-
PDF
- Split View
-
Views
-
Cite
Cite
Marie-Astrid Hoogerwerf, Jan Pieter R Koopman, Jacqueline J Janse, Marijke C C Langenberg, Roos van Schuijlenburg, Yvonne C M Kruize, Eric A T Brienen, Mikhael D Manurung, Petra Verbeek-Menken, Martha T van der Beek, Inge M Westra, Pauline Meij, Leo G Visser, Lisette van Lieshout, Sake J de Vlas, Maria Yazdanbakhsh, Luc E Coffeng, Meta Roestenberg, A Randomized Controlled Trial to Investigate Safety and Variability of Egg Excretion After Repeated Controlled Human Hookworm Infection, The Journal of Infectious Diseases, Volume 223, Issue 5, 1 March 2021, Pages 905–913, https://doi.org/10.1093/infdis/jiaa414
- Share Icon Share
Abstract
Controlled human hookworm infections could significantly contribute to the development of a hookworm vaccine. However, current models are hampered by low and unstable egg output, reducing generalizability and increasing sample sizes. This study aims to investigate the safety, tolerability, and egg output of repeated exposure to hookworm larvae.
Twenty-four healthy volunteers were randomized, double-blindly, to 1, 2, or 3 doses of 50 Necator americanus L3 larvae at 2-week intervals. Volunteers were monitored weekly and were treated with albendazole at week 20.
There was no association between larval dose and number or severity of adverse events. Geometric mean egg loads stabilized at 697, 1668, and 1914 eggs per gram feces for the 1 × 50L3, 2 × 50L3, and 3 × 50L3 group, respectively. Bayesian statistical modeling showed that egg count variability relative to the mean was reduced with a second infectious dose; however, the third dose did not increase egg load or decrease variability. We therefore suggest 2 × 50L3 as an improved challenge dose. Model-based simulations indicates increased frequency of stool sampling optimizes the power of hypothetical vaccine trials.
Repeated infection with hookworm larvae increased egg counts to levels comparable to the field and reduced relative variability in egg output without aggravating adverse events.
NCT03257072.
Hookworm infects about 230 million people worldwide [1]. Chronic infection causes iron deficiency anemia and protein loss among the world’s poorest [2], may impede children’s cognitive and physical development [3], and adversely affect pregnancy outcomes [4]. This results in high losses in annual productivity (estimated at between $7.5 and $138.9 billion) [5], perpetuating the poverty cycle [6]. The impact of periodical deworming, the cornerstone of current hookworm control programs, is impeded by high reinfection rates [7, 8] and the looming threat of anthelmintic resistance [9]. A safe and efficacious hookworm vaccine is therefore needed to aid hookworm control [10]; however, development is hampered by a lack of preclinical models since animal models do not reflect natural infection in humans.
Experimental infection of volunteers, so-called controlled human hookworm infection (CHHI) trials, could be an alternative method to screen novel products [11]. In other diseases, controlled human infection models have shown their usefulness in testing vaccine efficacy [12, 13]. However, their pertinence hinges on safety of the model, generalizability of results to natural infection, and adequately powered sample sizes.
Previous CHHI trials have shown that infections with 50 Necator americanus infectious larvae (L3) are well tolerated, with mild abdominal adverse events (AEs) and that skin eruptions can be alleviated by spreading the infectious dose over 4 sites [14, 15]. Resulting egg counts have varied, however, with some trials reaching 100% infectivity and egg counts comparable to field settings [15], with lower egg outputs in other studies [14, 16]. In addition, microscopic egg counts, the reference standard end point of CHHI trials, are highly variable [17], decreasing the power of hookworm infection studies to detect vaccine efficacy.
In a previous trial, our group used bayesian statistical modeling to describe the long-term kinetics of hookworm egg excretion [15] and found that 1 dose of 50L3 larvae resulted in egg counts plateauing about 13 weeks after infection, at a level comparable to low-endemic field settings. Although homogenizing the feces decreased the variability of egg counts in the same individual on the same day, considerable interindividual and intraindividual variation remained [15]. We hypothesized that repeated infection could increase egg outputs and potentially reduce variability relative to the mean without increasing AEs, enhancing the power of CHHI trials.
Here we report the outcome of a CHHI trial in which volunteers were exposed to multiple doses of N. americanus L3. Based on these data, we developed a bayesian model for the in-depth analysis of the variation in egg counts by dose group and performed hypothetical sample size calculations to determine the power of CHHI models in vaccine trials.
