-
PDF
- Split View
-
Views
-
Cite
Cite
C. A. Young, D. E. Hume, R. L. McCulley, FORAGES AND PASTURES SYMPOSIUM: Fungal endophytes of tall fescue and perennial ryegrass: Pasture friend or foe?, Journal of Animal Science, Volume 91, Issue 5, May 2013, Pages 2379–2394, https://doi.org/10.2527/jas.2012-5951
- Share Icon Share
ABSTRACT
Tall fescue [Lolium arundinaceum (Schreb.) Darbysh. syn. Festuca arundinacea Schreb.] and perennial ryegrass (Lolium perenne L.) are important perennial forage grasses utilized throughout the moderate- to high-rainfall temperate zones of the world. These grasses have coevolved with symbiotic fungal endophytes (Epichloë/Neotyphodium spp.) that can impart bioactive properties and environmental stress tolerance to the grass compared with endophyte-free individuals. These endophytes have proven to be very important in pastoral agriculture in the United States, New Zealand, and Australia, where forage grasses are the principal feed for grazing ruminants. In this review, we describe the biology of these grass-endophyte associations and implications for the livestock industries that are dependent on these forages. Endophyte alkaloid production is put in context with endophyte diversity, and we illustrate how this has facilitated utilization of grasses infected with different endophyte strains that reduce livestock toxicity issues. Utilization of tall fescue and use of perennial ryegrass in the United States, New Zealand, and Australia are compared, and management strategies focused predominantly on the success of endophyte-infected perennial ryegrass in New Zealand and Australia are discussed. In addition, we consider the impact of grass-endophyte associations on the sustainability of pasture ecosystems and their likely response to future changes in climate.
INTRODUCTION
Pastures dominated by forage grass species, managed for grazing animals and/or hay production, cover significant acreage worldwide. These areas are important economically, as they form the base of animal production systems; however, they also provide ecosystem services such as promoting carbon sequestration, preserving biodiversity, reducing soil degradation and loss, and maintaining water quantity and quality (Conant et al., 2001; Hopkins and Alison, 2006). Furthermore, pastures may have an increasing role in agriculture, as markets respond to public demand for more local and sustainable meat and milk production (Lasley et al., 2009).
Tall fescue [Lolium arundinaceum (Schreb.) Darbysh. syn. Festuca arundinacea Schreb.] and perennial ryegrass (Lolium perenne L.) are forage grasses utilized in pastures throughout the mesic, temperate zones of the world (Jung et al., 1996; Fribourg et al., 2009). Like many cool-season grasses, these two species are capable of forming symbiotic associations with fungal endophytes (Epichloë/Neotyphodium spp.). Although grass-fungal endophyte relationships are known to span the symbiotic continuum (Saikkonen et al., 2006), the tall fescue and perennial ryegrass endophyte symbioses are thought to be primarily mutualistic in nature (Saikkonen et al., 1998; Clay and Schardl, 2002). Endophyte infection in these species can confer environmental stress tolerance and protection from pests and herbivory to the host (Clay and Schardl, 2002; Fig. 1). Indeed, the broad-scale environmental adaptability, greater growth rates and competitiveness, and antiherbivore properties exhibited by these two forage species may be linked to their ability to form such symbioses.
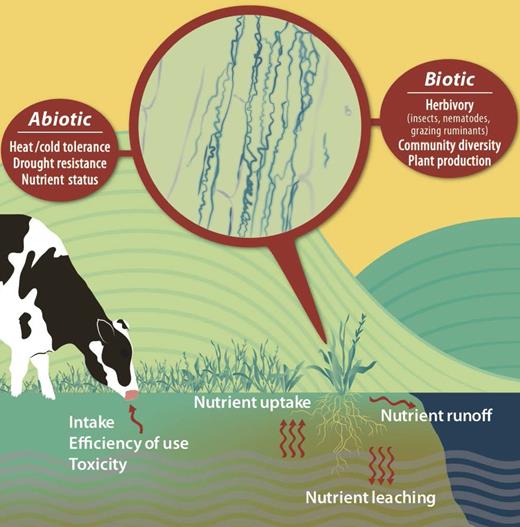
Conceptual diagram illustrating how fungal endophyte symbioses can impact host grass (tall fescue and perennial ryegrass) responses to abiotic factors and affect biotic ecological factors that together can influence pasture ecosystem dynamics, such as nutrient cycling and retention and animal production. See online version for figure in color.
Unfortunately, some of the antiherbivore alkaloid compounds produced by the symbiosis also impair animal performance (Stuedemann and Hoveland, 1988; Fletcher et al., 1999), which has significant economic consequences for the pastoral agricultural sectors, particularly in the United States, New Zealand, and Australia, where these grasses are the principal feed for grazing ruminants. These economic losses have spurred the identification, research, and development of naturally occurring, so-called novel or selected beneficial endophytes capable of producing the insect-active alkaloids but largely incapable of producing some or all of the mammalian-active toxins (Fletcher, 2012). Adoption and utilization of forage grasses infected with these selected endophytes is currently occurring worldwide and is likely to have animal health, economic, and environmental consequences (Malinowski and Belesky, 2006). Research has shown that the presence of these grass-endophyte associations on the landscape can have substantial ecological effects, spanning hierarchical scales from the individual host to the broader community and even the ecosystem level (Omacini et al., 2005; Rudgers and Clay, 2007). The environmental effects of grass symbiosis with the “common toxic” strain of the endophyte capable of producing the full suite of alkaloids are fairly well documented, but much less is known about the potential effects of broad-scale adoption and planting of the selected beneficial endophyte associations (Malinowski and Belesky, 2006). Furthermore, the future sustainability of pasture systems will depend, in part, on how these grass-endophyte associations respond to predicted climate change, a topic that has received limited attention to date (Compant et al., 2010).
In this review, we describe the biology of these grass-endophyte associations and implications for the livestock industries that are dependent on these forages in the United States, New Zealand, and Australia. In addition, we consider the impact of grass-endophyte associations on the sustainability of pasture ecosystems both now and under future climatic conditions.
Epichloë and Neotyphodium, fungal endophytes of grasses
In 1904, Freeman published a pivotal paper on a “seed fungus” present in the grass Lolium temulentum that extensively colonized the developing host seed and was able to transmit into germinating seedlings (Freeman, 1904). It has since been documented that many cool-season grasses contain endophytes from the Epichloë and Neotyphodium genera, collectively called epichloae, of the fungal family Clavicipitaceae (Schardl, 2010). These endophytes have been observed in host members representing most tribes of the Poaceae subfamily Pooideae (Clay and Schardl, 2002; Schardl, 2010). However, host specificity of each endophyte species appears to be restricted to a single host genus or closely related host genera (Schardl, 2010). A strong relationship of host and symbiont coevolution has been observed with significant phylogenetic codivergence in support of such tight host specificity (Schardl et al., 2008).
The success of epichloid endophytes within the host is due to a lifestyle strategy where the fungus systemically infects the aerial parts of the plant without causing disease. The endophyte is able to gain all nutrients from the host apoplast, and hyphal growth within the plant is maintained at the same rate as the host using intercalary extension (Christensen et al., 2008). Neotyphodium species and some Epichloë species can be transmitted vertically in seed where these associations are maintained over successive generations. However, some epichloae exhibit a sexual stage whereby during inflorescence development they ramify on the emerging stem, choking seed development. Isolates expressing the sexual stage are limited to horizontal transmission, but not all sexual isolates reproduce exclusively via the sexual stage, and as such, both vertical and horizontal transmissions can occur, even on the same plant (Sampson, 1933).
Phylogenetic analyses were able to prove that a number of asexual endophytes are interspecific hybrids consisting of genetic material from more than one inferred Epichloë progenitor (Moon et al., 2004). In fact, Neotyphodium coenophialum that colonizes tall fescue is even more complicated and considered a triparental hybrid with three ancestral Epichloë progenitors, Epichloë festucae, E. typhina, and one from the Lolium-associated endophyte (LAE) clade most similar to E. baconii. At least two other hybrid Neotyphodium species associate with tall fescue, N. sp. FaTG-2 (FaTG = Festuca arundinacea taxonomic group), which has E. festucae and the LAE ancestral progenitors, and N. sp. FaTG-3, with E. typhina and LAE ancestral progenitors (Moon et al., 2004). However, not all asexual species are considered interspecific hybrids. Perennial ryegrass is known to have the capability to harbor at least three different endophytes but is most commonly found infected with N. lolii, which is considered an asexual nonhybrid most similar to E. festucae (Moon et al., 2004). Other species that have been identified within perennial ryegrass are a sexual nonhybrid, E. typhina, and an asexual hybrid, N. sp. LpTG-2 (LpTG = Lolium perenne taxonomic group), which has E. festucae and E. typhina ancestry (Moon et al., 2004).
