-
PDF
- Split View
-
Views
-
Cite
Cite
Anna C Dilger, Bradley J Johnson, Paul Brent, Richard L Ellis, Comparison of beta-ligands used in cattle production: structures, safety, and biological effects, Journal of Animal Science, Volume 99, Issue 8, August 2021, skab094, https://doi.org/10.1093/jas/skab094
- Share Icon Share
Abstract
Technologies that increase the efficiency and sustainability of food animal production to provide meat for a growing population are necessary and must be used in a manner consistent with good veterinary practices, approved labeled use, and environmental stewardship. Compounds that bind to beta-adrenergic receptors (β-AR), termed beta-adrenergic receptor ligands (β-ligands), are one such technology and have been in use globally for many years. Though all β-ligands share some similarities in structure and function, the significance of their structural and pharmacological differences is sometimes overlooked. Structural variations in these molecules can affect absorption, distribution, metabolism, and excretion as well as cause substantial differences in biological and metabolic effects. Several β-ligands are available for use specifically in cattle production. Ractopamine and zilpaterol are beta-adrenergic agonists approved to increase weight gain, feed efficiency, and carcass leanness in cattle. They both bind to and activate β1- and β2-AR. Lubabegron is a newly developed selective beta-adrenergic modulator with unique structural and functional features. Lubabegron displays antagonistic behavior at the β1- and β2-AR but agonistic behavior at the β3-AR. Lubabegron is approved for use in cattle to reduce ammonia emissions per unit of live or carcass weight. Additionally, lubabegron can withstand prolonged use as the β3-AR lacks structural features needed for desensitization. Due to these unique features of lubabegron, this new β-ligand provides an additional option in cattle production. The individual properties of each β-ligand should be considered when making risk management decisions, as unique properties result in varying human food safety profiles that can determine appropriate safe β-ligand use.
Introduction
The importance of technologies to increase the efficiency of meat production and reduce its environmental impact cannot be overstated. Global population increases coupled with economic development in several parts of the world have increased the demand for animal protein (Speedy, 2003). Meanwhile, social and environmental phenomena, such as climate change and urban sprawl, threaten the availability of land and resources to produce food for this growing world demand (Zhang and Cai, 2011). Efficiency, often measured as the amount of feed needed to produce a unit of output (meat or milk), is a key determinant of environmental sustainability (Tilman et al., 2002). Furthermore, technologies can also be used to mitigate environmental emissions. These factors reinforce the importance of responsible technology use to improve environmental stewardship of livestock production. The use of technologies in food-producing animals is not without risk. The safe use of these compounds requires both animal welfare and food safety considerations. Therefore, ethical implementation of technologies developed to improve efficiency or reduce environmental emissions in food production must be done with safety as a top priority.
Phenethanolamines (Phenyl-CH(OH)-CH2-NH-R) have a prominent role in human physiology and as technologies in food animal production (Smith, 1998). These compounds bind to and elucidate effects from beta-adrenergic receptors (β-AR) and, therefore, can be broadly referred to as beta-adrenergic receptor ligands (β-ligands). Those that activate β-AR are termed beta-adrenergic agonists (β-AA), whereas those that block the action of β-AR are called β-antagonists or more commonly β-blockers. Another class of β-ligands, selective beta-adrenergic receptor modulators (SβM), has been developed to have agonist effects on specific β-AR subtypes while having blocking effects on other β-AR subtypes.
Some synthetic β-AA have been developed for use in food-producing animals. These include ractopamine for use in cattle, swine, and turkeys, and zilpaterol for use in cattle (Centner et al., 2014). In general, these β-AA are approved to increase efficiency and weight gain in livestock including cattle (FDA, 1998, 2003, 2006, 2008). Recently, another β-ligand, lubabegron, was approved for use in cattle (FDA, 2018). This compound, like other β-ligands, binds to β-AR, but it is not simply a β-AA. Lubabegron was developed to have a modulating effect on β-AR (i.e., an agonistic effect on the β3-AR but an antagonistic effect on β1-AR and β2-AR). Lubabegron was also developed to selectively bind to β-AR and not bind to non-β-AR, such as alpha-adrenergic, dopamine D1, dopamine D2, 5-hydroxytryptamine 2 (5-HT2), histamine H1, benzodiazepine, and γ-aminobutyric acid (GABAA), and muscarinic receptors (FDA, 2018). Thus, lubabegron is more appropriately described as an SβM. Lubabegron is classified as a beta-adrenergic agonist/antagonist by the U.S. Food and Drug Administration (FDA) Center for Veterinary Medicine (CVM). In addition to its unique mode of action, lubabegron is not approved to increase growth or carcass leanness. Instead, lubabegron reduces ammonia gas emissions from cattle per unit live or carcass weight.
This report highlights the biology, physiology, chemistry, and pharmacology of β-ligands, concentrating specifically on those used in cattle production. It is the goal of this paper to differentiate the characteristics of the β-ligands used in food animal production and discuss the safe use of these compounds as tools to increase efficiency and lessen the environmental impact of cattle production.
Use of β-Ligands in Human Medicine and Animal Agriculture
Human medicinal use
Phenethanolamines exhibit seven broad categories of pharmacological activities (e.g., sympathomimetic drugs, such as salbutamol or isoproterenol, or sympathomimetic blocking drugs, such as propranolol). Not all sympathomimetic drugs show each of the types of actions to the same degree, but many differences in their effects are only qualitative (Hilal-Dandan and Brunton, 2014). The pharmacological actions include:
A peripheral excitatory action on certain types of smooth muscle, such as those in blood vessels supplying the skin, kidney, and mucous membranes, and on gland cells, such as those in salivary and sweat glands.
A peripheral inhibitory action on certain other types of smooth muscle, such as those in the wall of the gut, in the bronchial tree, and in blood vessels supplying the skeletal muscle.
A cardiac excitatory action that increases heart rate and force of contraction.
Metabolic actions, such as an increase in the rate of glycogenolysis in the liver and muscle and liberation of free fatty acids from adipose tissue.
Endocrine actions, such as modulation (increasing or decreasing) of the secretion of insulin, renin, and pituitary hormones.
Actions in the central nervous system, such as respiratory stimulation, an increase in wakefulness and psychomotor activity, and a reduction in appetite.
Pre-junctional actions that either inhibit or facilitate the release of neurotransmitters, the inhibitory action being physiologically more important.
Biologically active derivatives of phenethanolamines began to emerge in the 1970s and 1980s as catecholamine derivatives and were initially sought for use as human drugs based on their pharmacological properties as bronchodilators. At present, β-AA have been investigated for multiple therapeutic applications, including treatment of asthma (Spitzer et al., 1992), as an aid for obesity and diabetes (Arch and Wilson, 1996), to halt or slow pre-term labor (Motazedian et al., 2010), and to alleviate symptoms of overactive bladder (Sacco and Bientinesi, 2012). For many applications, β-AA are used to relax smooth muscle. For example, the use of β-AA in treating asthma relaxes the smooth muscle in airways alleviates difficult breathing (Barisione et al., 2010). Mirabegron, the only approved β3-adrenergic receptor agonist, is used to treat overactive bladder by relaxing smooth muscle in the urethra (Alexandre et al., 2016). In the treatment of obesity and diabetes, β-AA stimulate the thermogenic activity of adipose tissue (Weyer et al., 1999) and uncouple substrate metabolism from adenosine triphosphate (ATP) production resulting in energy wastage.
Beta-adrenergic antagonists (β-blockers) are used to treat various cardiac diseases, such as hypertension, ischemic heart disease, and congestive heart failure (Bristow, 2003). Stimulation of β1-AR with β-AA increases cardiac muscle contractility (inotropic effect) and heart beat frequency (chronotropic effect; Brodde and Michel, 1999) to combat weakening of the myocardium or inadequate cardiac output due to high blood pressure. While endogenous stimulation of β1-AR is a short-term coping mechanism during heart failure, chronic stimulation actually hastens the progression of congestive heart failure (Lohse et al., 2003; Freedman and Lefkowitz, 2004). Therefore, antagonists of β1-AR are used to treat heart failure (Bristow, 2003).
Animal agriculture use
In livestock, the efficacy of β-AA to enhance animal growth performance, muscle accretion, and carcass yield has been extensively researched since the late 1970s. In 1999, ractopamine, a β-AA, was approved for use in pigs by the FDA with increased carcass leanness, increased average daily gain (ADG), and improved feed efficiency as the label indication (FDA, 1999). Later, it was approved by the FDA for similar claims in both cattle (FDA, 2003) and turkeys (FDA, 2008). Another β-AA, zilpaterol, was approved by the FDA in 2006 for use in cattle to increase the rate of weight gain, improve feed efficiency, and increase carcass leanness (FDA, 2006). Lubabegron was approved by the FDA in 2018 for use in cattle to reduce the environmental impact of production by reducing ammonia emissions per unit of live or carcass weight (FDA, 2018). These β-ligand products, when used safely and appropriately, can enhance growth and efficiency, increase lean meat yield, and reduce environmental impacts of meat production. Additionally, in the European Union, Australia, and some other countries, clenbuterol has limited therapeutic use for tocolysis in cattle and bronchodilating treatments in horses (EMEA, 2000).