METHODS
This study was a randomized, double-blind, placebo-controlled trial investigating the safety and tolerability of cumulative doses of N. americanus larvae up to 3 × 50 L3 and the variability in egg output after repeated infection with N. americanus L3. The trial was approved by the Leiden University Medical Center institutional review board (no. NL59186.058.17) and registered at clinicaltrials.gov (NCT03257072).
Study Subjects
Healthy male and female volunteers aged 18–45 years were recruited from the Leiden area in January and February 2018. All volunteers provided written informed consent before inclusion. Inclusion and exclusion criteria are described in Supplement A. Participants were all confirmed hookworm negative by polymerase chain reaction (PCR) before inclusion. N. americanus L3 larvae were cultured from feces of chronically infected hookworm donors, according to the principles of Good Manufacturing Practice and following a procedure described elsewhere [15, 18].
Study Procedures
Volunteers were randomized 1:1:1 to receive 1, 2, or 3 doses of 50 N. americanus L3 at 2-week intervals, totaling a cumulative dose of 50, 100, or 150 L3 (Figure 1). Volunteers in the 50 or 2 × 50L3 group first received, respectively, 2 doses or 1 dose of placebo. Randomization was performed through a randomization list made by an independent researcher. Larval doses were suspended in sterile water and divided over 4 gauze pads, which were applied to both upper arms (doses of 10 L3) and calves (doses of 15 L3) and left for 1 hour. As placebo, gauze pads with sterile water were used. Investigators and participants were blinded to dose allocation.
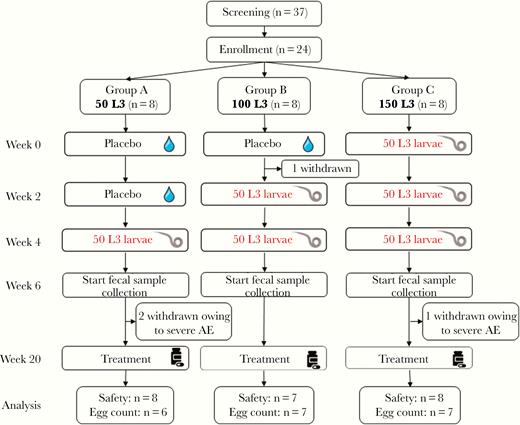
Volunteers were followed up weekly for 20 weeks. At each visit, AEs were collected and blood samples obtained for eosinophils and hemoglobin. Hemoglobin and eosinophils were measured using the Sysmex DI-60 automated cell morphology analyzer. Skin-related AEs, cough, sore throat, fever, and abdominal AEs were solicited at each visit. For every AE, timing, severity, and causality were recorded. AEs were scored as unrelated, unlikely, possibly, probably, or definitely related to hookworm infection and as mild (no interference with daily life), moderate (discomfort interfering with daily life), or severe (causing inability to perform usual daily activity). Two independent, blinded physicians divided the volunteers into 2 equal groups, the “high” and “low” abdominal AE groups, based on the safety data; division into groups was based on the severity, duration, and number of abdominal AEs, and consensus was reached for each volunteer.
At week 20 of the trial, all volunteers were treated with 400 mg of albendazole for 3 days. Two volunteers gave separate informed consent to postpone albendazole treatment and remain as donors for future studies. All other volunteers returned to the trial center on weeks 1, 2, 3, and 8 after treatment to ensure collection of AEs and complete cure of the infection.
Immunological Assays
Serum samples for analysis of antibodies were collected at weeks 0, 4, 8, 12, 16, 20, 23, and 28 of the trial. Hookworm-specific immunoglobulin (Ig) E, IgG, IgG1, and IgG4 were measured by means of enzyme-linked immunosorbent assay. Procedures are described in Supplement B. Specific antibody levels are expressed in arbitrary units per milliliter. Data were normalized with the baseline set at 0 and other measurements presented as arbitrary units per milliliter above baseline, peak value is defined as the largest rise in arbitrary units per millimeters compared to baseline.
Seroconversion was defined as any peak value (in arbitrary units per milliliter) 4 times above the standard deviation (SD). SD was determined in the week 4 samples of the 50L3 group, as baseline samples were normalized to 0 and at week 4 the 50L3 group had not yet been exposed, therefore enabling its use as a control group.
Parasitological Assays
Fecal samples were collected weekly from week 6 until week 20 of the trial and at 1 and 3 weeks after treatment. Kato-Katz slides were prepared fresh with 25 mg of stool, homogenized before preparation [18]. Two Kato-Katz slides per fecal sample were prepared, each read by a separate microscopist. The total number of eggs counted was multiplied by 20 and expressed as eggs per gram feces (epg) per collected stool sample. The method for detecting N. americanus DNA in stool with PCR is described in Supplement C.