Bioprotective Alkaloids
One of the most intriguing attributes of the epichloid endophytes is their ability to produce a range of bioactive compounds; ergot alkaloids, indole diterpenes, lolines (saturated aminopyrrolizidines), and peramine (pyrrolopyrazine). Although these compounds can be beneficial because they protect the host grass from predation by insects and nematodes (Timper et al., 2005; Popay, 2009b), the negative effects of the ergot alkaloids and indole diterpenes that result in production losses of grazing livestock are equally well known. Once it had been established that the endophyte was the cause of animal toxicity (Bacon et al., 1977; Fletcher and Harvey, 1981; Porter et al., 1981; Fletcher et al., 1999), significant research was undertaken to readily identify each alkaloid, elucidate the biosynthetic pathway, and identify the genes and gene products required for alkaloid production.
As found with other secondary metabolites, the genes required for ergot alkaloids, indole diterpenes, and lolines were identified as gene clusters contained in complex loci most often associated with repetitive sequences (Fleetwood et al., 2007; Young et al., 2009; Schardl et al., 2012). The ergot alkaloid, indole diterpene, and loline alkaloid gene clusters or loci, referred to as the EAS, IDT/LTM, and LOL loci, respectively, each consist of at least 11 genes required for alkaloid production (Spiering et al., 2005; Young et al., 2006; Schardl et al., 2013), whereas only the single nonribosomal peptide synthetase, perA, is required for peramine production (Tanaka et al., 2005).
Initially, research into alkaloid production focused on pathway end products of each alkaloid class; as such, ergovaline (i.e., an ergot alkaloid), lolitrem B, peramine, and lolines have been the most described compounds found in the epichloae. It is now understood that chemotypic diversity seen among and between epichloae species is far more complex, and this is often represented by the presence and absence of genes at each loci (Spiering et al., 2005; Young et al., 2009; Schardl et al., 2013). It is apparent that alterations within the gene content of each locus can explain differences found in alkaloid production, whereby pathway end product variation is explained by the accumulation of pathway intermediates. However, it is yet to be determined how such chemotypic diversity may equate to ecological fitness attributes of the grass-endophyte association.
In-depth genome sequencing of more than 10 haploid epichloid endophytes has provided evidence that when a pathway intermediate is detected as the most abundant compound, it is most often due to the complexity of the associated alkaloid gene locus (Schardl et al., 2013). When genes encoding key steps are missing or nonfunctional, the biosynthetic pathway will be truncated, and intermediate pathway products will accumulate. Alternatively, if none of the genes encoding the enzymatic steps are found in a genome or genes encoding early pathway steps are missing, then that class of compound is unable to be synthesized. To this end, isolates such as common toxic N. lolii are able to produce lolitrem B, an indole diterpene responsible for ryegrass staggers, and contain functional copies of all 11 genes at the IDT/LTM locus (Young et al., 2009). Those unable to produce lolitrems, such as common toxic N. coenophialum, contain no genes or are missing the early pathway genes, ltmG and ltmM (Takach et al., 2012), whereas producers of terpendoles (i.e., indole diterpene pathway intermediates) simply lack the later pathway genes ltmE and ltmJ that encode enzymes required for prenylation of the indole ring (Young et al., 2009; Table 1). Similarly, epichloae isolates that are capable of producing ergovaline, an ergot alkaloid associated with fescue toxicosis, have all genes present and functional within the EAS locus. Strains that only produce chanoclavine, a stable alkaloid intermediate, appear to have only functional early pathway genes dmaW, easE, easF, and easC. Nonergot alkaloid producers tend to lack these essential early pathway genes, although some isolates have been identified that contain EAS genes, but these genes are not expressed (Table 1; Schardl et al., 2013).
Alkaloid production and associated alkaloid gene loci found in commonly used endophyte-grass associations1
Species/host . | Strain2 . | Ergot alkaloids . | Indole diterpenes . | Lolines . | Peramine . |
---|---|---|---|---|---|
Neotyphodium coenophialum/Lolium arundinaceum | Common toxic | EAS cluster complete and functional. Ergovaline producer. | Most or all IDT/LTM genes missing. Unable to produce any indole diterpenes. | LOL cluster complete and functional. Produces N-formylloline and some earlier pathway intermediates. | perA gene complete and functional. Peramine producer. |
N. coenophialum/L. arundinaceum | AR542 (MaxQ) | Most EAS genes missing. Unable to produce ergot alkaloids. | Two LTM genes are missing. Functional early pathway. Terpendole producer. | LOL cluster appears complete but later steps nonfunctional. N-acetylnorloline producer. | perA gene complete and functional. Peramine producer. |
N. coenophialum/L. arundinaceum | AR584 (MaxQ II) | Most EAS genes missing. Unable to produce ergot alkaloids. | Two LTM genes are missing. Functional early pathway. Terpendole producer. | LOL cluster complete and functional. Produces N-formylloline and other earlier pathway intermediates. | perA gene complete and functional. Peramine producer. |
N. lolii/L. perenne | Common toxic | EAS cluster complete and functional. Ergovaline producer. | LTM cluster complete and functional. Lolitrem B producer. | Complete LOL cluster missing. Unable to produce lolines. | perA gene complete and functional. Peramine producer. |
N. lolii/L. perenne | AR1 | EAS cluster (or partial cluster) appears present but nonfunctional. Unable to produce ergot alkaloids. | Two LTM genes are missing. Functional early pathway. Terpendole producer. | Complete LOL cluster missing. Unable to produce lolines. | perA gene complete and functional. Peramine producer. |
N. lolii/L. perenne | AR37 | Complete EAS cluster appears to be missing. Unable to produce ergot alkaloids. | Two LTM genes are missing. Functional early pathway but likely to contain additional IDT/LTM gene(s) for extra prenylation step. | Complete LOL cluster missing. Unable to produce lolines. | perA gene missing reductase domain so nonfunctional. No peramine produced. |
Species/host . | Strain2 . | Ergot alkaloids . | Indole diterpenes . | Lolines . | Peramine . |
---|---|---|---|---|---|
Neotyphodium coenophialum/Lolium arundinaceum | Common toxic | EAS cluster complete and functional. Ergovaline producer. | Most or all IDT/LTM genes missing. Unable to produce any indole diterpenes. | LOL cluster complete and functional. Produces N-formylloline and some earlier pathway intermediates. | perA gene complete and functional. Peramine producer. |
N. coenophialum/L. arundinaceum | AR542 (MaxQ) | Most EAS genes missing. Unable to produce ergot alkaloids. | Two LTM genes are missing. Functional early pathway. Terpendole producer. | LOL cluster appears complete but later steps nonfunctional. N-acetylnorloline producer. | perA gene complete and functional. Peramine producer. |
N. coenophialum/L. arundinaceum | AR584 (MaxQ II) | Most EAS genes missing. Unable to produce ergot alkaloids. | Two LTM genes are missing. Functional early pathway. Terpendole producer. | LOL cluster complete and functional. Produces N-formylloline and other earlier pathway intermediates. | perA gene complete and functional. Peramine producer. |
N. lolii/L. perenne | Common toxic | EAS cluster complete and functional. Ergovaline producer. | LTM cluster complete and functional. Lolitrem B producer. | Complete LOL cluster missing. Unable to produce lolines. | perA gene complete and functional. Peramine producer. |
N. lolii/L. perenne | AR1 | EAS cluster (or partial cluster) appears present but nonfunctional. Unable to produce ergot alkaloids. | Two LTM genes are missing. Functional early pathway. Terpendole producer. | Complete LOL cluster missing. Unable to produce lolines. | perA gene complete and functional. Peramine producer. |
N. lolii/L. perenne | AR37 | Complete EAS cluster appears to be missing. Unable to produce ergot alkaloids. | Two LTM genes are missing. Functional early pathway but likely to contain additional IDT/LTM gene(s) for extra prenylation step. | Complete LOL cluster missing. Unable to produce lolines. | perA gene missing reductase domain so nonfunctional. No peramine produced. |
1Alkaloid production is controlled by genes present at the following loci (Schardl et al., 2013): EAS locus encodes gene products required for ergot alkaloid production (Panaccione et al., 2001; Wang et al., 2004; Fleetwood et al., 2007), IDT/LTM locus encodes gene products required for the production of indole diterpenes, including lolitrem B, terpendoles (earlier pathway intermediates), and janthitrems (requires an additional prenylation step; Young et al., 2006, 2009), LOL locus encodes gene products required for production of saturated pyrrolizidines, including N-formylloline and the earlier pathway intermediate N-acetylnorloline (Spiering et al., 2005), and perA encodes gene products for the production of peramine (Tanaka et al., 2005).
2MaxQ and MaxQ II are trademarks of Grasslanz Technology Ltd., Palmerston North, New Zealand.