Misuse of β-AA in animal production
Early clenbuterol studies in animals noted enlargement of striated muscle mass and decreased body fat deposition (Ricks et al., 1984; Petrou et al., 1995). Therefore, clenbuterol as well as a number of analogs (e.g., salbutamol) began to be used in food-producing animals without regulatory approvals. In the early 1990s, it was discovered that residues of these compounds in the tissues of illegally treated farm animals caused symptoms of acute poisoning in people in Spain, Italy, and France, with many of the cases requiring hospitalization for adverse cardiopulmonary effects (Martínez-Navarro, 1990; Pulce et al., 1991). Thus, undesirable effects stemming from their metabolism and rate of excretion have precluded their approval by the FDA (Mitchell and Dunnavan, 1998). Unfortunately, illegal use of clenbuterol continues with reports of misuse in Mexico (Valladares-Carranza et al., 2014), in show animals (Wood et al., 2010), and hospitalizations of people in China in 2006 and 2009 after consumption of pig meat and organs containing clenbuterol residues (CNN, 2009). More recently, in both 2006 and 2009, regulatory agencies such as the FDA, USDA Food Safety Inspection Service, and others in the European Union have implemented surveillance and compliance programs, which utilize sensitive laboratory methods to combat the misuse of clenbuterol and other illegal β2-AA. However, the documented residue detection incidence suggests a shift in the last decade to areas of Mexico, Central America, China, and possibly Indonesia (Barbosa et al., 2005; Ramos et al., 2009; Valladares-Carranza et al., 2014; Widiastuti and Anastasia, 2018; Xu et al., 2019). Thus, the illegal use of clenbuterol continues to be a global issue.
Characteristics of β-Ligands
Characteristics common to β-ligands
All β-ligands share some common structural features and signaling pathways. For example, many β-ligands contain the basic structure of the neurotransmitters epinephrine and norepinephrine (e.g., phenyl-CH(OH)-CH2-NH-R). This structure contains an aromatic benzene ring (with usually one or two substituents) attached to the ethanolamine structure on the carbon atom containing the hydroxyl substituent. The amino nitrogen may have one or more substituents attached to it. Structures of some representative β-ligands considered or used in veterinary medicine are shown in Figure 1 and have been reviewed by Smith (1998).
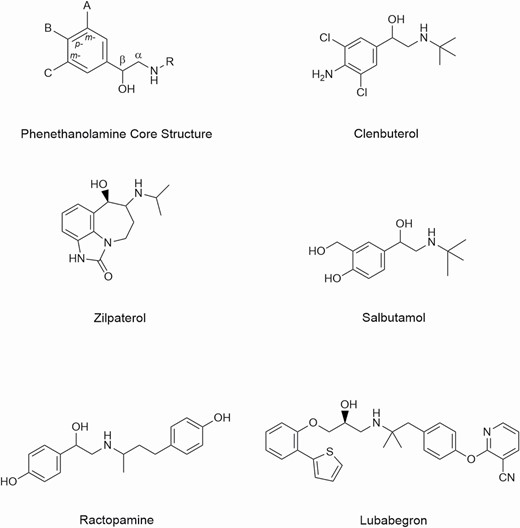
In terms of signaling, all β-ligands bind to β-AR. Binding of β-AA results in the activation of β-AR, which is coupled to the G stimulatory (Gs) protein. Activation of the Gs protein, in turn, activates adenylyl cyclase (Mersmann, 1998; Moody et al., 2000; Anderson et al., 2004). Adenylyl cyclase then converts ATP to cyclic adenosine monophosphate (cAMP; Mersmann, 1998; Moody et al., 2000; Anderson et al., 2004). Cyclic AMP then binds to the regulatory subunit of protein kinase A (PKA), and its catalytic subunit is released. PKA regulates many intracellular enzymes and regulatory factors that are significant determinants of metabolic regulation (Mersmann, 1998; Moody et al., 2000; Anderson et al., 2004). Conversely, binding of β-blockers does not elicit these signals; instead, β-blockers prevent the binding of β-AA and resulting in the reduction of downstream effects of β-AR activation.
Characteristics that allow differentiation of β-ligands
Beta-ligands have historically contained the same core structure (i.e., the phenethanolamine moiety), as is the case for ractopamine and clenbuterol (Figure 1). However, lubabegron contains a phenylethanolamine structure. Beta-ligands also differ in their chemical substituents on this core structure. These added chemical substituent structural features allow for the characterization of their unique pharmacological properties. The physical, chemical, and stereographic nature of β-ligands influences their Absorption, Distribution, Metabolism, and Excretion (ADME). Physicochemical factors play a rather prominent role in the transfer of drugs across cell membranes (absorption). Mechanisms by which drugs cross membranes and the physicochemical properties of molecules and membranes that influence this transfer are critical to understanding the disposition of drugs in the target species (distribution). Once in the body, drugs are degraded (metabolism) and then any residual parent drug and metabolic derivatives are eliminated (excretion). The characteristics of a drug that predict its ADME include its molecular size and structural features, degree of ionization, relative lipid solubility of its ionized and non-ionized forms, and binding to serum and tissue proteins (Hilal-Dandan and Brunton, 2014).
In particular, lipid solubility is an important determinant of the pharmacokinetic characteristics of a drug. Many properties, such as rate of absorption from the gut, penetration into tissues, and duration of action, can be predicted from knowledge of a drug’s lipid solubility. Compounds that exhibit polar functional groups, such as hydroxyl, amino, or carboxylic acid moieties, tend to be more hydrophilic in nature. Generally, hydrophilic metabolites are excreted through the kidneys (renal), whereas more hydrophobic metabolites are excreted through the feces (liver and bile; Anders, 1980; Lohr et al., 1998). Some derivatives of the phenethanolamine core structure contain halogen substituents (e.g., clenbuterol). These halogenated derivatives also tend to increase the lipid solubility of a molecule and may result in accumulation in lipid-rich tissues (adipose tissue). For example, poly-chlorinated furans and dioxins may have very long half-lives and persist in lipid tissues. In addition, the geometrical size of a halogen atom is larger than that of an oxygen, carbon, or hydrogen moiety and may limit transport through a cell membrane.
Major reactions involved in drug metabolism are oxidative reactions, such as N-dealkylation, O-dealkylation, aliphatic and aromatic hydroxylation, N-oxidation, S-oxidation, and deamination, hydrolysis reactions, and conjugation reactions, such as glucuronidation, sulfation or sulfonation, acetylation, methylation or alkylation, and glutathionation. Molecular size and structural features help determine the metabolic pathways used and rate of metabolism for compounds, with the latter being more important (Bocci et al., 2017; Zhang and Tang, 2018). Structures that contain a more stereochemically bulky group (compared with a less stereochemically bulky group in a set of analogs, such as a tertiary butyl group vs. an isopropyl group) may be metabolized more slowly. However, the chemical nature of the group is generally a more important feature in its biotransformation than steric size (see Figure 1, salbutamol). Furthermore, β-AA having halogenated aromatic ring substituents (e.g., chlorine substituents in clenbuterol) are not easily metabolized by oxidative and conjugative pathways. In fact, clenbuterol was designed specifically to resist rapid metabolic degradation by enzymes active toward aromatic hydroxyl groups (Morgan, 1990) and have a long plasma half-life. In contrast, β-AA having hydroxylated aromatic rings are readily metabolized, typically by conjugation, and have relatively short plasma half-lives (e.g., ractopamine). Substituents that are subject to metabolism by oxidative or conjugative detoxification mechanisms may be metabolized via renal pathways depending on the substituent (see Figure 1, ractopamine). In addition, in some instances, treatments to modify the urinary pH (e.g., addition of bicarbonate salts) can influence the excretion of hydrophilic metabolites.
The affinity of a drug to bind to a receptor site is affected by several chemical properties. The stereochemistry of a substance can determine its ability to bind effectively to a given receptor site. Therefore, when compounds have different stereoisomers, these various forms may have different biological properties. For example, there are four stereoisomers for ractopamine (RR, RS, SR, and SS), but RR isomers show the greatest activity (Mills et al., 2003). Another property that may affect receptor binding is exhibited by compounds that are lipophilic and contain amino groups, which hypothetically interact with receptors that are integral membrane proteins. Lipophilic amines are also chemically attracted to negatively charged phospholipid-containing cell membranes. Consequently, receptor binding may affect the strength of the biological response. When compounds are bound to receptors, metabolism and elimination may also be hindered (see Smith, 1998, for review). Furthermore, bulky substituents can also greatly affect the ability of the molecule to bind to the receptor. This may explain the unique antagonist activity of lubabegron for the β1 and β2 receptors and agonist activity for the β3 receptor, due to the substituents’ potential effect on binding affinity.