Statistical Analysis
Similar to other proof-of-concept vaccine efficacy studies, groups of 8 subjects were chosen. This sample size would give 80% power to detect a 50% relative reduction in egg counts.
The primary end point was the frequency and severity of AEs per group, assessed in the intention-to-treat population. Differences between groups were analyzed using Kruskal-Wallis or Mann-Whitney U tests. The secondary end point was the difference in egg load between groups. The egg load was defined as the excretion of eggs in feces between weeks 16 and 20 of the trial, based on Kato-Katz slides. Mean egg counts per individual were calculated, and log-transformed values were compared using a 1-way analysis of variance. Differences between groups for eosinophilic response and antibodies were evaluated using a Kruskal-Wallis test. The correlation between Kato-Katz and PCR findings was assessed by means of Spearman ρ.
To compare the level and variability of egg counts between groups in more detail and perform power calculations for hypothetical vaccine trials, we developed a bayesian nonlinear regression model describing the egg count time series of each individual. The development of this model is described in Supplement D. After establishing the model and generating the model parameters, we performed power calculations, primarily assuming a reduction in egg loads of 50% in a vaccine group compared with the control group, with additional calculations for a 30%–70% effect size. Power was calculated with the aim to detect a significant difference, (defined as a P value < .05, based on Wilcoxon signed rank test) for the difference in individual average egg counts (averaged over days and Kato-Katz slides) between trial arms.
RESULTS
Study Flow
In February 2018, 24 volunteers were included in the trial. Trial flow is shown in Figure 1, and participant characteristics in Table 1. Despite randomization, more female than male volunteers were included in the 50L3 group. One volunteer withdrew informed consent after 1 week for reasons unrelated to the trial. Two volunteers (randomized to the 50L3 group) withdrew 6 weeks after first exposure, and the third volunteer (from the 3 × 50L3 group) withdrew at 9 weeks owing to severe abdominal AEs. All symptoms resolved with albendazole treatment. Safety data, eosinophils, and immunoglobulin results available until withdrawal for these volunteers have been included in the intention-to-treat analysis; however, because these volunteers withdrew before detection of eggs, their data are not included in the per-protocol analysis of egg excretion.
Participant Characteristics and Number and Duration of Adverse Events for Each Study Group
Characteristic . | Study Group . | . | . | . | P Valuea . |
---|---|---|---|---|---|
. | Total (n = 23) . | 50 L3 (n = 8) . | 2 × 50 L3 (n = 7) . | 3 × 50 L3 (n = 8) . | . |
Participant sex, no. | |||||
Female | 15 | 7 | 4 | 4 | .25 |
Male | 8 | 1 | 3 | 4 | |
Age, median (range), y | 22 (19–41) | 27.5 (19–38) | 21 (19–33) | 23 (19–41) | .33 |
History of travel to hookworm-endemic area, no. | 11 | 4 | 4 | 3 | .74 |
History of gastrointestinal disease, no. | 1 | 1b | 0 | 0 | .38 |
AEs per volunteer, median (range), no. | 16 (8–31) | 17 (11–24) | 17 (9–31) | 16 (8–27) | .90 |
Duration of rash, median (range), d | 34 (0–77) | 18.5 (0–49) | 37 (15–50) | 41.5 (21–77) | .09 |
Abdominal AEs, total no. (% of total) | 99 (100) | 32 (32) | 39 (39) | 28 (28) | .68 |
Related abdominal AEs per volunteer, median (range), no. | 4 (0–10) | 4 (0–6) | 4 (1–10) | 4 (0–7) | .68 |
Maximum severity of abdominal AEs, no. | |||||
None | 2 | 1 | 0 | 1 | .97 |
Mild | 7 | 2 | 2 | 3 | |
Moderate | 3 | 1 | 1 | 1 | |
Severe | 11 | 4 | 4 | 3 | |
Duration of abdominal AEs, median (range), d | 22 (0–125) | 21.5 (0–38) | 29 (4–125) | 23.5 (0–110) | .64 |
Volunteers in the “high” abdominal AE group, no.c | 12 | 4 | 4 | 4 | .95 |
Characteristic . | Study Group . | . | . | . | P Valuea . |
---|---|---|---|---|---|
. | Total (n = 23) . | 50 L3 (n = 8) . | 2 × 50 L3 (n = 7) . | 3 × 50 L3 (n = 8) . | . |
Participant sex, no. | |||||
Female | 15 | 7 | 4 | 4 | .25 |
Male | 8 | 1 | 3 | 4 | |
Age, median (range), y | 22 (19–41) | 27.5 (19–38) | 21 (19–33) | 23 (19–41) | .33 |
History of travel to hookworm-endemic area, no. | 11 | 4 | 4 | 3 | .74 |
History of gastrointestinal disease, no. | 1 | 1b | 0 | 0 | .38 |
AEs per volunteer, median (range), no. | 16 (8–31) | 17 (11–24) | 17 (9–31) | 16 (8–27) | .90 |
Duration of rash, median (range), d | 34 (0–77) | 18.5 (0–49) | 37 (15–50) | 41.5 (21–77) | .09 |
Abdominal AEs, total no. (% of total) | 99 (100) | 32 (32) | 39 (39) | 28 (28) | .68 |
Related abdominal AEs per volunteer, median (range), no. | 4 (0–10) | 4 (0–6) | 4 (1–10) | 4 (0–7) | .68 |
Maximum severity of abdominal AEs, no. | |||||
None | 2 | 1 | 0 | 1 | .97 |
Mild | 7 | 2 | 2 | 3 | |
Moderate | 3 | 1 | 1 | 1 | |
Severe | 11 | 4 | 4 | 3 | |
Duration of abdominal AEs, median (range), d | 22 (0–125) | 21.5 (0–38) | 29 (4–125) | 23.5 (0–110) | .64 |
Volunteers in the “high” abdominal AE group, no.c | 12 | 4 | 4 | 4 | .95 |
Abbreviation: AEs, adverse events.