Alkaloid production and associated alkaloid gene loci found in commonly used endophyte-grass associations1
Species/host . | Strain2 . | Ergot alkaloids . | Indole diterpenes . | Lolines . | Peramine . |
---|---|---|---|---|---|
Neotyphodium coenophialum/Lolium arundinaceum | Common toxic | EAS cluster complete and functional. Ergovaline producer. | Most or all IDT/LTM genes missing. Unable to produce any indole diterpenes. | LOL cluster complete and functional. Produces N-formylloline and some earlier pathway intermediates. | perA gene complete and functional. Peramine producer. |
N. coenophialum/L. arundinaceum | AR542 (MaxQ) | Most EAS genes missing. Unable to produce ergot alkaloids. | Two LTM genes are missing. Functional early pathway. Terpendole producer. | LOL cluster appears complete but later steps nonfunctional. N-acetylnorloline producer. | perA gene complete and functional. Peramine producer. |
N. coenophialum/L. arundinaceum | AR584 (MaxQ II) | Most EAS genes missing. Unable to produce ergot alkaloids. | Two LTM genes are missing. Functional early pathway. Terpendole producer. | LOL cluster complete and functional. Produces N-formylloline and other earlier pathway intermediates. | perA gene complete and functional. Peramine producer. |
N. lolii/L. perenne | Common toxic | EAS cluster complete and functional. Ergovaline producer. | LTM cluster complete and functional. Lolitrem B producer. | Complete LOL cluster missing. Unable to produce lolines. | perA gene complete and functional. Peramine producer. |
N. lolii/L. perenne | AR1 | EAS cluster (or partial cluster) appears present but nonfunctional. Unable to produce ergot alkaloids. | Two LTM genes are missing. Functional early pathway. Terpendole producer. | Complete LOL cluster missing. Unable to produce lolines. | perA gene complete and functional. Peramine producer. |
N. lolii/L. perenne | AR37 | Complete EAS cluster appears to be missing. Unable to produce ergot alkaloids. | Two LTM genes are missing. Functional early pathway but likely to contain additional IDT/LTM gene(s) for extra prenylation step. | Complete LOL cluster missing. Unable to produce lolines. | perA gene missing reductase domain so nonfunctional. No peramine produced. |
Species/host . | Strain2 . | Ergot alkaloids . | Indole diterpenes . | Lolines . | Peramine . |
---|---|---|---|---|---|
Neotyphodium coenophialum/Lolium arundinaceum | Common toxic | EAS cluster complete and functional. Ergovaline producer. | Most or all IDT/LTM genes missing. Unable to produce any indole diterpenes. | LOL cluster complete and functional. Produces N-formylloline and some earlier pathway intermediates. | perA gene complete and functional. Peramine producer. |
N. coenophialum/L. arundinaceum | AR542 (MaxQ) | Most EAS genes missing. Unable to produce ergot alkaloids. | Two LTM genes are missing. Functional early pathway. Terpendole producer. | LOL cluster appears complete but later steps nonfunctional. N-acetylnorloline producer. | perA gene complete and functional. Peramine producer. |
N. coenophialum/L. arundinaceum | AR584 (MaxQ II) | Most EAS genes missing. Unable to produce ergot alkaloids. | Two LTM genes are missing. Functional early pathway. Terpendole producer. | LOL cluster complete and functional. Produces N-formylloline and other earlier pathway intermediates. | perA gene complete and functional. Peramine producer. |
N. lolii/L. perenne | Common toxic | EAS cluster complete and functional. Ergovaline producer. | LTM cluster complete and functional. Lolitrem B producer. | Complete LOL cluster missing. Unable to produce lolines. | perA gene complete and functional. Peramine producer. |
N. lolii/L. perenne | AR1 | EAS cluster (or partial cluster) appears present but nonfunctional. Unable to produce ergot alkaloids. | Two LTM genes are missing. Functional early pathway. Terpendole producer. | Complete LOL cluster missing. Unable to produce lolines. | perA gene complete and functional. Peramine producer. |
N. lolii/L. perenne | AR37 | Complete EAS cluster appears to be missing. Unable to produce ergot alkaloids. | Two LTM genes are missing. Functional early pathway but likely to contain additional IDT/LTM gene(s) for extra prenylation step. | Complete LOL cluster missing. Unable to produce lolines. | perA gene missing reductase domain so nonfunctional. No peramine produced. |
1Alkaloid production is controlled by genes present at the following loci (Schardl et al., 2013): EAS locus encodes gene products required for ergot alkaloid production (Panaccione et al., 2001; Wang et al., 2004; Fleetwood et al., 2007), IDT/LTM locus encodes gene products required for the production of indole diterpenes, including lolitrem B, terpendoles (earlier pathway intermediates), and janthitrems (requires an additional prenylation step; Young et al., 2006, 2009), LOL locus encodes gene products required for production of saturated pyrrolizidines, including N-formylloline and the earlier pathway intermediate N-acetylnorloline (Spiering et al., 2005), and perA encodes gene products for the production of peramine (Tanaka et al., 2005).
2MaxQ and MaxQ II are trademarks of Grasslanz Technology Ltd., Palmerston North, New Zealand.
Selecting Beneficial Endophytes
The epichloid endophytes can be naturally carried through multiple seed generations and easily distributed across the land; therefore, it seemed feasible to establish other combinations of endophyte and host, considered novel or selected endophyte associations, with naturally occurring endophytes, that reduce livestock toxicity (Latch and Christensen, 1985) because they lack the capability to produce some of the alkaloids. Success of this procedure would require a pure culturable endophyte with low or no negative alkaloid expression, the ability to introduce the endophyte into a number of individual seedlings from either endophyte-free seed or seed heat treated to kill the resident endophyte, and a way of distinguishing the resident, likely to be common toxic endophyte, from an introduced counterpart. Once established, the selected endophyte would need to persist in the new host and transmit effectively through seed production and storage for distribution to farmers and ranchers.
To determine the depth of naturally occurring diversity that might be found within endophyte populations, one must first consider the diversity of the hosts with whom they associate. Tall fescue and perennial ryegrass originated from Eurasia, with Mediterranean tall fescue also found in northern Africa (Sleper and West, 1996; Hannaway et al., 1997). The coevolution of host and symbiont, along with clear ecological advantages of maintaining endophyte infection, indicates that unique environments may provide the source of endophyte diversity equating to differences with alkaloid potential. Although molecular biology technologies can now rapidly provide large amounts of data to determine differences between isolates (Ekanayake et al., 2012; Takach et al., 2012), initial screening for endophyte diversity was determined by traditional mycological methods and analyzing infected plant material for pathway end products (Christensen et al., 1993; TePaske et al., 1993). Of most interest were endophyte associations where lolitrem B and ergot alkaloids could not be detected. However, it was also important to determine if the selected endophyte-infected material would still provide insect resistance and retain the other beneficial features evident with common toxic strains (Hunt and Newman, 2005; Timper et al., 2005; Popay and Thom, 2009).
The tall fescue N. coenophialum strains AR542 and AR584 have been successfully developed and marketed in cultivars for the United States, New Zealand, and Australia (Table 1). Cultivars infected with these strains have good agronomic performance and animal productivity (Hume et al., 2009; Hopkins et al., 2010). The strains AR542 and AR584 have functional genes and biosynthetic pathways for the production of lolines and peramine (Table 1), alkaloids that reduce the level of insect herbivory. No fescue toxicosis occurs in animals grazing AR542- and AR584-infected cultivars (Bouton et al., 2002; Hopkins et al., 2010) as these strains lack most EAS genes and are therefore unable to produce ergot alkaloids (C. A. Young and J. E. Takach, The Samuel Roberts Noble Foundation, Ardmore, Oklahoma, unpublished data; Table 1). They also differ from common toxic N. coenophialum by the production of terpendoles, intermediates in the indole diterpene pathway (Table 1; W. J. Mace AgResearch, New Zealand and C. A. Young, unpublished data). The strains AR542 and AR584 are genetically very similar (Ekanayake et al., 2012), but they produce different loline end products, which indicates differences at the LOL locus between these two isolates (Table 1).
The selected N. lolii endophytes AR1 and AR37 have been extensively used in perennial ryegrass in New Zealand and Australia. Neither AR1 nor AR37 has the capability of producing ergot alkaloids or lolitrem B (Table 1), but they can produce other indole diterpenes (Young et al., 2009). Cultivars containing AR1 are known to produce terpendoles (Young et al., 2009) and peramine and have provided insect protection against Listronotus bonariensis (Argentine stem weevil) and Balanococcus poae (pasture mealybug; Tables 1 and 2; Pennell et al., 2005; Popay and Thom, 2009). The only known group of alkaloids produced by AR37 is the epoxy-janthitrems because the EAS (ergot alkaloid) and LOL (loline) loci are absent from the genome and perA (peramine) contains a deletion of the final reductase domain and appears nonfunctional (Table 1; Fleetwood et al., 2011). However, cultivars containing AR37 have excellent insect resistance that might be due to the bioactivity of the epoxy-janthitrems (Popay and Thom, 2009).