Differentiating β-ligands used in cattle production
Currently, there are three FDA-approved β-ligands for use in the production of cattle for beef (food production): ractopamine, zilpaterol, and lubabegron. However, for comparison, information regarding the ADME of clenbuterol is also included. Clenbuterol is not approved for use in food-producing animals but, as mentioned previously, has been implicated in illegal usage in several countries. The ability to directly compare these β-ligands is limited for several reasons. First, there exist no ADME studies in which multiple β-ligands have been used and these data collected. The data that are available only make use of a single compound, as these studies are completed during the process of gaining approval from regulatory bodies for their use. Second, these data have been collected over multiple decades. During this time, both methods for determining this information and, at times, the definitions of these terms have changed. Finally, studies are not always performed in the same species. Therefore, the data in Table 1, while incomplete, represent the best knowledge available to differentiate β-ligands from one another.
. | Ractopamine1 . | Lubabegon2 . | Zilpaterol3 . | Clenbuterol4 . |
---|---|---|---|---|
Route of administration | Feed additive | Feed additive | Feed additive | Injectable |
Lipid solubility | Hydrochloride salt. Soluble in hydrophilic media such as water and some polar solvents | Hemifumarate salt. Not soluble in water. Soluble in ethanol/citric acid solution | Hydrochloride salt. Soluble in hydrophilic media such as water at pH 1 to 10. Slightly soluble in methanol but not ethanol | Hydrochloride salt. High solubility in water, ethanol, and methanol |
Absorption | Readily absorbed in the gut into plasma | Readily absorbed in the gut into plasma | Readily absorbed in the gut into plasma | Readily absorbed in the gut into plasma |
Distribution | Throughout the body to heart, lungs, liver, muscle, and adipose tissue | Throughout the body to heart, lungs, liver, muscle, and adipose tissue | Throughout the body to heart, lungs, liver, muscle, and adipose tissue | Throughout the body to heart, lungs, liver, muscle, and adipose tissue |
Biotransformation | Glucuronide conjugation with some di-glucuronide conjugation | Oxidative transformation | Conjugation pathways limited or absent. Parent, desisopropanol, and hydroxyl metabolites account for more than 90% of identifiable residues in cattle urine | Glucuronide conjugation and oxidative transformation |
Excretion | 46% excreted in urine and 52% in feces | 80% excreted in feces after oral dose in cattle | 88% in urine and 2% to 3% in feces in cattle | 50% to 85% in urine and 5% to 30% in feces after oral, intramuscular, or intravenous dose in cattle |
. | Ractopamine1 . | Lubabegon2 . | Zilpaterol3 . | Clenbuterol4 . |
---|---|---|---|---|
Route of administration | Feed additive | Feed additive | Feed additive | Injectable |
Lipid solubility | Hydrochloride salt. Soluble in hydrophilic media such as water and some polar solvents | Hemifumarate salt. Not soluble in water. Soluble in ethanol/citric acid solution | Hydrochloride salt. Soluble in hydrophilic media such as water at pH 1 to 10. Slightly soluble in methanol but not ethanol | Hydrochloride salt. High solubility in water, ethanol, and methanol |
Absorption | Readily absorbed in the gut into plasma | Readily absorbed in the gut into plasma | Readily absorbed in the gut into plasma | Readily absorbed in the gut into plasma |
Distribution | Throughout the body to heart, lungs, liver, muscle, and adipose tissue | Throughout the body to heart, lungs, liver, muscle, and adipose tissue | Throughout the body to heart, lungs, liver, muscle, and adipose tissue | Throughout the body to heart, lungs, liver, muscle, and adipose tissue |
Biotransformation | Glucuronide conjugation with some di-glucuronide conjugation | Oxidative transformation | Conjugation pathways limited or absent. Parent, desisopropanol, and hydroxyl metabolites account for more than 90% of identifiable residues in cattle urine | Glucuronide conjugation and oxidative transformation |
Excretion | 46% excreted in urine and 52% in feces | 80% excreted in feces after oral dose in cattle | 88% in urine and 2% to 3% in feces in cattle | 50% to 85% in urine and 5% to 30% in feces after oral, intramuscular, or intravenous dose in cattle |
. | Ractopamine1 . | Lubabegon2 . | Zilpaterol3 . | Clenbuterol4 . |
---|---|---|---|---|
Route of administration | Feed additive | Feed additive | Feed additive | Injectable |
Lipid solubility | Hydrochloride salt. Soluble in hydrophilic media such as water and some polar solvents | Hemifumarate salt. Not soluble in water. Soluble in ethanol/citric acid solution | Hydrochloride salt. Soluble in hydrophilic media such as water at pH 1 to 10. Slightly soluble in methanol but not ethanol | Hydrochloride salt. High solubility in water, ethanol, and methanol |
Absorption | Readily absorbed in the gut into plasma | Readily absorbed in the gut into plasma | Readily absorbed in the gut into plasma | Readily absorbed in the gut into plasma |
Distribution | Throughout the body to heart, lungs, liver, muscle, and adipose tissue | Throughout the body to heart, lungs, liver, muscle, and adipose tissue | Throughout the body to heart, lungs, liver, muscle, and adipose tissue | Throughout the body to heart, lungs, liver, muscle, and adipose tissue |
Biotransformation | Glucuronide conjugation with some di-glucuronide conjugation | Oxidative transformation | Conjugation pathways limited or absent. Parent, desisopropanol, and hydroxyl metabolites account for more than 90% of identifiable residues in cattle urine | Glucuronide conjugation and oxidative transformation |
Excretion | 46% excreted in urine and 52% in feces | 80% excreted in feces after oral dose in cattle | 88% in urine and 2% to 3% in feces in cattle | 50% to 85% in urine and 5% to 30% in feces after oral, intramuscular, or intravenous dose in cattle |
. | Ractopamine1 . | Lubabegon2 . | Zilpaterol3 . | Clenbuterol4 . |
---|---|---|---|---|
Route of administration | Feed additive | Feed additive | Feed additive | Injectable |
Lipid solubility | Hydrochloride salt. Soluble in hydrophilic media such as water and some polar solvents | Hemifumarate salt. Not soluble in water. Soluble in ethanol/citric acid solution | Hydrochloride salt. Soluble in hydrophilic media such as water at pH 1 to 10. Slightly soluble in methanol but not ethanol | Hydrochloride salt. High solubility in water, ethanol, and methanol |
Absorption | Readily absorbed in the gut into plasma | Readily absorbed in the gut into plasma | Readily absorbed in the gut into plasma | Readily absorbed in the gut into plasma |
Distribution | Throughout the body to heart, lungs, liver, muscle, and adipose tissue | Throughout the body to heart, lungs, liver, muscle, and adipose tissue | Throughout the body to heart, lungs, liver, muscle, and adipose tissue | Throughout the body to heart, lungs, liver, muscle, and adipose tissue |
Biotransformation | Glucuronide conjugation with some di-glucuronide conjugation | Oxidative transformation | Conjugation pathways limited or absent. Parent, desisopropanol, and hydroxyl metabolites account for more than 90% of identifiable residues in cattle urine | Glucuronide conjugation and oxidative transformation |
Excretion | 46% excreted in urine and 52% in feces | 80% excreted in feces after oral dose in cattle | 88% in urine and 2% to 3% in feces in cattle | 50% to 85% in urine and 5% to 30% in feces after oral, intramuscular, or intravenous dose in cattle |
In general, β-ligands are absorbed readily by the gut into the plasma with peak plasma concentrations occurring 1 to 3 h after oral administration. Beta-ligands are then distributed through the plasma to various tissues, such as muscle, adipose tissue, liver, kidney, brain, and lungs. These compounds are metabolized both in the intestine and in the liver.
Ractopamine is administered as a feed additive in the form of a hydrochloride salt. It is soluble in water and some polar solvents. It is readily absorbed and distributed in the plasma to various organs. It binds to and activates β1 and β2 receptors found throughout the body including in the muscle and adipose tissue. Ractopamine is metabolized by glucuronide conjugation with some di-glucuronide conjugation mainly in the liver (Figure 2). Ractopamine fed to cattle is excreted in the feces and urine (FDA, 2003).
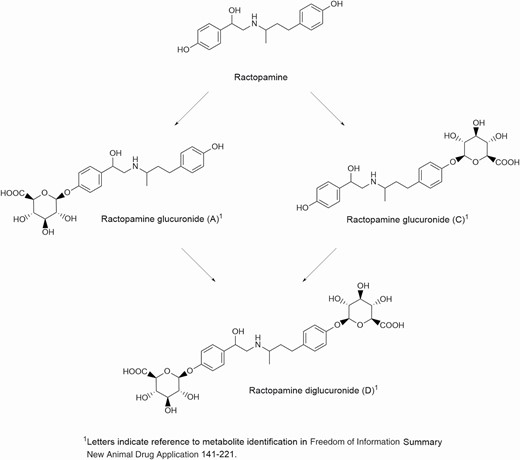
Zilpaterol is administered as a feed additive in the form of a hydrochloride salt. It is soluble in water and slightly soluble in methanol but not ethanol. It is readily absorbed in the gut and distributed in the plasma to various organs. It binds to and activates β1 and β2 receptors found throughout the body including in the muscle and adipose tissue. Unlike other β-ligands, zilpaterol does not undergo extensive conjugation (Figure 3); the parent, desisopropyl, and hydroxyl metabolites account for over 90% of identifiable residues in cattle urine. Metabolism occurs in the liver and those metabolites are rapidly excreted in the urine and feces (JECFA, 2014, 2016).