aP values based on Kruskal-Wallis test.
bGastric reflux.
cDivision into “high” and “low” abdominal AE groups was based on the severity, duration, and number of abdominal AEs.
Participant Characteristics and Number and Duration of Adverse Events for Each Study Group
Characteristic . | Study Group . | . | . | . | P Valuea . |
---|---|---|---|---|---|
. | Total (n = 23) . | 50 L3 (n = 8) . | 2 × 50 L3 (n = 7) . | 3 × 50 L3 (n = 8) . | . |
Participant sex, no. | |||||
Female | 15 | 7 | 4 | 4 | .25 |
Male | 8 | 1 | 3 | 4 | |
Age, median (range), y | 22 (19–41) | 27.5 (19–38) | 21 (19–33) | 23 (19–41) | .33 |
History of travel to hookworm-endemic area, no. | 11 | 4 | 4 | 3 | .74 |
History of gastrointestinal disease, no. | 1 | 1b | 0 | 0 | .38 |
AEs per volunteer, median (range), no. | 16 (8–31) | 17 (11–24) | 17 (9–31) | 16 (8–27) | .90 |
Duration of rash, median (range), d | 34 (0–77) | 18.5 (0–49) | 37 (15–50) | 41.5 (21–77) | .09 |
Abdominal AEs, total no. (% of total) | 99 (100) | 32 (32) | 39 (39) | 28 (28) | .68 |
Related abdominal AEs per volunteer, median (range), no. | 4 (0–10) | 4 (0–6) | 4 (1–10) | 4 (0–7) | .68 |
Maximum severity of abdominal AEs, no. | |||||
None | 2 | 1 | 0 | 1 | .97 |
Mild | 7 | 2 | 2 | 3 | |
Moderate | 3 | 1 | 1 | 1 | |
Severe | 11 | 4 | 4 | 3 | |
Duration of abdominal AEs, median (range), d | 22 (0–125) | 21.5 (0–38) | 29 (4–125) | 23.5 (0–110) | .64 |
Volunteers in the “high” abdominal AE group, no.c | 12 | 4 | 4 | 4 | .95 |
Characteristic . | Study Group . | . | . | . | P Valuea . |
---|---|---|---|---|---|
. | Total (n = 23) . | 50 L3 (n = 8) . | 2 × 50 L3 (n = 7) . | 3 × 50 L3 (n = 8) . | . |
Participant sex, no. | |||||
Female | 15 | 7 | 4 | 4 | .25 |
Male | 8 | 1 | 3 | 4 | |
Age, median (range), y | 22 (19–41) | 27.5 (19–38) | 21 (19–33) | 23 (19–41) | .33 |
History of travel to hookworm-endemic area, no. | 11 | 4 | 4 | 3 | .74 |
History of gastrointestinal disease, no. | 1 | 1b | 0 | 0 | .38 |
AEs per volunteer, median (range), no. | 16 (8–31) | 17 (11–24) | 17 (9–31) | 16 (8–27) | .90 |
Duration of rash, median (range), d | 34 (0–77) | 18.5 (0–49) | 37 (15–50) | 41.5 (21–77) | .09 |
Abdominal AEs, total no. (% of total) | 99 (100) | 32 (32) | 39 (39) | 28 (28) | .68 |
Related abdominal AEs per volunteer, median (range), no. | 4 (0–10) | 4 (0–6) | 4 (1–10) | 4 (0–7) | .68 |
Maximum severity of abdominal AEs, no. | |||||
None | 2 | 1 | 0 | 1 | .97 |
Mild | 7 | 2 | 2 | 3 | |
Moderate | 3 | 1 | 1 | 1 | |
Severe | 11 | 4 | 4 | 3 | |
Duration of abdominal AEs, median (range), d | 22 (0–125) | 21.5 (0–38) | 29 (4–125) | 23.5 (0–110) | .64 |
Volunteers in the “high” abdominal AE group, no.c | 12 | 4 | 4 | 4 | .95 |
Abbreviation: AEs, adverse events.