Summary of the various Neotyphodium endophyte associations of tall fescue and perennial ryegrass, with a focus on United States, New Zealand (NZ), and Australia: Livestock toxicity issues, insect resistance properties, management issues for each, and potential ecological effects
Item . | Common toxic endophyte infected . | Selected endophyte infected . | Endophyte free . |
---|---|---|---|
Livestock toxicity1 | |||
Tall fescue | Fescue toxicosis. | No symptoms of fescue toxicosis. | None. |
Perennial ryegrass | Ryegrass staggers, perennial ryegrass toxicosis. | No or reduced symptoms of endophyte toxicosis, dependent on alkaloid profile of endophyte strain. | None. |
Insect resistance1 | |||
Tall fescue | Species that are impacted: U.S.: Fall armyworm (Spodoptera frugiperda); bluegrass webworm (Parapediasia teterella); billbugs (Sphenophorus spp.); leafhopper (Draeculacephala antica); bird cherry oat aphid (Rhopalosiphum padi); greenbug (Schizaphis graminum); sugarcane aphid (Sipha flava); Russian wheat aphid (Diuraphis noxia); southern masked chafer (Cyclocephala lurida); Japanese beetle (Popillia japonica); oriental beetle [Anomala (syn. Exomala) orientalis]; cereal leaf beetle (Chaetocnema pulicaria); barley leaf beetle (Oulema melanopus). NZ and Australia: root aphid (Aploneura lentisci); Argentine stem weevil (Listronotus bonariensis). | Dependent on alkaloid profile of endophyte strain. U.S.: Fall armyworm; bird cherry oat aphid; cereal leaf beetle. NZ and Australia: Black beetle (Heteronychus arator); root aphid; Argentine stem weevil; grass grub (Costelytra zealandica); pasture mealybug (Balanococcus poae). | None in comparison to endophyte-infected plants. |
Perennial ryegrass | Species that are impacted: U.S.: Fall armyworm; cereal leaf beetle; black cutworm (Agrotis ipsilon); Russian wheat aphid; greenbug; bluegrass billbug (Sphenophorus parvulus); bluegrass webworm; sod webworms (Crambus spp.). NZ and Australia: Black beetle; Argentine stem weevil; pasture mealybug; root aphid; crickets (Teleogryllus spp.). | U.S.: Cereal leaf beetle; fall armyworm; black cutworm. NZ and Australia: Black beetle; Argentine stem weevil; pasture mealybug; root aphid; pasture tunnel moth (Philobota spp.); porina (Wiseana cervinata). | None in comparison to endophyte-infected plants. |
Degree of usage and management issues1 | |||
Tall fescue | |||
Acreage | Large in U.S. None or minimal in NZ and Australia. | Small, but increasing in U.S., NZ, and Australia. | Small in U.S. Large in NZ and Australia. |
Pros | Persistent, low-input requirements, can tolerate relatively heavy grazing. | No fescue toxicosis. Greater persistence and grazing tolerance than endophyte free. | No toxicosis. |
Cons | Fescue toxicosis. | May require more attention to management to prevent overgrazing, especially under stressful conditions. | Poor agronomic performance and persistence. |
Perennial ryegrass | |||
Acreage | Minor in U.S. Large in NZ and Australia. | Not sold in the U.S. Widely sold in NZ and Australia. | Majority of acreage in U.S. Small in NZ and Australia. |
Pros | Persistent, low-input requirement, can tolerate relatively heavy grazing. | No or reduced toxicosis. Persistence is better than endophyte free and similar to or better than toxic depending on strain. | No toxicosis. |
Cons | Perennial ryegrass toxicosis, including ryegrass staggers. | Depending on strain, may be less persistent than common toxic. | Poor agronomic performance and persistence. |
Ecological effecs1 | |||
Tall fescue | Increases soil carbon sequestration and nitrification rates; alters soil communities and process; slows litter decomposition; reduces plant and arthropod diversity and abundance; alters herbivory and succession. | Can alter soil trace gas fluxes and nitrification rates, soil microbial communities, arthropod and mammalian herbivory, and plant diversity, depending on the cultivar and novel endophyte strain utilized. | Supports greater plant and arthropod diversity; faster succession; faster litter decomposition; lower carbon sequestration. |
Perennial ryegrass | Alters competition with other plant species and interactions with mycorrhizae; increases soil nitrification rates; alters herbivory. | Increases soil nitrification rates; alters interactions with mycorrhizae; alters herbivory | Lower soil nitrification rates; affects competition with other plant species. |
Item . | Common toxic endophyte infected . | Selected endophyte infected . | Endophyte free . |
---|---|---|---|
Livestock toxicity1 | |||
Tall fescue | Fescue toxicosis. | No symptoms of fescue toxicosis. | None. |
Perennial ryegrass | Ryegrass staggers, perennial ryegrass toxicosis. | No or reduced symptoms of endophyte toxicosis, dependent on alkaloid profile of endophyte strain. | None. |
Insect resistance1 | |||
Tall fescue | Species that are impacted: U.S.: Fall armyworm (Spodoptera frugiperda); bluegrass webworm (Parapediasia teterella); billbugs (Sphenophorus spp.); leafhopper (Draeculacephala antica); bird cherry oat aphid (Rhopalosiphum padi); greenbug (Schizaphis graminum); sugarcane aphid (Sipha flava); Russian wheat aphid (Diuraphis noxia); southern masked chafer (Cyclocephala lurida); Japanese beetle (Popillia japonica); oriental beetle [Anomala (syn. Exomala) orientalis]; cereal leaf beetle (Chaetocnema pulicaria); barley leaf beetle (Oulema melanopus). NZ and Australia: root aphid (Aploneura lentisci); Argentine stem weevil (Listronotus bonariensis). | Dependent on alkaloid profile of endophyte strain. U.S.: Fall armyworm; bird cherry oat aphid; cereal leaf beetle. NZ and Australia: Black beetle (Heteronychus arator); root aphid; Argentine stem weevil; grass grub (Costelytra zealandica); pasture mealybug (Balanococcus poae). | None in comparison to endophyte-infected plants. |
Perennial ryegrass | Species that are impacted: U.S.: Fall armyworm; cereal leaf beetle; black cutworm (Agrotis ipsilon); Russian wheat aphid; greenbug; bluegrass billbug (Sphenophorus parvulus); bluegrass webworm; sod webworms (Crambus spp.). NZ and Australia: Black beetle; Argentine stem weevil; pasture mealybug; root aphid; crickets (Teleogryllus spp.). | U.S.: Cereal leaf beetle; fall armyworm; black cutworm. NZ and Australia: Black beetle; Argentine stem weevil; pasture mealybug; root aphid; pasture tunnel moth (Philobota spp.); porina (Wiseana cervinata). | None in comparison to endophyte-infected plants. |
Degree of usage and management issues1 | |||
Tall fescue | |||
Acreage | Large in U.S. None or minimal in NZ and Australia. | Small, but increasing in U.S., NZ, and Australia. | Small in U.S. Large in NZ and Australia. |
Pros | Persistent, low-input requirements, can tolerate relatively heavy grazing. | No fescue toxicosis. Greater persistence and grazing tolerance than endophyte free. | No toxicosis. |
Cons | Fescue toxicosis. | May require more attention to management to prevent overgrazing, especially under stressful conditions. | Poor agronomic performance and persistence. |
Perennial ryegrass | |||
Acreage | Minor in U.S. Large in NZ and Australia. | Not sold in the U.S. Widely sold in NZ and Australia. | Majority of acreage in U.S. Small in NZ and Australia. |
Pros | Persistent, low-input requirement, can tolerate relatively heavy grazing. | No or reduced toxicosis. Persistence is better than endophyte free and similar to or better than toxic depending on strain. | No toxicosis. |
Cons | Perennial ryegrass toxicosis, including ryegrass staggers. | Depending on strain, may be less persistent than common toxic. | Poor agronomic performance and persistence. |
Ecological effecs1 | |||
Tall fescue | Increases soil carbon sequestration and nitrification rates; alters soil communities and process; slows litter decomposition; reduces plant and arthropod diversity and abundance; alters herbivory and succession. | Can alter soil trace gas fluxes and nitrification rates, soil microbial communities, arthropod and mammalian herbivory, and plant diversity, depending on the cultivar and novel endophyte strain utilized. | Supports greater plant and arthropod diversity; faster succession; faster litter decomposition; lower carbon sequestration. |
Perennial ryegrass | Alters competition with other plant species and interactions with mycorrhizae; increases soil nitrification rates; alters herbivory. | Increases soil nitrification rates; alters interactions with mycorrhizae; alters herbivory | Lower soil nitrification rates; affects competition with other plant species. |
1Key references for livestock toxicity are Bouton et al. (1993, 2002), Fletcher et al. (1999), Bluett et al. (2005), and Hopkins et al. (2010). For insects, key references are Popay and Bonos (2005), Ball et al. (2006, 2011), Clement et al. (2009, 2011), Popay and Thom (2009), and Popay (2009a). For degree of usage and management issues, key references are Fletcher (2012) and Aiken and Strickland (2013). For ecological effects, key references are Marks et al. (1991), Clay et al. (1993, 2005), Clay and Holah (1999), Richmond et al. (2003), Sayer et al. (2004), Lemons et al. (2005), Hunt and Newman (2005), Rudgers and Clay (2007, 2008), Rudgers et al. (2007, 2010), Mack and Rudgers (2008), Siegrist et al. (2010), Bowatte et al. (2011), Liu et al. (2011), McNear and McCulley (2012), Raman et al. (2012), and Iqbal et al. (2012, 2013).