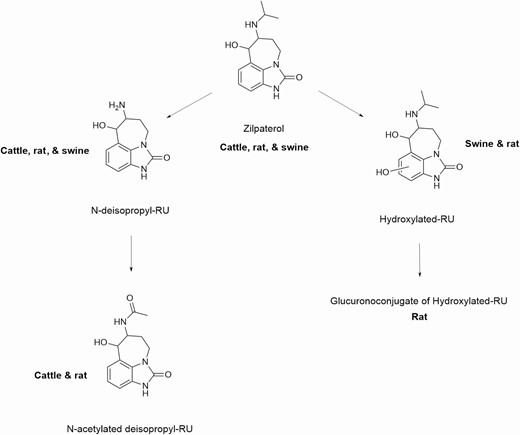
Lubabegron is administered as a feed additive in the form of a hemifumarate salt and is not soluble in water. It is readily absorbed in the gut and distributed in the plasma to various organs. It binds to and activates β3 receptors found throughout the body including in the muscle and adipose tissue but acts as an antagonist to β1 and β2 receptors. Lubabegron is metabolized by oxidative transformation (Figure 4) in the liver and excreted not only mainly in the feces but also in urine (FDA, 2018).
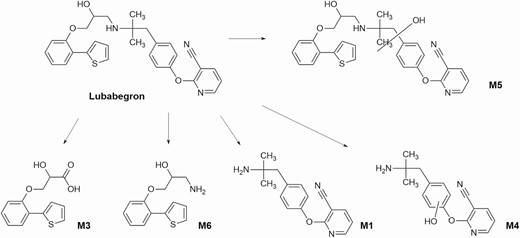
Clenbuterol is administered as a hydrochloride salt and is soluble in water, ethanol, and methanol. Clenbuterol is readily absorbed in the gut and distributed in the plasma to various organs. It binds to and activates β2 receptors in the muscle, adipose tissue, and heart (along with other organs). Clenbuterol is metabolized mainly in the liver (Figure 5) by oxidative transformation and to a lesser extent, glucuronide conjugation. It is excreted as metabolites not only mainly in urine but also in feces (EMEA, 2000).
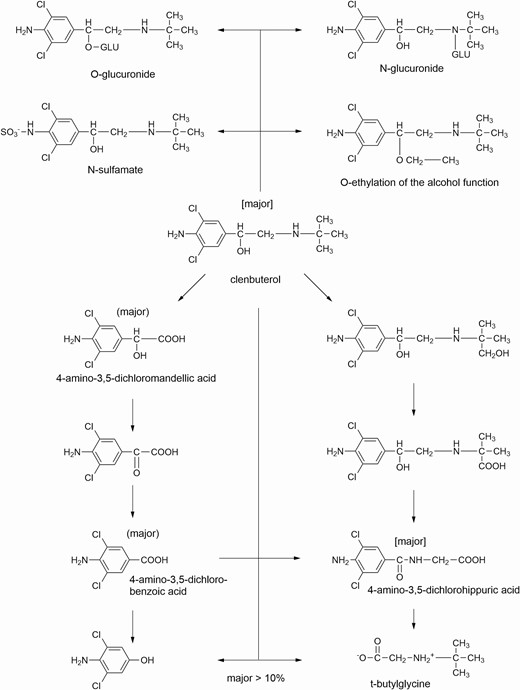
Comparison of Mode of Action of β-ligands Used in Cattle Production
As mentioned previously, the β-AA are currently used in both human and veterinary medicine and, in accord with good veterinary practice (GVP) for the purpose of performance enhancement, have certain characteristics that can cause potentially unwanted side effects. Historically, β-ligands for use in animal agriculture have been β-AA that act on β1- and β2-AR, which have the potential for adverse effects in humans if not used properly. Beta-ligands also demonstrate lipolytic activity and cause downregulation of the receptors after prolonged agonist activity (Smith, 1998). As alluded to previously, the mode of action and pharmacokinetics of lubabegron differ from the existing β-AA. Lubabegron (Figure 1) belongs to a novel class of SβM intended for use in animal food production to decrease ammonia gas emissions per unit of live or carcass weight in beef cattle.
With regard to the pharmacology of lubabegron, in vitro experiments were performed to assess its interaction with multiple receptor systems in humans and comparative animal models (FDA, 2018). Collectively, results indicate that lubabegron is different from classically defined β1 and β2-agonists, e.g., ractopamine and zilpaterol, which bind to and produce, primarily, an agonist response at the β1- and β2-AR (Moody et al., 2000, 2005; Verhoeckx et al., 2005). Instead, lubabegron is a β3-AR agonist that also exhibits a high affinity for the β1- and β2-AR but with antagonistic activity.
The antagonist activity of lubabegron at β1 and β2 receptors prevents the stimulation of the β-AR found in the heart (β1) and trachea/bronchi (β2) of humans and, in doing so, avoids the potential negative side effects associated with β1 and β2 receptor activation. Experiments in human subjects demonstrated β1-antagonism. A higher dose of isoproterenol was required to increase heart rate when subjects were administered lubabegron (FDA, 2018). While ractopamine and zilpaterol result in increased heart rate at differing concentrations following oral treatment, heart rate in lubabegron-treated animals decreased. This indicated that, among other factors, lubabegron does not activate the β1- or β2-AR with any degree of significance. It is unclear what effect there might be of β2 receptor antagonism by lubabegron on the heart rate of cattle.
In relation to lipolytic activity, studies conducted in Holstein steers demonstrated no increase in non-esterified fatty acid levels despite increases recorded from cattle administered ractopamine (Figure 6). These results suggest that lubabegron lacks lipolytic activity. The lack of lipolytic effect could be due to lower expression of β 3-AR agonist activity of lubabegron in bovine adipose tissue and higher expression of β1-AR and β2-AR antagonistic activity in this tissue (Sillence and Matthews, 1994; Van Liefde et al., 1994). Under this scenario, the β1-AR and β2-AR antagonist behavior of lubabegron could decrease lipolysis in adipose tissue, whereas the β3-AR agonist activity could increase skeletal muscle hypertrophy, possibly due to the differences in the second messenger systems and enzyme expression in skeletal muscle compared with adipose tissues. All three receptor subtypes activate cAMP through Gs protein (Moody et al., 2005), suggesting that differences in receptor activation effects may be due to differences in downstream pathways present in various cell types.
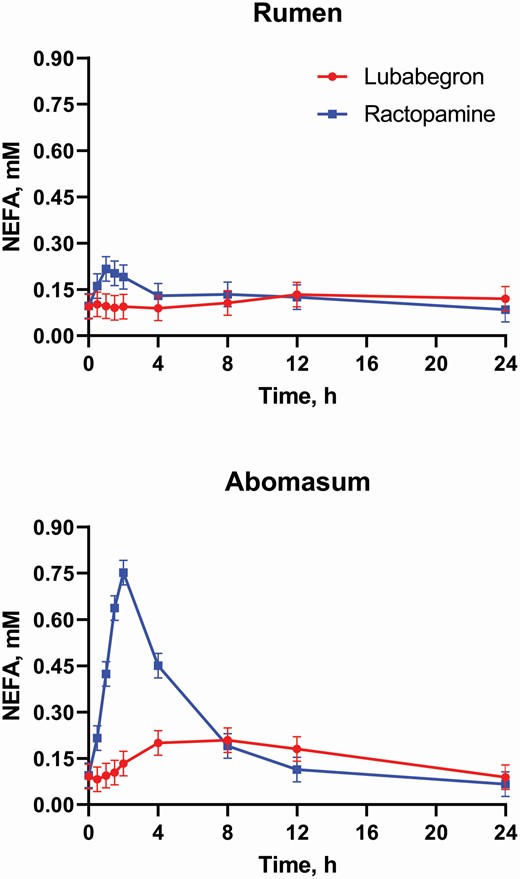
Twelve Holstein steers (200 to 250 kg BW) were fitted with permanent rumen fistulae and adapted to an 80% concentrate diet were utilized. Treatments consisted of 0.5 mg/kg lubabegron or 2.0 mg/kg ractopamine each alternately administered ruminally or post-ruminally, in a replicated (n = 3) 4 × 4 Latin Square with a 2 × 2 factorial arrangement. Blood samples were collected via jugular catheter 30 min prior to dosage and at 0.5, 1, 1.5, 2, 4, 8, 12, and 24 h post dosage. Plasma was harvested and then stored at −80 °C until assayed for nonesterified fatty acids (NEFA). Ractopamine and lubabegron doses in this study represent approximately two-times the maximum approved labeled dose for each compound.