aP values based on Kruskal-Wallis test.
bGastric reflux.
cDivision into “high” and “low” abdominal AE groups was based on the severity, duration, and number of abdominal AEs.
Clinical Data
No serious AEs occurred. Most common related AEs were pruritus and rash during the first weeks after exposure (n = 23) and abdominal AEs from week 2 after first infection (n = 21). Two volunteers reported no abdominal symptoms, and 5 reported only mild abdominal bloating or flatulence for <5 days. Other gastrointestinal symptoms started from week 2 after exposure, peaked at weeks 4 and 5, and resolved 8 weeks after first infection in the majority of volunteers (13 of 16). Symptoms in the remaining 3 volunteers resolved with albendazole treatment. Based on the combination of severity and duration of AEs, 12 volunteers were classified in the “high” and 11 in the “low” abdominal AE group.
There were no significant differences in the number or severity of AEs between study groups (Table 1); the number of volunteers experiencing grade 3 AEs or any abdominal AE were similar across study groups. Hemoglobin levels stayed stable throughout the trial (Figure 2A). Eosinophil counts increased in all groups, starting 3 weeks after first exposure, with a peak at week 6 after initial infection (Figure 2B). There was no difference in mean peak eosinophil counts between groups (P = .4).
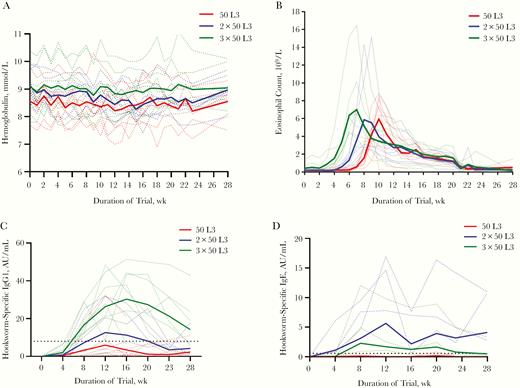
A, B, Hemoglobin levels (A) and eosinophil counts (B) for individual volunteers (dotted lines) and mean for each study group (continuous lines). C, D, Immunoglobulin (Ig) G1 antibody (C) and IgE (D) responses for individual volunteers (dotted lines) and mean for each study group (continuous lines); horizontal dotted line represents threshold for seroconversion (increase from baseline to 4 times the standard deviation). Abbreviation: AU, arbitrary units.
Hookworm-specific antibodies increased with additional doses and peaked at week 12 or 16 of the trial, although there was a large variation between individuals. Increases in IgG1 levels differed significantly between dose groups (P = .01 for peak IgG1 value between groups) (Figure 2C). IgG1 seroconversion was reached in 3, 4, and 7 volunteers for the 50 L3, 2 × 50 L3, and 3 × 50 L3 groups, respectively, which did not reach statistical significance (χ 2 test, P = .12). For IgE, seroconversion was observed in 3 volunteers in the 2 × 50 L3 group and 2 in the 3 × 50 L3 group. (Figure 2D). IgG4 responses were less pronounced than for IgG1 levels, did not show clear differences between dose groups, and reached seroconversion in 2 (50 L3), 3 (2 x 50 L3), and 2 (3 x 50 L3) volunteers (Supplementary Figure 1A). Total IgG levels are displayed in Supplementary Figure 1B.
Parasitological Analysis
Volunteers in the 50 L3 group secreted hookworm eggs, as seen with Kato-Katz slides, a median of 7 weeks after first infection (range, 5–8 weeks), compared with 8 weeks (range, 7–9 weeks) in the 2 × 50 L3 and 3 × 50 L3 groups (P = .68). Egg loads differed significantly between groups; the geometric means of the mean counts per individual were 697 (95% confidence interval, 228–2131), 1668 (979–2840), and 1914 (1455–2517) epg, respectively, for the 50 L3, 2 × 50L3, and 3 × 50 L3 groups (1-way analysis of variance, P = .04) (Figure 3). Post hoc testing for between group difference showed weak differences between the 50 L3 group and the other 2 groups (P = .1 [50 L3 vs 2 × 50L3] and P = .05 [50 L3 vs 3 × 50 L3]) and no difference between the 2 × 50 and 3 × 50 L3 groups (P = .6) (Figure 3).