Summary of the various Neotyphodium endophyte associations of tall fescue and perennial ryegrass, with a focus on United States, New Zealand (NZ), and Australia: Livestock toxicity issues, insect resistance properties, management issues for each, and potential ecological effects
Item . | Common toxic endophyte infected . | Selected endophyte infected . | Endophyte free . |
---|---|---|---|
Livestock toxicity1 | |||
Tall fescue | Fescue toxicosis. | No symptoms of fescue toxicosis. | None. |
Perennial ryegrass | Ryegrass staggers, perennial ryegrass toxicosis. | No or reduced symptoms of endophyte toxicosis, dependent on alkaloid profile of endophyte strain. | None. |
Insect resistance1 | |||
Tall fescue | Species that are impacted: U.S.: Fall armyworm (Spodoptera frugiperda); bluegrass webworm (Parapediasia teterella); billbugs (Sphenophorus spp.); leafhopper (Draeculacephala antica); bird cherry oat aphid (Rhopalosiphum padi); greenbug (Schizaphis graminum); sugarcane aphid (Sipha flava); Russian wheat aphid (Diuraphis noxia); southern masked chafer (Cyclocephala lurida); Japanese beetle (Popillia japonica); oriental beetle [Anomala (syn. Exomala) orientalis]; cereal leaf beetle (Chaetocnema pulicaria); barley leaf beetle (Oulema melanopus). NZ and Australia: root aphid (Aploneura lentisci); Argentine stem weevil (Listronotus bonariensis). | Dependent on alkaloid profile of endophyte strain. U.S.: Fall armyworm; bird cherry oat aphid; cereal leaf beetle. NZ and Australia: Black beetle (Heteronychus arator); root aphid; Argentine stem weevil; grass grub (Costelytra zealandica); pasture mealybug (Balanococcus poae). | None in comparison to endophyte-infected plants. |
Perennial ryegrass | Species that are impacted: U.S.: Fall armyworm; cereal leaf beetle; black cutworm (Agrotis ipsilon); Russian wheat aphid; greenbug; bluegrass billbug (Sphenophorus parvulus); bluegrass webworm; sod webworms (Crambus spp.). NZ and Australia: Black beetle; Argentine stem weevil; pasture mealybug; root aphid; crickets (Teleogryllus spp.). | U.S.: Cereal leaf beetle; fall armyworm; black cutworm. NZ and Australia: Black beetle; Argentine stem weevil; pasture mealybug; root aphid; pasture tunnel moth (Philobota spp.); porina (Wiseana cervinata). | None in comparison to endophyte-infected plants. |
Degree of usage and management issues1 | |||
Tall fescue | |||
Acreage | Large in U.S. None or minimal in NZ and Australia. | Small, but increasing in U.S., NZ, and Australia. | Small in U.S. Large in NZ and Australia. |
Pros | Persistent, low-input requirements, can tolerate relatively heavy grazing. | No fescue toxicosis. Greater persistence and grazing tolerance than endophyte free. | No toxicosis. |
Cons | Fescue toxicosis. | May require more attention to management to prevent overgrazing, especially under stressful conditions. | Poor agronomic performance and persistence. |
Perennial ryegrass | |||
Acreage | Minor in U.S. Large in NZ and Australia. | Not sold in the U.S. Widely sold in NZ and Australia. | Majority of acreage in U.S. Small in NZ and Australia. |
Pros | Persistent, low-input requirement, can tolerate relatively heavy grazing. | No or reduced toxicosis. Persistence is better than endophyte free and similar to or better than toxic depending on strain. | No toxicosis. |
Cons | Perennial ryegrass toxicosis, including ryegrass staggers. | Depending on strain, may be less persistent than common toxic. | Poor agronomic performance and persistence. |
Ecological effecs1 | |||
Tall fescue | Increases soil carbon sequestration and nitrification rates; alters soil communities and process; slows litter decomposition; reduces plant and arthropod diversity and abundance; alters herbivory and succession. | Can alter soil trace gas fluxes and nitrification rates, soil microbial communities, arthropod and mammalian herbivory, and plant diversity, depending on the cultivar and novel endophyte strain utilized. | Supports greater plant and arthropod diversity; faster succession; faster litter decomposition; lower carbon sequestration. |
Perennial ryegrass | Alters competition with other plant species and interactions with mycorrhizae; increases soil nitrification rates; alters herbivory. | Increases soil nitrification rates; alters interactions with mycorrhizae; alters herbivory | Lower soil nitrification rates; affects competition with other plant species. |
Item . | Common toxic endophyte infected . | Selected endophyte infected . | Endophyte free . |
---|---|---|---|
Livestock toxicity1 | |||
Tall fescue | Fescue toxicosis. | No symptoms of fescue toxicosis. | None. |
Perennial ryegrass | Ryegrass staggers, perennial ryegrass toxicosis. | No or reduced symptoms of endophyte toxicosis, dependent on alkaloid profile of endophyte strain. | None. |
Insect resistance1 | |||
Tall fescue | Species that are impacted: U.S.: Fall armyworm (Spodoptera frugiperda); bluegrass webworm (Parapediasia teterella); billbugs (Sphenophorus spp.); leafhopper (Draeculacephala antica); bird cherry oat aphid (Rhopalosiphum padi); greenbug (Schizaphis graminum); sugarcane aphid (Sipha flava); Russian wheat aphid (Diuraphis noxia); southern masked chafer (Cyclocephala lurida); Japanese beetle (Popillia japonica); oriental beetle [Anomala (syn. Exomala) orientalis]; cereal leaf beetle (Chaetocnema pulicaria); barley leaf beetle (Oulema melanopus). NZ and Australia: root aphid (Aploneura lentisci); Argentine stem weevil (Listronotus bonariensis). | Dependent on alkaloid profile of endophyte strain. U.S.: Fall armyworm; bird cherry oat aphid; cereal leaf beetle. NZ and Australia: Black beetle (Heteronychus arator); root aphid; Argentine stem weevil; grass grub (Costelytra zealandica); pasture mealybug (Balanococcus poae). | None in comparison to endophyte-infected plants. |
Perennial ryegrass | Species that are impacted: U.S.: Fall armyworm; cereal leaf beetle; black cutworm (Agrotis ipsilon); Russian wheat aphid; greenbug; bluegrass billbug (Sphenophorus parvulus); bluegrass webworm; sod webworms (Crambus spp.). NZ and Australia: Black beetle; Argentine stem weevil; pasture mealybug; root aphid; crickets (Teleogryllus spp.). | U.S.: Cereal leaf beetle; fall armyworm; black cutworm. NZ and Australia: Black beetle; Argentine stem weevil; pasture mealybug; root aphid; pasture tunnel moth (Philobota spp.); porina (Wiseana cervinata). | None in comparison to endophyte-infected plants. |
Degree of usage and management issues1 | |||
Tall fescue | |||
Acreage | Large in U.S. None or minimal in NZ and Australia. | Small, but increasing in U.S., NZ, and Australia. | Small in U.S. Large in NZ and Australia. |
Pros | Persistent, low-input requirements, can tolerate relatively heavy grazing. | No fescue toxicosis. Greater persistence and grazing tolerance than endophyte free. | No toxicosis. |
Cons | Fescue toxicosis. | May require more attention to management to prevent overgrazing, especially under stressful conditions. | Poor agronomic performance and persistence. |
Perennial ryegrass | |||
Acreage | Minor in U.S. Large in NZ and Australia. | Not sold in the U.S. Widely sold in NZ and Australia. | Majority of acreage in U.S. Small in NZ and Australia. |
Pros | Persistent, low-input requirement, can tolerate relatively heavy grazing. | No or reduced toxicosis. Persistence is better than endophyte free and similar to or better than toxic depending on strain. | No toxicosis. |
Cons | Perennial ryegrass toxicosis, including ryegrass staggers. | Depending on strain, may be less persistent than common toxic. | Poor agronomic performance and persistence. |
Ecological effecs1 | |||
Tall fescue | Increases soil carbon sequestration and nitrification rates; alters soil communities and process; slows litter decomposition; reduces plant and arthropod diversity and abundance; alters herbivory and succession. | Can alter soil trace gas fluxes and nitrification rates, soil microbial communities, arthropod and mammalian herbivory, and plant diversity, depending on the cultivar and novel endophyte strain utilized. | Supports greater plant and arthropod diversity; faster succession; faster litter decomposition; lower carbon sequestration. |
Perennial ryegrass | Alters competition with other plant species and interactions with mycorrhizae; increases soil nitrification rates; alters herbivory. | Increases soil nitrification rates; alters interactions with mycorrhizae; alters herbivory | Lower soil nitrification rates; affects competition with other plant species. |
1Key references for livestock toxicity are Bouton et al. (1993, 2002), Fletcher et al. (1999), Bluett et al. (2005), and Hopkins et al. (2010). For insects, key references are Popay and Bonos (2005), Ball et al. (2006, 2011), Clement et al. (2009, 2011), Popay and Thom (2009), and Popay (2009a). For degree of usage and management issues, key references are Fletcher (2012) and Aiken and Strickland (2013). For ecological effects, key references are Marks et al. (1991), Clay et al. (1993, 2005), Clay and Holah (1999), Richmond et al. (2003), Sayer et al. (2004), Lemons et al. (2005), Hunt and Newman (2005), Rudgers and Clay (2007, 2008), Rudgers et al. (2007, 2010), Mack and Rudgers (2008), Siegrist et al. (2010), Bowatte et al. (2011), Liu et al. (2011), McNear and McCulley (2012), Raman et al. (2012), and Iqbal et al. (2012, 2013).