There are numerous examples in the literature demonstrating that the long-term use of β-AA results in decreased effectiveness due to downregulation and desensitization of the β-AR. This process is thought to consist of three main mechanisms (Lohse et al., 1990). The first mechanism is the phosphorylation of receptors through cAMP-dependent protein kinases (Ferguson, 2001; Coman et al., 2009). The second mechanism is the phosphorylation of specific agonist-dependent β-AR kinases (Lohse et al., 1990). The third mechanism is the intracellular sequestration of the ligand-receptor complex (i.e., movement of the receptor away from the cell surface; Lohse et al., 1990; Coman et al., 2009). Two normal functions of the β-AR are lost once the β-AR experiences intracellular sequestration. First, the β-AA, being hydrophilic in nature, can no longer bind to the β-AR because the cell membrane acts as a barrier. Second, the β-AR is uncoupled from the Gs protein and no longer stimulates adenylyl cyclase activity (Waldo et al., 1983). Therefore, even if β-AA are present, when receptors are sequestered inside the cell, no downstream effects of β-AA occur. In contrast to β-AA, β-blockers do not result in the phosphorylation of receptors from activation of β-AR signaling cascades. Thus, their binding to receptors does not result in receptor sequestration.
The β3-AR is unique compared with β1- and β2-AR because the β3-AR does not appear to downregulate after long-term activation (Carpéné et al., 1993; Nantel et al., 1993, 1994; Mersmann, 1998). This lack of β3-AR downregulation appears to be because the receptor does not internalize within the cell upon binding, in contrast with the mechanism of β1-AR and β2-AR (Nantel et al., 1993). Therefore, as a β3-AR agonist, lubabegron does not produce desensitization. The approval of lubabegron for extended us (91d) also suggests the lack of receptor desensitization.
International Standards for β-Ligands
Risk assessment introduction
Risk assessment for residues of veterinary drugs at the national and international levels has been ongoing for more than 30 yr, and it continues to evolve as new science permits. Risk assessment is a rigorous process that consistently applies a set of scientifically guided principles to evaluate the safety of veterinary drugs in food-producing animals to provide assurance of their safety for consumers (FAO/WHO, 2009). This risk assessment relies on these veterinary drugs being used according to conditions established by regulatory authorities and in accordance with GVP. In general, GVP includes the use of veterinary drugs in accordance with label directions; however, there is no official internationally agreed definition of GVP. The World Organisation for Animal Health and the World Veterinary Association have agreed to abide by a Code of Practice whereby practitioners take an oath to protect the health and well-being of all animals. In addition, different regions of the world have developed their own guidelines. An example appears on the Federation of Veterinarians of Europe website (http://www.fve.org/uploads/publications/docs/gvp.pdf).
In a broader context, risk assessment is the scientific component of risk analysis that regulatory authorities apply to carry out their public health responsibilities for safe foods.
Definitions of risk analysis and risk assessment are usually available in publications from national authorities that have responsibilities for food safety. Consequently, there may be several different definitions of risk assessment and analysis developed by individual national regulatory authorities in accordance with their own laws and regulations. Nonetheless, regulatory authorities develop their definitions and procedures with a strict focus on the safety and efficacy of products for use in food-producing animals. As such, it would be difficult to present risk assessment standards in a universally applicable and comprehensive manner. Due to the significant international trade in foods of animal origin, it makes sense to describe the definitions agreed to by the Codex Alimentarius Commission (CAC), which is an intergovernmental body and not a regulatory entity. These definitions are agreed upon by the 190 member states of Codex. The Codex Procedural Manual (WHO, 2013) provides a set of definitions on risk analysis. For this discussion, four terms are provided for introductory guidance:
• Hazard: A biological, chemical, or physical agent in, or condition of, food with the potential to cause an adverse health effect.
• Risk: A function of the probability of an adverse effect and the severity of the effect, consequential to a hazard(s) in food.
• Risk analysis: A process consisting of three components—risk assessment, risk management, and risk communication.
• Risk assessment: A scientifically based process consisting of the following steps: 1) hazard identification, 2) hazard characterization, 3) exposure assessment, and 4) risk characterization.
Risk assessment for veterinary drugs in foods
A quantitative risk assessment for residues of veterinary drugs in foods includes a hazard assessment (the toxicological assessment). An inspection of the available literature reveals that there is a large amount of individual data on parameters such as plasma half-lives, estimates of drug availability, biotransformation and excretion pathways, and receptor-binding selectivity from various sources as summarized in Tables 1–3 and the comprehensive review by Smith (1998). The data reported in the bulk of the open literature on these β-ligands are primarily from their potential use in human medicine applications, such as treatment for chronic obstructive pulmonary diseases. While application for potential use in human medicine is beyond the scope of this report, these data aid our understanding of the risk of inadvertent acute exposure of the consumer to drug residues in food. A toxicological assessment of a compound results in the determination of the amount of compound that exhibits an adverse effect in a test species. This can be established by studies in humans or extrapolated from animal studies. This level is termed the “no observed adverse effect limit” (NOAEL) or a “no observed effect level” (NOEL). Safety factors are applied to this NOAEL to establish an acceptable daily intake (ADI), defined as the amount of a compound a person could consume each day for a lifetime with no adverse health effects.
Compound . | NOEL/NOAEL . | NOEL level, µg/kg BW . | Observed (adverse) effect in humans . | ADI, µg/kg BW . |
---|---|---|---|---|
Clenbuterol1 | NOEL | 0.04 | Bronchodilation | 0 to 0.004 |
Ractopamine2 | NOEL | 67 | Acute cardiac response | 0 to 1.25 |
Zilpaterol3 | LOAEL | 0.76 | Tremors | 0 to 0.04 |
Lubabegron4 | NOEL | 160 | Decreased blood pressure and heart rate | 0 to 3 |
Compound . | NOEL/NOAEL . | NOEL level, µg/kg BW . | Observed (adverse) effect in humans . | ADI, µg/kg BW . |
---|---|---|---|---|
Clenbuterol1 | NOEL | 0.04 | Bronchodilation | 0 to 0.004 |
Ractopamine2 | NOEL | 67 | Acute cardiac response | 0 to 1.25 |
Zilpaterol3 | LOAEL | 0.76 | Tremors | 0 to 0.04 |
Lubabegron4 | NOEL | 160 | Decreased blood pressure and heart rate | 0 to 3 |
1Clenbuterol is not approved for use in food-producing animals in the United States; therefore, no ADI has been determined by the FDA. In the European Union (Directive EU 96/22), clenbuterol is approved for tocolysis in parturient cows. Data are from EMEA (2000).
Compound . | NOEL/NOAEL . | NOEL level, µg/kg BW . | Observed (adverse) effect in humans . | ADI, µg/kg BW . |
---|---|---|---|---|
Clenbuterol1 | NOEL | 0.04 | Bronchodilation | 0 to 0.004 |
Ractopamine2 | NOEL | 67 | Acute cardiac response | 0 to 1.25 |
Zilpaterol3 | LOAEL | 0.76 | Tremors | 0 to 0.04 |
Lubabegron4 | NOEL | 160 | Decreased blood pressure and heart rate | 0 to 3 |
Compound . | NOEL/NOAEL . | NOEL level, µg/kg BW . | Observed (adverse) effect in humans . | ADI, µg/kg BW . |
---|---|---|---|---|
Clenbuterol1 | NOEL | 0.04 | Bronchodilation | 0 to 0.004 |
Ractopamine2 | NOEL | 67 | Acute cardiac response | 0 to 1.25 |
Zilpaterol3 | LOAEL | 0.76 | Tremors | 0 to 0.04 |
Lubabegron4 | NOEL | 160 | Decreased blood pressure and heart rate | 0 to 3 |
1Clenbuterol is not approved for use in food-producing animals in the United States; therefore, no ADI has been determined by the FDA. In the European Union (Directive EU 96/22), clenbuterol is approved for tocolysis in parturient cows. Data are from EMEA (2000).
