle panel). No significant energy difference was observed between EBOV and EBOV G87A in the binding capacity to hNPC1/TET loop 1 and loop 2 (data not shown). RAVV A87G, however, led to favorable binding for almost all the amino acid residues in the hNPC1/TET loops (Figure 3A, right panel). Although, interestingly, A87 of RAVV GP did not directly interact with loop 1, A87G resulted in favorable energy contributions to binding to hNPC/TET loop 1, especially to I419 and Q421 residues in loop 1.
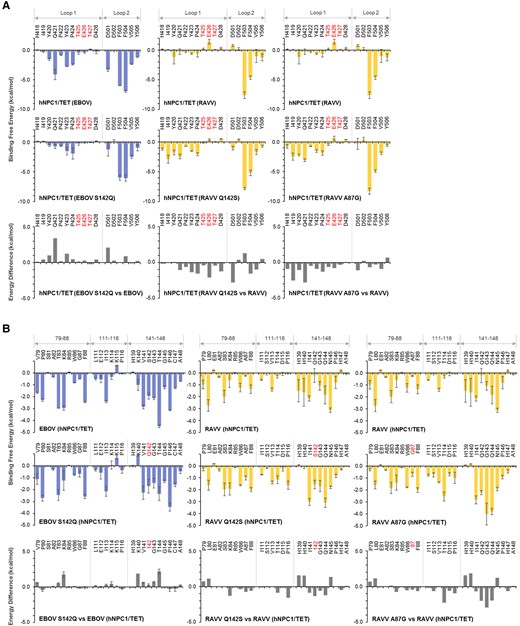
Per-residue decomposition analysis of human Niemann-Pick C1 (hNPC1) and hNPC1/TET for the interactions with mutant Ebola virus (EBOV) and Ravn virus (RAVV) glycoproteins (GPs). (A) Per-residue contributions to the binding free energies of loop 1 and loop 2 in the hNPC1/TET for the interactions with EBOV S142Q, RAVV Q142S, and RAVV A87G mutants were calculated using molecular mechanics/generalized Born surface area (MM/GBSA) free energy decomposition analysis. Each molecular dynamics (MD) simulation was conducted 3 times, and averages and standard errors (SE) are shown. Amino acid residues that are distinct from those of hNPC1 (ie, TET at positions 426–428) are shown in red. Differences in binding free energies were calculated by subtraction of the values of wild-type GPs from those of the respective GP mutants. (B) Per-residue contributions to the binding free energy of EBOV S142Q, RAVV Q142S, or RAVV A87G for the interaction with hNPC1/TET were calculated using MM/GBSA free energy decomposition analysis. The numbering scheme for GP was adapted from EBOV. Each MD simulation was conducted 3 times, and averages and SE are shown. Mutated amino acid residues were shown in red (ie, residues 87 and142 of RAVV GP and EBOV GP, respectively). Differences of binding free energies were calculated by subtraction of the values of wild-type GPs from those of the respective GP mutants.
We then examined the effects of the amino acid substitutions EBOV S142Q, RAVV Q142S, EBOV G87A, and RAVV A87G on the binding free energy to interact with hNPC/TET. Although the EBOV S142Q substitution had little effect on the energy contribution of residue 142 itself, it destabilized not only K140 and T144 near S142, but also K84 located opposite the Q142 in the NPC1 binding pocket (Figure 3B, left panel). Conversely, the substitution from Q to S at position 142 of RAVV GP resulted in a favorable energy contribution of residue 142 and its vicinity (Figure 3B, middle panel). It is interesting to note that these substitutions also affected the energy contribution of K84. None of the amino acid residues of EBOV S142Q were found to induce remarkable changes of the energy contribution (data not shown). The A87G substitution of RAVV GP had a little effect for itself, but it markedly increased the hNPC1/TET-binding potential of residues 141–144, located opposite residue 87 (Figure 3B, right panel).