To date, selection of endophytes has focused on the knowledge attained from alkaloid production, yet not all beneficial traits can be explained simply by production of these bioprotective compounds. It is apparent from PCR-based approaches, as well as recent genome sequencing, that other compounds are likely to be produced by the endophyte (Johnson et al., 2007; Schardl et al., 2013). Therefore, it is foreseeable in the future that with more emphasis on genome and transcriptome sequencing, other positive attributes can be identified for endophyte selection.
TALL FESCUE DISTRIBUTION AND USE
Tall fescue is a widely adapted temperate forage grass and, in particular, is more tolerant than perennial ryegrass to soil acidity, salinity, greater soil aluminum concentrations, waterlogging, and summer drought and heat (Buckner et al., 1979; Easton et al., 1994; Fribourg et al., 2009). In the United States, tall fescue is the predominant temperate forage grass occupying approximately 14 million hectares (Ball et al., 1993), mainly in the eastern half of the country (Fig. 2A). It is generally infected with common toxic endophyte (Ball et al., 1993), producing ergot alkaloids that are responsible for an array of toxicities in livestock (Stuedemann and Hoveland, 1988). Endophyte infection is needed to ensure tall fescue is productive, particularly in the southern half of the transition zone in eastern United States (Fig. 2A; Belesky and West, 2009). The majority of the tall fescue in the United States is the continental, summer-active type, but recently, Mediterranean, summer-dormant types have been adopted into forage systems of the hotter and drier edges of the tall fescue adaptation zone (e.g., Texas and Oklahoma; Fig. 2A; Hopkins and Bhamidimarri, 2009; Malinowski et al., 2009).
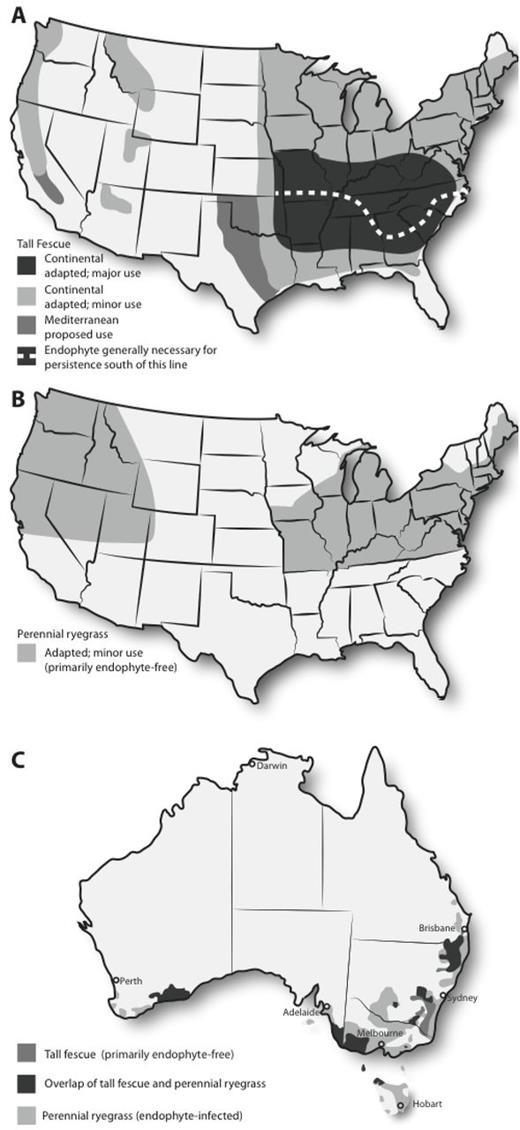
Distribution of use and zones of adaptation for (A) tall fescue (continental summer active and Mediterranean summer dormant types) in the United States, (B) perennial ryegrass in the United States, and (C) tall fescue and perennial ryegrass in Australia. These maps are based on Belesky and West (2009) and C. P. West (Texas Tech University, Lubbock, personal communication) for (A), Hannaway et al. (1997, 1999) with modifications provided by T. Stratton (AgResearch, Ashville, NC, personal communication) for (B), and Wheatley (2005) for (C).
In contrast to the United States, use of tall fescue in the temperate high-rainfall pasture zones of New Zealand and Australia is limited (Belgrave et al., 1990; Easton et al., 1994; Wheatley, 2005). Reasons for the relatively poor use of this potentially valuable forage species are numerous and include slow establishment when compared with perennial ryegrass and failure of farmers to adopt specific grazing management and fertilizer practices (Easton et al., 1994). Tall fescue pasture cultivars for New Zealand and Australia have been bred free of toxic endophytes. Endophyte-infected tall fescue does occur but is mostly confined to turf, roadsides, wastelands, and near waterways (Easton et al., 1994). Endophyte-infected plants can be identified in pastures in a limited number of districts as a naturalized grass (Easton et al., 1994) and in the past was sown in some pastures in Australia (Harris et al., 2008). These naturalized and sown tall fescues are infected with ergovaline-producing endophyte strains and have long been known as highly toxic to livestock (Easton et al., 1994).
The prospect of using endophytes to enhance the agronomic performance of tall fescue cultivars in New Zealand and Australia first became apparent in an agronomic trial in northern New Zealand where frequency of endophyte-infected tillers increased over time (Easton and Cooper, 1997). Further trials examined the agronomic performance of tall fescue infected with selected endophytes. Compared with endophyte-free tall fescue, the equivalent cultivars infected with selected endophytes had enhanced grass production in a range of environments in both New Zealand (Tozer et al., 2007; Hume et al., 2009) and southeastern Australia (Wheatley et al., 2003; Harris et al., 2008; D. E. Hume, unpublished data). The advantages of endophyte-infected tall fescue range from being limited to some seasons or years to being essential for the survival and productivity of tall fescue, with endophyte-free cultivars failing to survive beyond 1 yr. The degree of improvement conferred by endophyte infection varies between regions corresponding closely to the severity of both soil water deficit and insect pest pressure, particularly in the summer-autumn period. As with perennial ryegrass, insect pests appear to be the primary biotic factor involved, with selected endophytes reducing damage from five insect pests (Table 2; Popay, 2009b).
In Australia, tall fescue is limited to 1.1 million hectares of sown pasture, 7% of its potential adaptive area of 16.8 million hectares, whereas perennial ryegrass is found in a much greater area of 5.9 million hectares, 41% of its potential adaptive area of 14.4 million hectares (Hill et al., 1998; Fig. 2C). The areas currently sown with tall fescue are largely within the perennial ryegrass zone, the main exception being the southern tablelands of New South Wales. Most importantly, perennial ryegrass in Australia is endophyte infected, which enhances its survival and productivity (Quigley, 2000; Lowe et al., 2008), whereas sown tall fescue has largely been endophyte free. Given the evidence presented above, it is highly likely that the use of tall fescue with selected endophytes will broaden the area where this species is sown in Australia to be similar to and possibly extend beyond that of endophyte-infected ryegrass. The potential zone in New Zealand for using selected endophyte-infected tall fescue is in areas that experience higher summer temperatures and summer-autumn moisture stress, combined with insect predation that limits persistence and yield of perennial ryegrass (Easton et al., 1994).
PERENNIAL RYEGRASS USE AND DISTRIBUTION
Perennial ryegrass is adapted to a wide range of temperate climates and fertile soils throughout the world (Jung et al., 1996). It is valued for its fast establishment, palatability, high yields of quality herbage, and tolerance to a range of conditions and grazing managements. It is well suited to much of New Zealand and the high-rainfall (i.e., >650 mm/yr) temperate zone of Australia, where it is the preferred sown grass species (Fig. 2C). Old pastures have greater rates of infection with the common toxic endophyte, and most cultivars are sold with an increased amount of common toxic or selected endophyte strains (Hume and Barker, 2005). The pressure of indigenous and introduced insect pests has meant the presence of the endophyte is essential in most regions for grass survival and productivity (Table 2; Popay et al., 1999; Quigley, 2000). In contrast, use of forage perennial ryegrass in the United States is limited to 110,000 ha (Cool and Hannaway, 2004), predominately in the coastal northwest and irrigated intermountain valleys of the West, the Midwest, and the Northeast (Fig. 2B). Most forage perennial ryegrass cultivars are free of endophyte (Mellbye et al., 2006), with no claims being made by U.S. seed companies about endophyte levels or strain.