Compound . | MRL, µg/kg . | . | . | . | . | Withholding time . |
---|---|---|---|---|---|---|
. | Muscle . | Liver . | Kidney . | Fat . | Milk . | . |
Clenbuterol1 | 0.2 | 0.5 | 0.5 | — | 0.05 | — |
Ractopamine2 | 10 | 40 | 90 | 10 | NA3 | 0 d (<12 h) |
Zilpaterol4 | 0.5 | 3.5 | 3.3 | — | NA | 2 to 4 d5 (77 h4) |
Lubabegron6 | 2 | 7 | 7 | 2 | NA | 0 d (<12 h)7 |
Compound . | MRL, µg/kg . | . | . | . | . | Withholding time . |
---|---|---|---|---|---|---|
. | Muscle . | Liver . | Kidney . | Fat . | Milk . | . |
Clenbuterol1 | 0.2 | 0.5 | 0.5 | — | 0.05 | — |
Ractopamine2 | 10 | 40 | 90 | 10 | NA3 | 0 d (<12 h) |
Zilpaterol4 | 0.5 | 3.5 | 3.3 | — | NA | 2 to 4 d5 (77 h4) |
Lubabegron6 | 2 | 7 | 7 | 2 | NA | 0 d (<12 h)7 |
1Clenbuterol is not approved for use in food-producing animals in the United States. In the European Union (Directive EU 96/22), clenbuterol is approved for tocolysis in parturient cows. In 2000, MRL were established by The European Agency for the Evaluations of Medicinal Products, Veterinary Medicines, and Information Technology Unit (EMEA, 2000).
2MRL as determined by Canadian Veterinary Drugs Directorate on a free base basis (http://www.hc-sc.gc.ca/dhp-mps/vet/mrl-lmr/2013-2-prop-eng.php).
3NA, not applicable.
4MRL were proposed in JECFA (2014) but have not been adopted.
6MRL as determined by Canadian Veterinary Drugs Directorate.
Compound . | MRL, µg/kg . | . | . | . | . | Withholding time . |
---|---|---|---|---|---|---|
. | Muscle . | Liver . | Kidney . | Fat . | Milk . | . |
Clenbuterol1 | 0.2 | 0.5 | 0.5 | — | 0.05 | — |
Ractopamine2 | 10 | 40 | 90 | 10 | NA3 | 0 d (<12 h) |
Zilpaterol4 | 0.5 | 3.5 | 3.3 | — | NA | 2 to 4 d5 (77 h4) |
Lubabegron6 | 2 | 7 | 7 | 2 | NA | 0 d (<12 h)7 |
Compound . | MRL, µg/kg . | . | . | . | . | Withholding time . |
---|---|---|---|---|---|---|
. | Muscle . | Liver . | Kidney . | Fat . | Milk . | . |
Clenbuterol1 | 0.2 | 0.5 | 0.5 | — | 0.05 | — |
Ractopamine2 | 10 | 40 | 90 | 10 | NA3 | 0 d (<12 h) |
Zilpaterol4 | 0.5 | 3.5 | 3.3 | — | NA | 2 to 4 d5 (77 h4) |
Lubabegron6 | 2 | 7 | 7 | 2 | NA | 0 d (<12 h)7 |
1Clenbuterol is not approved for use in food-producing animals in the United States. In the European Union (Directive EU 96/22), clenbuterol is approved for tocolysis in parturient cows. In 2000, MRL were established by The European Agency for the Evaluations of Medicinal Products, Veterinary Medicines, and Information Technology Unit (EMEA, 2000).
2MRL as determined by Canadian Veterinary Drugs Directorate on a free base basis (http://www.hc-sc.gc.ca/dhp-mps/vet/mrl-lmr/2013-2-prop-eng.php).
3NA, not applicable.
4MRL were proposed in JECFA (2014) but have not been adopted.
6MRL as determined by Canadian Veterinary Drugs Directorate.
After hazard assessment, an evaluation for exposure must be completed. People are exposed to residues of compounds through the consumption of animal products, but not all products are consumed in the same amounts. For example, when people consume whole muscle products (steaks and roasts), serving sizes are usually larger than when people consume organ or variety meats (kidneys and livers). As a consequence, the amount of residue that is allowable in a particular animal tissue to comply with the ADI will vary based, in part, on the consumption factor of that tissue. The report of the Expert FAO/WHO Committee on Exposure Assessment held in conjunction with the 75th Joint FAO/WHO Expert Committee on Food Additives (JECFA) meeting in November 2011 makes this abundantly clear, and readers are encouraged to review that report for details as it is beyond the scope of this paper.
Management of the risk involves recommending maximum residue limits (MRL) for residues in food animal tissues to provide assurance of compliance with the health-based guidance value (typically, the ADI). This requires an assessment of residue exposure, including factors such as residue metabolic profiles, drug residues of toxicological significance in food animal tissue, residue data determined from food animal studies using recommended doses administered to animals in accord with labeling guidelines, pharmacokinetics, and identification of a marker residue compound upon which the recommended MRL is based. On some occasions, residues that are demonstrated to have no toxicological significance, such as those from substances with a microbiological-based ADI (e.g., those residues with no microbiological activity against the test organisms), may be discounted.
An ideal marker residue is a selected compound that typically has a well-defined and stable relationship to the total residues of toxicological concern. The residue marker is usually determined using radiolabel drug in control studies. The marker residue may be either the parent drug or a stable metabolite identified in the tissues of interest. The same marker residue is used for the assessment of all food animal tissues. Marker residue relationships to total residues of toxicological concern have a ratio of less than 1 (e.g, 0.1 to 0.9). When calculating the residue exposure in individual tissues, the measured residue exposure in each tissue is corrected to total residues of toxicological concern using the marker residue concentrations in the individual tissues. Exposure is calculated in each tissue separately and the summation of residue exposures from all tissues is compared with the health-based guidance value.
International standards for β-ligand residues in food animal tissues
The assessment of ADME, as well as the chemical and physical properties of the individual substances, broadens the understanding of the factors that influence a risk assessment and are critical to the outcomes of the risk assessment. Substances for consideration by JECFA are based on recommendations of the Codex Committee on Residues of Veterinary Drugs in Food (CCRVDF). Criteria have been developed by that committee for consideration, including that the product is registered for veterinary use by a national authority, is relevant in international trade, and is safe and effective veterinary drug and that all relevant studies will be made available in a timely manner. On occasions, because of a lack of available contemporary scientific studies for submission to JECFA, including appropriate ADME or a lack of safety and efficacy studies in food-producing animals, not all CCRVDF recommended substances are either partially or fully evaluated (e.g., only an ADI but no recommended MRL is issued). Reasons may vary, such as the submitted data do not meet current regulatory standards, newer studies determine that the substance is no longer considered safe for use in food-producing animals (e.g., new studies determine potential genotoxicity), or that a sponsor is unable or unwilling to provide the necessary studies. The number of substances for which current international risk assessments for use in veterinary medicine have been completed is quite limited. Despite 30 yr of risk assessment for veterinary medicines, only about 100 veterinary medicines have met the data requirements for recommendation and adoption as standards by Codex Standards and the CAC. The outcomes of the CAC do not affect the decisions of individual national regulatory authorities regarding a particular veterinary drug.
To date, among β-ligands, only clenbuterol, ractopamine, and zilpaterol have been considered by Codex, but standards have been established for only two compounds—clenbuterol and ractopamine (Table 3). The MRL (Table 3) for zilpaterol were recommended by JECFA in 2016, but the CCRVDF has not formally agreed to recommend its adoption by the CAC. For those wishing to further review the Codex standards for these veterinary drugs, Codex uses the terminology “β2-adrenoceptor agonist” for these compounds.
Perhaps as a consequence of failing to recognize the differences among various β-AA, scientific and national political risk-management decisions have led to highly polarized positions when trying to adopt global standards. JECFA has conducted risk assessments for these compounds and then undertaken subsequent assessments that reconfirm initial findings that there are no safety issues when approved compounds are used as labels direct. Even so, groups debate and do not accept these findings during efforts by the CAC to adopt international standards. In some instances, Codex standards have only been agreed upon with very specific provisions, such as tocolysis in cattle and bronchodilating treatments in horses. Disagreements between countries regarding the safety of β-AA that focus on the outcomes of the risk assessments occur, often irrespective of understanding the differences among the β-AA. Countries that approve the use of these molecules rely on and support the risk assessment outcomes. Those that do not permit growth-promoting agents in food animal production are doing so based on national legislation and not necessarily based on scientific outcomes. Debate about these issues has been, and is expected to continue to be, highly contentious. However, it is vital to recognize the differences between various β-ligands, including β-AA and more recently developed SβM like lubabegron, as differences in their risk assessments may provide scientific justification for having different policies pertaining to their use.
Risk assessment for lubabegron and comparison to other β-ligands
In the FDA-required studies, lubabegron demonstrated substantial pharmacologic toxicological differences compared with ractopamine and zilpaterol. The critical factor for determining the NOEL used by FDA to establish an ADI was higher in dose concentration for lubabegron compared with the other β-ligands (Table 2). In data from human trials, NOEL of lubabegron (FDA, 2018) was 160 µg/kg body weight (BW), whereas the NOEL for ractopamine was 67 µg/kg BW (FDA, 2003). The lowest observed adverse effect level (LOAEL) of zilpaterol was 0.76 µg/kg BW (JECFA, 2014). The NOEL for clenbuterol is even lower at 0.04 µg/kg BW (EMEA, 2000). After the most relevant study is identified (usually the study with the lowest no effect level), a safety factor is applied in order to calculate the ADI and reported as a range from zero to a finite number using only one significant number (e.g., 0 to 0.05 mg/kg BW).