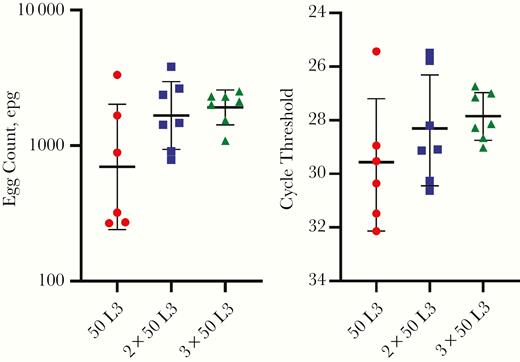
Individual mean Kato-Katz slide measurements (left) and cycle threshold values (right) at weeks 16–20 of the trial. Red circles represent the 50L3 group; blue squares, 2 × 50L3 group; green triangles, 3 × 50L3 group; lines, geometric means; and error bars geometric standard deviations. Abbreviation: epg, eggs per gram feces.
Infection intensity as determined with PCR mirrored these findings (P = .02) and was strongly correlated with counts based on Kato-Katz slides (P ≤ .001; ρ = –0.79) (Supplementary Figure 2). PCR and Kato-Katz slide counts were all negative 3 weeks after treatment.
Bayesian Statistical Modeling
Using bayesian statistical modeling, we first assessed the relative contributions of each L3 dose to the overall egg load. Allowing dose contributions to vary freely between all groups confirmed an equal contribution of the first and second dose of L3 and a lesser contribution of the third dose (estimated at a factor of 0.4 relative to a single dose; 95%-bayesian credible interval [BCI], .01–1.0). Next, when we allowed the level of temporal overdispersion (ie, daily variation relative to the mean) to vary freely between all 3 groups, the level of overdispersion was a factor of 1.9 higher (95% BCI, 1.0–3.2) in the single-dose compared with the 2-dose group; there was no significant difference in temporal overdispersion (and thus relative variation) between the 2- and 3-dose groups (difference factor, 1.1; 95% BCI, .5–1.9).
We therefore further simplified the model assuming that temporal overdispersion differed only between the single dose group (k = 3.2; 95% BCI, 2.0–4.9) compared with the other 2 dose groups (k = 5.6; 4.1–7.5; ie, different by a factor of 1.9, [95% BCI, 1.01–3.05]). We verified that random effects for the plateau level and timing of increases in egg counts within each individual were uncorrelated (Supplementary Figure 3).
We estimated that 1, 2, or 3 doses of 50 L3 resulted in a posterior mean egg load at plateau levels of 760 (95% BCI, 640–840), 1520 (1360–1680), and 1800 (1560–2080) epg, respectively, corresponding with the descriptive statistics of egg loads described elsewhere (Figure 4). The rise in egg counts due to each single dose reached 50% of its maximum level at 67 days ( approximately 10 weeks) after exposure (95% BCI, 63–70 days) and 97.5% of its maximum after another 18 days (15–22 days). Interindividual variation in the timing of the initial rising phase was minimal, with an SD on the logarithmic scale of 0.08 (95% BCI, .05–.11). A summary of the final model estimated parameters is provided in Supplementary Table 1, and estimated and measured individual egg counts in Supplementary Figure 4.
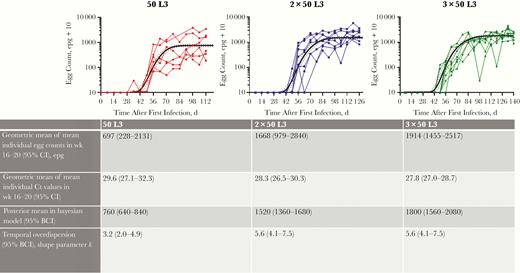
Egg counts as observed and predicted by a bayesian nonlinear regression model, for 50L3 (left), 2 × 50L3 (middle), and 3 × 50L3 (right) groups. Solid line in each panel represents the group average; dashed lines, upper and lower limits for the 95% bayesian credible interval (BCI) of the point estimate. Table below graphs shows mean values for Kato-Katz slides and polymerase chain reaction, bayesian posterior means, and shape parameter k for temporal overdispersion; for the latter, higher values indicate less variability relative to the mean. Abbreviations: BCI, bayesian credible interval; CI, confidence interval; Ct, cycle threshold; epg, eggs per gram feces.