DISCUSSION
We previously reported that cell lines expressing FBKT1 NPC1 or hNPC1/TET were susceptible to EBOV GP-mediated infection but much less susceptible to MARV GP-mediated infection than hNPC1-expressing cells [17]. In this study, MD simulations and binding free energy calculations were carried out to investigate the effects of these amino acid changes on intermolecular interactions between NPC1 and GP. Our simulations in the present study demonstrated that amino acid substitutions from SGA to TET at positions 425–427 of hNPC1 markedly decreased the binding affinity to RAVV GP, whereas the substitutions had little effect on the binding affinity to EBOV GP. These results well support the above-mentioned experimental observations in the previous study [17].
Our in silico analysis has revealed that G426 of hNPC1 loop 1 directly contacts S142 of EBOV and Q142 of RAVV GPs [33]. Therefore, it is likely that the presence of E426 in FBKT1 NPC1 loop 1 might cause impaired interactions with Q142 of MARV GP. Indeed, our simulations showed that amino acid swapping at position 142 between EBOV and RAVV GPs switched the binding affinity to hNPC1/TET. These results provided structural and energetic evidence for our previous experimental findings on the effects of the substitutions at position 142 of EBOV and MARV GPs [17]. In addition, the A87G substitution also affected the binding affinity to hNPC/TET (Figure 3A and B, right panels), also supporting our previous results [17]. It has been reported that a single mutation, V141A in EBOV GP, increases its binding affinity to NPC1 in a bat species (Eidolon helvum), suggesting that the V141A substitution likely creates a more sterically favorable (open) NPC1-binding site [34]. Similarly, the A87G substitution might affect the overall pocket volume and/or shape of the NPC1-binding pocket of MARV GP.
The binding structure of EBOV GPcl and hNPC1-C has provided important information for understanding the potential differences in host preferences among filoviruses, as well as for structure-based drug design [17, 25, 35–37]. However, there is no available information on the structure of the GP-NPC1 complex for other filovirus GPs and other animal NPC1 orthologues. By utilizing molecular modeling methods and MD simulations, we previously revealed that the binding structure of EBOV GPcl-hNPC1 differed from that of RAVV GPcl-hNPC1 [33]. This difference in binding modes between EBOV and RAVV GPs is likely due to the pocket volumes and/or shapes of their RBS exposed on GPcl [33]. This could be one of the mechanisms that may account for the differential tropism of EBOV and MARV depending on host cells.
CONCLUSIONS
The results obtained in the present computational study are well consistent with our previous experimental data [17] and provide structural and energetic bases for the differential binding modes of Ebola and Marburg virus glycoproteins to NPC1 of human and bat origins. Our approaches increase the understanding of the mechanisms underlying the host cell tropism of filoviruses.
Notes
Acknowledgments. We thank Mr. Kim Barrymore for editing the manuscript.
Author contributions. MI designed the study. MI performed study. MI, TH, and AT analyzed the data. MI and AT wrote the paper. All authors reviewed and edited the drafts and approved the final version.
Financial support. This work was supported by KAKENHI (20H03140, 21H02734, and 23H02371), a Grant-in-Aid for Scientific Research from the Ministry of Education, Culture, Sports, Science and Technology (MEXT), the Japanese Initiative for Progress of Research on Infectious Disease for Global Epidemics (J-PRIDE) (JP18fm0208101 and JP19fm0208001) and the Science and Technology Research Partnership for Sustainable Development (SATREPS) (JP22jm0110019) from the Japan Agency for Medical Research and Development (AMED), and Platform Project for Supporting Drug Discovery and Life Science Research (Basis for Supporting Innovative Drug Discovery and Life Science Research [BINDS]) from AMED under Grant Number JP20am0101114 (support number 0367).
Supplement sponsorship. This article appears as part of the supplement “10th International Symposium on Filoviruses.”
References
Author notes
Potential conflicts of interest. All authors: No reported conflicts of interest. All authors have submitted the ICMJE Form for Disclosure of Potential Conflicts of Interest. Conflicts that the editors consider relevant to the content of the manuscript have been disclosed.