The ADI (Table 2) for β-ligands also differ greatly, reflective of their different NOEL. Clenbuterol, with the lowest NOEL, has the lowest ADI at 0.004 µg/kg BW, 10× lower than that of zilpaterol (0.04 µg/kg BW). In contrast, the ADI for ractopamine is 1 µg/kg BW per day, and the ADI for lubabegron is 3 µg/ kg BW per day. A higher ADI is generally indicative of lower absolute toxicological effects. Given this relatively larger ADI in comparison to other β-ligands, lubabegron could, in fact, be inherently considered safer than ractopamine and zilpaterol. Consideration of recommended MRL is less straightforward, as consideration of the relationship of the marker residue to total residues of toxicological concern may greatly influence the recommended MRL. Substances that have a relatively high ratio of marker residue to total residue (e.g., 0.6) would result in relatively higher MRL recommendations than the same substance where the marker residue to total residues is relatively low (e.g., 0.06).
Considerations when using MRL in risk management
While the MRL of a tissue establishes the level of compound in a particular tissue to allow for compliance with the ADI limit, it is not without complications. First, MRL are established with a particular testing procedure. In the approval process of the FDA, new animal drugs are registered along with a protocol for testing of residues. These protocols specify the methods used to collect tissues, proper storage of tissues prior to testing, testing methods, limits of detection, and test sensitivity. Deviations from these procedures can result in variable results.
MRL are established for a particular tissue based on two factors. First, the ADI is determined, which is a function of the compound and not the tissue. The ADI does not differ between tissues because it is established for the compound itself. Second, MRL are established based on how much of a particular tissue (food) is consumed by people; this is termed a consumption factor. Tissues that are not consumed as food (e.g., bones, hooves, and urine) do not have MRL established for them, as there is no consumption factor for these items. Therefore, detection of compound residues in these tissues is not an indication of proper or improper use of compounds. Detection of a certain level of compound in a tissue without an established MRL cannot be used as an indication of a “violative residue” simply because there is no standard to violate. Along the same lines, MRL are not interchangeable between tissues. Due to differences in consumption factors and residue concentrations in various tissues, MRL differ between tissues (Table 3). For example, muscle typically has lower MRL than the liver. If a tissue without an MRL is tested for residues, applying the MRL from another tissue is not appropriate for two reasons. First, consumption factors may differ between those tissues, leading to differences in the exposure of individuals consuming those tissues. Second, both the rate and type of metabolism may differ between tissues, resulting in not only different concentrations of residues but also different marker:total ratios between tissues. Therefore, MRL are tissue-specific. Historically, the tissues of muscle, fat, liver, and kidney have been considered representative tissues, and, when residues in these tissues are within established safe tolerances, all tissues are considered safe for human consumption.
In recent years, the establishment of MRL for the global community has brought to light the vast differences in consumption of animal tissues between countries and cultures and the limited flexibility to consider other animal tissues using the “muscle, liver, kidney, and fat” food animal model diet. Animal products typically consumed in Western cultures are not consumed at the same rates in Eastern cultures, and vice versa. Therefore, prior to 2011 (and the 75th JECFA meeting), almost all regulatory authorities, including Codex, did not include the lung and other offal tissue in exposure estimates. Newer models for estimating food exposure from a larger number of potential edible tissues (e.g., heart and intestine) have continued to evolve. The database, hosted by WHO, currently contains summary statistics of 37 surveys from 26 countries (only surveys with a survey duration of 2 d or more). The consumption database was initially developed to be used by FAO/WHO scientific committees in particular for dietary exposure assessment and provides summary statistics at three levels of food categorization for a total of about 500 items at level 3. Chronic Individual Food Consumption Database—summary statistics (CIFOCOss) will from now on be continuously implemented with data from additional surveys conducted by FAO/WHO (CIFOCOss, n.d.).
One excellent example of this cultural difference in consumption regards the lungs. In the United States and many European countries, lungs are not considered food and are not processed or sold as such. Lung soup, however, is consumed by some individuals in China. Reports indicate that the appropriate consumption factor for lung soup might be as high as 300 g/d (JECFA, 2010). This is not likely 300 g of lung tissue consumption per day and instead includes broth, vegetables, and other items in these soups. Even so, lungs are particularly prone to residues of parent compound of β-ligands due to the increased prevalence of β2-AR in lungs. When compounds bind strongly to these receptors, they are protected from metabolism. Therefore, residues in the lung may be virtually 100% parent compound. For risk assessment of the use of β-ligands in cattle production, however, it should be noted that pig lungs are consumed as food in Asian countries such as China, but cattle lungs are consumed much less frequently. Furthermore, it is unclear how the unique pharmacological properties of lubabegron (a β-blocker for β2-AR) would alter the residues of lubabegron in lung tissue compared with ractopamine or zilpaterol, which are β-agonists for β2-AR. To date, there are no established MRL for any veterinary drugs including the β-ligands in lung tissue.
Biological Response/Effects of Safe Use in Accordance with Regulatory Approval and GVPs
Facing excessively fat carcasses in the early 1980s, β-AA were investigated as a method to partition nutrients away from fat and toward muscle accretion (Ricks et al., 1984). Early compounds such as clenbuterol improved lean tissue deposition at the expense of fat but were never approved in meat-producing animals due to detrimental effects on meat tenderness and concerns regarding their potential toxicity in humans due to the persistent residues in meat (Dikeman, 2007). There are currently three β-AA approved in various parts of the world. Ractopamine is approved for use in pigs, turkeys, and cattle, whereas zilpaterol and lubabegron are approved only for use in cattle. Therefore, the discussion of the effects of these β-AA will be restricted to their effects in cattle. As previously discussed, all β-ligands share some structural features and signaling pathways. Therefore, the effects of their use in animals also share some common similarities. However, their individual differences in structure, and therefore function, also result in differences in their effects in livestock.
Ractopamine
Ractopamine is approved by the FDA for use in cattle to increase the rate of weight gain, improve feed efficiency, and increase carcass leanness in animals fed in confinement for slaughter for the last 28 to 42 d on feed. According to the approval by the FDA, ractopamine has a 0-d withdrawal before the slaughter of cattle (FDA, 2018). A comprehensive meta-analysis evaluating the effects of ractopamine supplementation in feedlot cattle (Lean et al., 2014) included 31 peer-reviewed articles, theses, and proceedings, which accounted for 68 total trials. Lean et al. (2014) reported a 6.5 kg increase in final BW for ractopamine-supplemented cattle in the 44 trials that included ractopamine. This improvement in BW occurred without any significant effect on dry matter intake (DMI), as differences in DMI were calculated to be effectively zero (0.003 kg/d) between control and ractopamine-supplemented cattle. Therefore, it seems as though improvements in BW gain occur independently of intake mechanisms, which consistently results in improved feed efficiency (Lean et al., 2014).
In addition to improvements in live weight gain and feed efficiency, feeding ractopamine improves lean tissue accretion as observed through improved carcass weights. In analyzing the 54 trials that included hot carcass weight (HCW), Lean et al. (2014) reported a weighted mean improvement of 6.2 kg when cattle were supplemented with ractopamine. Of those 54 comparisons, only four reported a reduction in HCW with ractopamine feeding. Increased HCW results from increased muscle deposition. One indication of this effect is greater loin muscle area (LMA) in ractopamine-fed cattle, though results are conflicting on this point. While Scramlin et al. (2010) reported similar LMA between ractopamine-supplemented cattle and controls, others have shown significant improvements. Gruber et al. (2007) reported a 2.3-cm2 increase in LMA when feedlot cattle received 200 mg/(head · d) ractopamine for the final 28 d, and a collection of studies reported similar improvements of 1.7 to 8.9 cm2 in the LMA of calf-fed Holsteins (Vogel et al., 2009). Often used as a measure of increased muscularity, LMA is not always a clear indicator of improved carcass yield, as evidenced by Scramlin et al. (2010). Howard et al. (2014) demonstrated this in a study evaluating the lean meat yield of carcasses from ractopamine-supplemented cattle [300 or 400 mg/(head · d)]. Compared with controls, ractopamine supplemented-cattle had improved saleable yield of whole-muscle cuts by 0.61% and 0.86% for steers supplemented 300 or 400 mg/(head · d), respectively. Of the weight added to total whole-muscle saleable yield as a result of ractopamine supplementation, nearly half was in the round (Howard et al., 2014). Regardless of the location of this lean tissue accretion, it is well established that ractopamine supplementation improves HCW with little to no influence on carcass fat (adjusted rib fat thickness, kidney, heart, and pelvic fat percentage, or marbling score) of beef carcasses (Gruber et al., 2007; Scramlin et al., 2010; Howard et al., 2014).