Correlation Between AEs, Egg Counts, and Antibody Response
Volunteers with a higher egg output had higher mean hookworm-specific IgG1 levels (egg load, R = 0.537 and P = .01; cycle threshold [Ct], R = –0.586 and P = .007 for) but not higher IgE or IgG4 levels or eosinophil counts. Volunteers with more abdominal AEs had higher eosinophil counts (mean, 9.1 × 109 vs 4.4 × 109 in “high” AEs versus “low” AEs; P = .003), but an equal number of eggs (geometric mean, 914 vs 1086 epg; P = .6) (Figure 5). Antibody levels were not correlated with AEs or eosinophil counts.
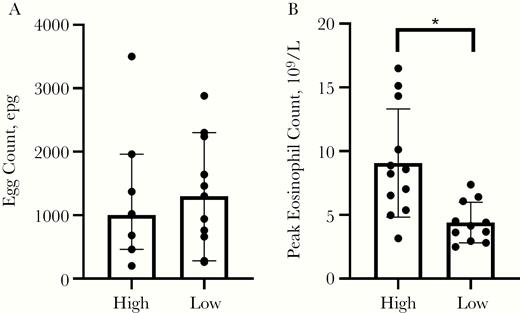
Comparison of volunteers in the upper (“high”) and lower (“low”) groupings according to the severity, duration, and number of abdominal adverse events, for both egg load (A; shown as median with 95% CI) and eosinophilia (B; shown as mean with standard deviation). *P = .003. Abbreviations: CI, confidence interval; epg, eggs per gram feces.
Power Calculations
With the bayesian nonlinear regression model, power calculations were performed by repeatedly simulating synthetic data for a 2-armed trial, assuming the use of a vaccine that reduces egg counts by 50% compared with placebo. Sampling of stools was assumed to start during the stable phase in week 15 after first infection. Although multiple doses of L3 increase study power somewhat (owing to higher egg counts in the control group and lower random daily variation within individuals), power is driven mostly by the number of repeated samples collected at weekly intervals (Figure 6). A power of >80% can be reached in groups as small as 6 volunteers if samples are taken for 5 weeks with 2 doses of 50 L3. In contrast, a power of only 60% is reached with a single sample in groups of 15 volunteers with 3 infectious doses. Assessing multiple Kato-Katz slides per sample does not improve power compared with a single slide (Figure 6). The power calculation was repeated with an expected vaccine efficacy of 30%–70%. This showed that with sufficiently frequent sampling even at only 30%, efficacy groups of 15 participants can reach 80% power (Supplementary Figure 5).
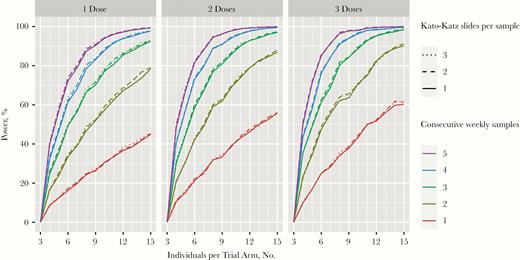
Power calculation using bayesian modeling of egg counts showing the number of individuals per trial arm in a vaccine trial, assuming 50% reduction in egg counts in the vaccine versus the placebo group, after 1 (left), 2 (middle), or 3 (right) doses of 50L3. Colors represent the numbers of weekly samples obtained with the top line in each graph representing 5 samples and each subsequent lower line one sampling less, the lowest line represents 1 sample.
Discussion
This dose escalation study showed that higher cumulative doses of hookworm L3 did not enhance AEs, which displayed high interindividual variability and a reasonable tolerability profile. Repeated infectious doses resulted in an increase in total egg counts and decreased relative variability in egg excretion. Power calculations based on a bayesian nonlinear regression model showed that repeated sampling is the most important parameter determining the power of the CHHI model to detect potential vaccine efficacy.
Volunteers reported a considerable number of abdominal AEs, with 3 volunteers receiving rescue treatment early owing to severe abdominal AEs. However, these events do not seem to be related to L3 dose, because 2 of the rescued volunteers were in the lowest dose group. Within each dose group we found considerable interindividual variation in the number and severity of AEs. Severity of AEs correlated with eosinophilic response, which both peak around week 6 after infection. We hypothesize that this may reflect symptomatic eosinophilic enteritis, which has previously been associated with hookworm attrition [19], but we could not establish a relationship between egg load and eosinophil counts.