Perennial ryegrass infected with the common toxic endophyte can cause ill health in a wide range of animal species, including deer, cattle, horses, and sheep (Fletcher et al., 1999; di Menna et al., 2012). The most apparent of these health effects is the occurrence of the neuromuscular disorder ryegrass staggers during late spring, summer, and autumn due to the ingestion of high concentrations of the endophyte-produced alkaloid, lolitrem B (di Menna et al., 2012). Other alkaloids produced by the common toxic ryegrass endophyte, such as ergovaline, also have major effects on livestock, such as reduced productivity and heat stress (Fletcher et al., 1999; Bluett et al., 2005). The toxic effects on livestock are most prevalent in New Zealand and Australia because of the widespread distribution of perennial ryegrass infected with common toxic endophyte. A key difference between these countries is sporadic outbreaks of perennial ryegrass endophyte toxicosis in Australia, where large numbers of animals die (e.g., >100,000 in 2002; Reed et al., 2011b). Reports of toxicity in the United States have been confined to California and Oregon, where sheep and cattle graze endophyte-infected pastures or are fed endophyte-infected straw from seed crops of turf ryegrasses (Galey et al., 1993; Fisher et al., 2004).
Solutions to the Ryegrass-Endophyte Dilemma: Lessons from Down Under
Several strategies exist for farmers to overcome the dilemma in New Zealand and Australia, where the common toxic endophyte in perennial ryegrass impairs livestock performance yet is needed to impart important agronomic traits to the grass host. Each strategy has its own limitations that are reflected in the level of adoption, as discussed subsequently. Many of these strategies are similar to those considered in the case of the tall fescue–endophyte symbiosis also discussed by Aiken and Strickland (2013).
Forage without Endophytes.
A relatively simple solution to avoid the detrimental effects of endophyte on livestock is to eliminate endophyte from sown perennial ryegrass seed. This approach was initially used for tall fescue in the United States and provided excellent animal performance (Bouton et al., 2002). However, it soon became apparent that this solution was unacceptable in practice, as endophyte-free tall fescue pastures were too short-lived in stressful environments (Bouton et al., 1993). Equally for perennial ryegrass in New Zealand and Australia, endophyte-free perennial ryegrass generally suffers from poor agronomic performance largely because of insect pressure (Popay et al., 1999; Quigley, 2000). To date, biocontrol agents for insect pests of endophyte-free ryegrass, such as the parasitic wasp of the Argentine stem weevil introduced into New Zealand from South America (Goldson et al., 1993), did not eliminate the need for endophyte-mediated protection (Popay et al., 2011) because of the presence of four other pests (Table 2). Therefore, endophyte-free pastures are limited to a few regions with low climatic stresses and few insect pests (Widdup and Ryan, 1992) or to farming systems where stress is minimized (e.g., through irrigation) or where a short pasture life is acceptable (e.g., cropping). In addition, it is difficult to establish and maintain endophyte-free pastures. In high-stress environments endophyte-free pastures can be rapidly repopulated with common toxic endophyte-infected ryegrass (Hume and Barker, 2005). Infected plants have superior agronomic performance through better pest protection and reduced intensity of grazing compared with endophyte-free plants (Cosgrove et al., 2002).
Alternative nonendophytic forages can be grown for livestock to consume during the warm seasons of the year when endophyte toxins are greatest and therefore risk of animal toxicity is greater. Nonendophytic grass options will depend on the region and use but include species such as orchard grass (Dactylis glomerata L.), Harding grass (Phalaris aquatica L.), and Bromus spp. (Bromus willdenowii Kunth and B. stamineus Desv.). These species generally have less feed quality than perennial ryegrass, may have other undesirable traits (e.g., phalaris toxicity), and have specific management requirements, all of which impede the widespread adoption of these grasses. Various legumes (e.g., alfalfa, Medicago sativa L.), herbs (e.g., chicory, Cichorium intybus L.), and summer forage Brassica species are also options, but again they require specific management practices, which limits their use. The ability to implement these options may be limited in drought-prone areas, where animals are most at risk from ryegrass-endophyte toxicosis, particularly in dryland hill country where cultivatable land is a small proportion of the total farm.
Diluting Endophyte Toxins.
Reducing the level of ryegrass-endophyte toxicosis through dilution of endophyte toxins in the diet of the animal is possible by manipulating pasture composition and feeding supplements. Subterranean and white clovers (Trifolium subterraneum L. and T. repens L., respectively) are commonly grown with perennial ryegrass in New Zealand and Australia. For Friesian weaner cattle, inclusion of white clover in a grazed sward of common toxic endophyte-infected ryegrass improved BW gains, reduced the intensity of ryegrass staggers, and decreased body temperatures (Cosgrove et al., 1996). However, because endophyte-infected perennial ryegrass is known to suppress both subterranean and white clover (Foot et al., 1988; Sutherland et al., 1999), it can be difficult to obtain sufficiently high proportions of clovers in the pasture to be effective in diluting the endophyte toxins. Furthermore, when clovers would be most beneficial, in the hot, dry conditions of summer and autumn, they are either not present (e.g., subterranean clover is an annual) or present in very small proportions in the pasture as their growth is greatly affected by the adverse climatic conditions. Pastures that include other legume species, herbs, and nonendophytic grass species in the mixture that are better suited to dry summer-autumn conditions [e.g., red clover (T. pratense L.), chicory, orchard grass] will also dilute the endophyte toxins in pasture but may also have associated problems (e.g., bloat when grazing legumes and milk taint in dairy cows grazing chicory).
Feeding supplements (e.g., cereal grains) and conserved forages (e.g., hay and silage) will dilute the intake of endophyte toxins. Ideally, conserved forage should be from endophyte-free species [e.g., alfalfa, corn (Zea mays L.)] as hay and silage made from endophyte-infected ryegrass may have high concentrations of endophyte toxins (Fletcher, 2005).
Grazing Management.
Grazing management of pastures can be changed to reduce the intake of endophyte toxins by grazing animals. In New Zealand, Keogh and Clements (1993) showed that through a rapid rotational grazing management (i.e., daily shifts), ryegrass staggers in sheep was prevented in late summer to early autumn. This is because sheep only grazed the upper portion of the ryegrass tillers, thereby minimizing the intake of lolitrem B, which, like ergovaline, is concentrated primarily in the base of the grass plant (Watson et al., 1999). It has been proposed that the decreased occurrence of ryegrass staggers in dairy cows compared with sheep is because milking cows are generally moved to fresh leafy pasture every 24 h or less (Prestidge, 1993). Although this rapid rotational grazing management strategy can be highly effective when ryegrass staggers is a short-term problem, its success is limited when pastures are toxic for extended periods and regrowth of the grass is limited by dry conditions, as eventually livestock are exposed to increased toxin concentrations in the base of the pasture. In addition, this strategy does not eliminate losses in animal productivity as subclinical effects may still occur (Bluett et al., 2005).
Animal Breeding and Treatments.
Relatively little effort has gone into determining the potential for animal breeding to reduce the effects of endophyte toxicosis. Morris et al. (2007) reported a heritability estimate in sheep of 0.36 for resistance-susceptibility to ryegrass staggers. At least some commercial breeders are selecting for this trait (Hewett, 1983), but this practice is not widespread and is failing to make any apparent impact on the occurrence of this disorder in the wider farming community. With rapid advances in the use of genetic tools in animal breeding programs, this may become a more effective option in the future. The heritability of resistance-susceptibility to the subclinical effects of endophyte is unknown. Feed additives (Reed et al., 2011a) and immunization (Prestidge, 1993) are possible ways to counteract toxicoses, although the costs and difficulty in administering these treatments may limit their adoption by farmers.
Using Selected Endophytes.
The possibility that endophytes may differ in their bioactive properties was pursued by New Zealand researchers with the goal of identifying endophyte strains that were nontoxic to livestock but still imparted bioprotective properties to the host (Latch and Christensen, 1985). These selected endophytes are considered to be the most promising option for breaking the ryegrass-endophyte dilemma (Heeswijck and McDonald, 1992; Prestidge, 1993). This approach relied heavily on research that identified specific endophyte alkaloids responsible for certain bioactivities. For example, peramine is nontoxic to livestock but reduces damage by the Argentine stem weevil (Rowan and Gaynor, 1986; Pownall et al., 1995). To date, five selected endophytes have been released on the market in a wide range of perennial and long-rotation (L. boucheanum Kunth., syn. L. hybridum Hausskn.) ryegrass cultivars in New Zealand and Australia (Milne, 2007). In terms of market uptake, the most successful selected endophyte to date has been the AR1 strain. Within 3 yr of commercial release, cultivars infected with the AR1 endophyte represented 80% of the total endophyte-infected perennial ryegrass seed sales (Milne, 2007). Cultivars infected with AR1 offer improved insect tolerance over endophyte-free equivalents and do not cause any of the animal toxicity problems associated with the common toxic endophyte (Fletcher et al., 1999; Popay et al., 1999).