Zilpaterol
Zilpaterol is approved for use in cattle to increase the rate of weight gain, improve feed efficiency, and increase carcass leanness in cattle fed in confinement for slaughter for the last 20 to 40 d on feed. It requires a 3-d withdrawal prior to slaughter in the United States and a 4-d withdrawal in other countries, including Canada.
Comprehensive meta-analyses of the effects of zilpaterol have not been published, but individual peer-reviewed studies do support its use to increase the rate of gain. Feeding zilpaterol to steers the last 20 to 40 d of the feeding period with a 3-d withdrawal increased ADG (Vasconcelos et al., 2008; Elam et al., 2009) and resulted in increased final live weights by an average of 9 kg. Feed intake has been reported to either remain unchanged (Elam et al., 2009) or decrease (Vasconcelos et al., 2008) during the zilpaterol feeding period. Due to these effects on DMI, feed efficiency (gain: feed) is increased by zilpaterol feeding in the last 20 to 40 d in beef steers.
There appears to be an interesting relationship between live BW and HCW changes in cattle fed zilpaterol. Even though live BW is increased an average of 9 kg in beef steers fed zilpaterol compared with control steers, HCW was increased by approximately 15 kg (Vasconcelos et al., 2008; Elam et al., 2009). In heifers, HCW was increased by 11.1 kg due to zilpaterol feeding the last 20 d as compared with non-supplemented heifers (Rathmann et al., 2012). The greater increase in carcass weight relative to live BW is due in part to dramatic changes in dressing percent. Dressing percent is increased approximately 1.5 to 2.0 percentage units due to zilpaterol administration. Increased dressing percentage likely results from increased lean deposition in the carcass. Rathmann et al. (2012) fed steers a finishing ration that included zilpaterol for 0, 20, 30, or 40 d with a 3-d withdrawal and evaluated carcass composition and cutability. They found that zilpaterol had a strong effect on carcass cutout because 22 of the 33 subprimal yields were increased with zilpaterol compared with control. Carcasses from cattle fed zilpaterol were leaner and had more protein and moisture compared with those from the control group. These increases in carcass leanness and saleable meat yield are supported by several other studies of beef cattle (Kellermeier et al., 2009; Shook et al., 2009) and calf-fed Holstein cattle (Beckett et al., 2009; Boler et al., 2009; Holmer et al., 2009). The addition of zilpaterol to beef cattle diets has a negative effect on marbling scores in carcasses. Marbling scores could be reduced 20 to 50 points in carcasses from cattle fed zilpaterol compared with control carcasses (Elam et al., 2009; Vasconcelos et al., 2008).
Lubabegron
Lubabegron is approved for the reduction of ammonia gas emissions per unit of live or carcass weight in beef steers and heifers fed in confinement during the last 14 to 91 d prior to slaughter. Therefore, lubabegron does not have a label claim focused on production efficiency (weight gain or carcass leanness). Instead, the indication for lubabegron includes an environmental component whereby it is labeled to lessen the environmental effect of cattle production by reducing the ammonia emitted.
Ammonia emissions are a result of nitrogen excretion via urinary and fecal production. A number of equations have been developed to predict nitrogen excretion in beef cattle (Waldrip et al., 2013), and it has been concluded that ammonia flux from beef cattle is related to many factors, including nitrogen intake, animal housing conditions, and manure storage facilities (Todd et al., 2011; Gao et al., 2014). Less focus has been dedicated to the relationship of growth-promoting management strategies in reducing gas emissions and, in particular, ammonia emissions.
Reductions of emissions resulting from increased efficiency of tissue accretion could possibly be explained in a modified model of nutrient partitioning. In traditional models of nutrient partitioning, absorbed nutrients are allocated to body tissues in order of priority, with internal organs having the highest priority followed by skeletal muscle and then lastly, adipose tissue. In this way, adipose tissue is seen as only a “storage place” for excess energy. While this traditional view largely ignores the regulatory role of adipose tissue in metabolism, it does provide context for the commonly observed reductions in adipose tissue deposition in favor of skeletal muscle accretion with various growth-promoting technologies. When considering emissions of cattle, emissions of all types (gaseous, urine, and fecal) can be viewed as other “tissue” where nutrients are “deposited.”
A more microscopic view of nutrient partitioning considers subunits of nutrients even to the elemental level (e.g., nitrogen or carbon). This view becomes useful when considering emissions as a part of nutrient partitioning as elements, rather than nutrients, are measured. When nutrients are not deposited into body tissues or are produced and not deposited as part of metabolism, they are excreted as waste through the urine, feces, and gases. Therefore, by increasing the amount of nutrients that are deposited into body tissues, the amount of nutrients excreted by the animal decreases. Conversely, conserving elements from being excreted by the animal increases the availability of those elements that could be deposited into body tissues.
To support the approval of lubabegron to reduce ammonia gas emissions in cattle, several studies were conducted in environmental chambers capable of measuring these gaseous emissions (FDA, 2018). Cattle were fed doses of lubabegron ranging from 1.25 g/ton to 20 g/ton for 14 or 91 d. With 14 d of feeding, lubabegron tended (P = 0.093) to reduce ammonia emissions per pound of HCW in a dose-dependent manner. It is interesting to note that nonstandardized ammonia emissions were decreased, and the reduction of ammonia per pound of HCW was not simply a result of increased HCW, because HCW did not differ between treatments. Additionally, cattle were fed doses of lubabegron ranging from 1.25 g/ton to 20 g/ton for 91 d in environmental chambers (FDA, 2018). In this study, ammonia emissions were reduced both per pound of live weight and per pound of HCW for all doses evaluated. When fed 5 g/ton lubabegron, ammonia emissions per pound HCW were reduced 16%—a combination of an absolute reduction in ammonia by approximately 12% and an increase in carcass weight of approximately 5%. Clinical field safety studies (FDA, 2018) also indicate that weight gain and feed efficiency were not negatively affected by lubabegron administration, but, to date, no commercial data have been published regarding these traits.
Conclusions
In order to determine the parameters for safe use of technologies to increase the efficiency and sustainability of food animal production, the individual characteristics of compounds must be considered. Though all β-ligands share some similarities in terms of structure and function, differences between β-ligands should be recognized when applying the outcomes of risk assessment for their use. When used in accordance with GVP and labeling guidance, tissues from cattle given ractopamine, zilpaterol, and lubabegron are all safe to consume. The individual parameters of safe use (dosage, duration, and withdrawal) differ between β-ligands because they, themselves, are different. Thus, when making risk management decisions, these differences must be recognized. Beta-ligands cannot simply be treated as a monolithic group.
In contrast to ractopamine and zilpaterol, which are approved for use in cattle to increase weight gain and lean meat deposition, lubabegron is approved to reduce ammonium gas emissions per unit of live or carcass weight from cattle. Ractopamine and zilpaterol exert their actions by binding to and activating β1- and β2-AR to increase protein deposition. In contrast, lubabegron is an antagonist of β1- and β2-AR but is an agonist for β3-AR. By virtue of its antagonistic effects at β1- and β2-AR, lubabegron lacks strong lipolytic activity. It can also remain efficacious during prolonged use, as the β3-AR lacks structural features needed for desensitization. Additionally, lubabegron is more extensively metabolized than ractopamine and zilpaterol. The outcomes of the risk assessment for lubabegron as well as its unique properties should be considered in selecting the most appropriate veterinary product to aid in meeting world demand for food animal protein for the growing world population.
Abbreviations
- ADG
average daily gain
- ADI
acceptable daily intake
- ADME
Absorption, Distribution, Metabolism, and Excretion
- ATP
adenosine triphosphate
- BW
body weight
- CAC
Codex Alimentarius Commission
- cAMP
cyclic adenosine monophosphate
- CCRVDF
Codex Committee on Residues of Veterinary Drugs in Food
- CIFOCOss
Chronic Individual Food Consumption Database—summary statistics
- CVM
Center for Veterinary Medicine
- DMI
dry matter intake
- FDA
U.S. Food and Drug Administration
- Gs
G stimulatory
- GVP
good veterinary practice
- HCW
hot carcass weight
- JECFA
Joint FAO/WHO Expert Committee on Food Additives
- LMA
loin muscle area
- LOAEL
lowest observed adverse effect level
- MRL
maximum residue limits
- NEFA
nonesterified fatty acid
- NOAEL
no observed adverse effect limit
- NOEL
no observed effect level
- PKA
protein kinase A
- SβM
selective beta-adrenergic modulator
- WHO
World Health Organization
- β-AA
beta-adrenergic agonists
- β-AR
beta-adrenergic receptors
- β-blockers
beta-adrenergic antagonists
- β-ligands
beta-adrenergic receptor ligands
Conflict of interest statement
This author team was provided original data, administrative assistance, and compensation by Elanco. This work represents the interpretations of the author team. Additionally, A.C.D. has previously received funding to support work regarding β-ligands in cattle and pigs from Elanco and Zoetis. B.J.J. has previously received research funding from Elanco, Merck, and Zoetis to support work regarding β-ligands in cattle.