Larval dose and eosinophilic response were not related; however, there was a clear relationship between dose and humoral responses to the larvae. Remarkably, we found IgG1 to be the most pronounced responding immunoglobulin subtype in this acute infection, contrasting epidemiological studies where IgE and IgG4 are hallmarks of active chronic infection [20]. IgE has been observed to develop only after repeated exposure [20] and may confer protection [21]. We did observe an IgE response in some volunteers after repeated exposure, but, given the limited response in only a few volunteers, we were not able to confirm any protective effect.
PCR results showed a similar pattern of increased infectious loads with higher cumulative infectious doses and showed good correlation with Kato-Katz slide outcomes. However, the high SD between 2.0 and 0.9 Ct, corresponding to a variation double or quadruple the amount of DNA, indicates high variability in PCR outcomes. This may be caused by both variability in the egg excretion itself and by differences in the number of DNA copies per egg, because an N. americanus egg may be in a 2–16-cell stage, increasing possible sources for variation [22]. In addition to the more difficult field applicability of PCR techniques and supplementary variability of Ct values between laboratories [22], this underscores that microscopic techniques still remain the cornerstone of hookworm diagnosis and highlights the necessity to improve the power and accuracy of diagnostic outcome measures.
Repeated dosing resulted in egg counts that are more representative of infection levels in endemic areas, with mean counts only slightly below the World Health Organization threshold for moderate infection (2000 epg) [23]. These egg counts are higher than previously reported using a single inoculation [14, 15], enabling a better comparison with natural infection. However, the third dose does not seem to add significantly to the total egg output compared with 2 doses. It is possible that repeated exposure induces some immunity against the larvae of the subsequent infection, resulting in fewer surviving worms, or that competition for nutrients and feeding sites occurs as the number of larvae being established in the intestine simultaneously is higher than in a natural, trickled infection.
The high variability of egg secretion complicates the use of the CHHI model for vaccine testing. We therefore used bayesian statistical modeling to more accurately estimate individual and population egg outputs and to better describe the variability in egg counts. The use of bayesian modeling carries the advantage of a robust inference and the possibility of carrying uncertainties of parameter estimates into power calculations, providing more accurate power estimates. This confirmed that the third infectious dose has a very limited contribution to the egg count plateau and to the reduction of relative variability, compared with 2 doses. Consequently, the use of the 2 × 50L3 dose seems the most rational option for future use in CHHI, because it leads to egg counts comparable to endemic areas, with reduced relative variability compared with a single dose and without aggravating AEs.
The power calculation based on the bayesian statistical model underscores the importance of repeated sampling over time, which has more impact on study power than the number of infections. More importantly, if we assume that variation between sampling on consecutive days is similar as variation within weekly samples and therefore take 5 consecutive samples during the first weeks of the plateau phase (from week 13 after infection), the duration of a CHHI trial may be substantially shortened from our current 20-week follow-up—for example, to 15 weeks. Naturally, sample sizes in future vaccine studies may be further increased to also detect differences in number or severity of AEs.
Owing to the staggered design, the timing of the first infection varied between different dose groups. However, the egg load was analyzed at the plateau level for all groups, ensuring comparability of outcomes. The study was underpowered to detect a difference in AEs. Given the remarkably equal distribution of AEs in the groups, significant differences seem unlikely. Although study set-up cannot mimic natural repeated infection with very frequent exposure to small inoculae and group sizes were diminished owing to withdrawal of 4 volunteers, this study remains the largest to date to investigate any kind of repeated exposure to hookworm infection.
In conclusion, the current trial has further advanced the CHHI model by introducing repeated challenge in the model and underscoring the importance of repeated sampling after development of the plateau phase. These findings are an important step in the applicability of the CHHI model for future vaccine and drug research.
Supplementary Data
Supplementary materials are available at The Journal of Infectious Diseases online. Consisting of data provided by the authors to benefit the reader, the posted materials are not copyedited and are the sole responsibility of the authors, so questions or comments should be addressed to the corresponding author.
Notes
Acknowledgments. The authors thank Chelsea Gootjes and Mark Dekker for their contribution to the hookworm culture and for preparing and reading Kato-Katz slides, Beckley Nosoh for performing the polymerase chain reaction assays, and Martijn Bauer for his advice as safety monitor of this study.
Disclaimer. The funders had no role in the study design, data collection and analysis, decision to publish, or preparation of the manuscript. The views, opinions, assumptions or any other information set out in this article are solely those of the authors and should not be attributed to the funders or any person connected with them.
Financial support. This work was supported by the Dioraphte Foundation and the Dutch Research Council (grant 016.Veni.178.023 to L. E. C.).
Potential conflicts of interest. All authors: No reported conflicts. All authors have submitted the ICMJE Form for Disclosure of Potential Conflicts of Interest. Conflicts that the editors consider relevant to the content of the manuscript have been disclosed.