The prospect that a selected endophyte could be found that was agronomically superior to the common toxic endophyte has been realized in the AR37 strain. Perennial ryegrass infected with AR37 is agronomically superior to perennial ryegrass infected with the common toxic endophyte because it protects ryegrass from a wider range of insect pests, including porina (Aploneura lentisci) and root aphid (Wiseana cervinata; Hume et al., 2007; Popay and Hume, 2011). In terms of animal performance, AR37 does not cause any of the subclinical effects of common toxic endophyte-infected ryegrass but has been associated with ryegrass staggers (Fletcher and Sutherland, 2009; Thom et al., 2013). However, the incidence of ryegrass staggers has been, on average, less frequent and less severe than on common toxic infected perennial ryegrass. Further options for optimizing the characteristics of selected endophytes are being investigated by introducing loline-producing strains to ryegrass from their native hosts of tall fescue and meadow fescue (Easton et al., 2007).
ENDOPHYTE-GRASS SYMBIOSES EFFECTS ON PASTURE SUSTAINABILITY
Sustainable pastures can be broadly defined as those that require relatively few inputs, retain and cycle nutrients efficiently, produce forage of sufficient quality and quantity to support animal gain or hay production and economic goals, and are relatively resilient to climate variability and pest pressure and outbreaks (Scott et al., 2000). They may also contribute to preserving biodiversity, maintaining water quality and quantity, and potentially mitigating future changes in atmospheric CO2 concentrations by sequestering carbon in the soil (Kemp et al., 2000; Franzluebbers, 2005). Fungal endophyte-grass symbioses can impact some of these sustainability variables and ecosystem services (Fig. 1), but the nature and magnitude of these effects can be dependent on the surrounding environmental conditions (e.g., nutrient availability and heat-drought stress; Belesky and West, 2009). Therefore, pasture sustainability under current and future climatic conditions is likely to be governed, at least to some degree, by the response of endophyte-grass relationships. This section will summarize the current state of knowledge on how endophyte-grass symbioses can contribute to these pasture ecosystem variables, focusing only on tall fescue and perennial ryegrass literature. We do not aim to be exhaustive in our literature citations, rather choosing to limit citations to only 1 or 2 references from both grass hosts when possible. There have been several recent reviews that include more extensive literature citations on many of the subjects covered in this section (Popay and Bonos, 2005; Malinowski and Belesky, 2006; Rudgers and Clay, 2007; Compant et al., 2010; McNear and McCulley, 2012; Omacini et al., 2012).
Numerous studies have shown that greater levels of infection with the common toxic endophyte strains substantially increase plant persistence, forage production, and dominance of both tall fescue and perennial ryegrass in pastures (Pedersen et al., 1990; Joost, 1995; Eerens et al., 1997). Similarly, common toxic endophyte strains provide significant pest protection to the host from a variety of insect pests (Table 2), nematodes, and plant diseases (Popay and Bonos, 2005). Both enhanced forage production and the pest protection derived from the endophytes most likely increase the sustainability of these systems. However, as has been noted previously, some of the alkaloids produced by the common toxic endophyte strains can create significant animal health issues, which if severe enough, can substantially reduce the economic profitability of these same systems (Hoveland, 1993). Furthermore, the alkaloids produced by common toxic strains have been shown to alter vole herbivory in ways that reduce pasture plant community diversity (Clay et al., 2005) and impact successional trajectories (Rudgers et al., 2007), negatively impact other wildlife that utilize these ecosystems (Madej and Clay, 1991; Fortier et al., 2000), and alter nonpest insect assemblages and reduce abundances (Rudgers and Clay, 2008). Use of selected endophyte associations can eliminate the grazing livestock toxicity issues associated with the common toxic strain (Fletcher, 1999; Hopkins and Alison, 2006) and maintain, to some degree, pest protection and forage production and persistence enhancements over endophyte-free stands (Bouton et al., 2002; Hunt and Newman, 2005; Popay and Bonos, 2005; Fletcher, 2012); also, limited evidence indicates they may produce less dramatic reductions in plant community diversity compared with the common toxic form (Rudgers et al., 2010). Therefore, the utilization of selected endophytes may best balance the advantages and disadvantages of grass-endophyte effects on aboveground parameters contributing to pasture sustainability and other ecosystem services.
Effects of fungal endophyte infection on belowground processes have received less attention to date than the aboveground effects. This may be related to the fact that the endophyte is rarely, if ever, detected in belowground plant parts (Hinton and Bacon, 1985). However, a growing body of evidence indicates that the endophyte symbiosis can alter a wide variety of belowground variables (McNear and McCulley, 2012; Omacini et al., 2012). Such alterations include altering root exudates (Malinowski et al., 2004; Van Hecke et al., 2005) and nutrient uptake by the plant (Malinowski et al., 2000), reducing the abundance of mycorrhizal spores in the soil and the colonization of grass roots by mycorrhizae (Chu-Chou et al., 1992; Guo et al., 1992; Muller, 2003; Mack and Rudgers, 2008; Liu et al., 2011), changing soil microbial community structure and function (Eerens et al., 1998; Sayer et al., 2004; Buyer et al., 2011; Iqbal et al., 2012), reducing litter decomposition (Lemons et al., 2005; Siegrist et al., 2010), impacting trace gas fluxes from the soil to the atmosphere (Iqbal et al., 2013), and changing overall nutrient cycling and storage (Franzluebbers and Stuedemann, 2005; Bowatte et al., 2011; Iqbal et al., 2012). Some of these effects most likely contribute to the enhanced carbon sequestration observed in common toxic endophyte infected vs. endophyte-free tall fescue stands in the United States (Iqbal et al., 2012), which represents a significant ecosystem service that appears to be enhanced through the grass-endophyte association. However, the majority of this work has focused on evaluating the effect of infection with common toxic endophyte strain. In one tall fescue study, where the selected endophytes AR542 and AR584 were evaluated for their effect on soil inorganic nitrogen pools and soil trace gas fluxes, there was as much variation observed between the stands infected with the different endophyte strains as between the common toxic infected and endophyte-free stands (Iqbal et al., 2013). It remains to be seen whether selected endophytes will reduce, increase, or not alter effect sizes on these belowground processes compared with common toxic forms.
Like many plant-microbe relationships (Compant et al., 2010), grass-endophyte symbioses have been shown to be sensitive to climate change factors, such as elevated atmospheric CO2 concentrations, elevated temperatures, and altered water availability. The majority of this symbiosis work has been performed on tall fescue, although perennial ryegrass pastures have also been shown to be sensitive to climate change (e.g., Newton et al., 2010). Increased atmospheric CO2 stimulates host growth, a common response for cool-season grasses (Morgan, 2005), and influences litter chemistry in complex ways, depending on endophyte infection status and nutrient availability (Marks and Clay, 1990; Newman et al., 2003; Hunt et al., 2005; Chen et al., 2007). Many studies have shown that common toxic endophyte infection improves water relations during and increases tillering and plant recovery after drought (Malinowski and Belesky, 2006), and at least one study has indicated that endophyte infection improves host plant performance under the stress of elevated temperatures (Marks and Clay, 1996). However, in contrast to these results and those illustrating that common toxic infection improves plant persistence in hot-dry environments, a multifactor field climate change study conducted in the United States failed to observe substantial increases in endophyte infection frequency within the tall fescue community over a 5-yr experimental time frame in response to elevated temperature, drought, or the combination of the two treatments (Brosi et al., 2011). Rather, endophyte infection frequency responded to elevated atmospheric CO2 (i.e., increased approximately 10%; Brosi et al., 2011). Furthermore, in another study, tall fescue tillers infected with common toxic endophyte experienced elevated mortality in response to increased temperatures compared with endophyte-free tall fescue, indicating that common toxic endophyte infection may incur a cost to tall fescue under certain environmental conditions (Brosi, 2011). In these climate manipulation studies, elevated heat and reduced water availability increased concentrations of loline and ergot alkaloids in common toxic endophyte infected tillers; however, in the project where atmospheric CO2 was manipulated, increased CO2 concentrations reduced them, in such a way that alkaloid concentrations in plots experiencing both elevated CO2 and heat were not statistically different from those measured in the ambient control plots. Increased concentrations of ergot alkaloids, especially under hotter, drier conditions, are likely to exacerbate fescue toxicosis effects on grazing ruminants, requiring additional management efforts or the adoption of selected endophytes or forage systems. However, if increased atmospheric CO2 mitigates this grass-endophyte symbiotic response, then perhaps toxicosis will not worsen in these regions. These studies clearly illustrate the sensitivity of the grass-endophyte symbiosis to environmental parameters that are likely to be impacted by climate change; however, much remains to be evaluated in this area, especially with regard to toxicosis issues and other potential ecological effects. Furthermore, to our knowledge, there has been no exploration to date of the response of selected endophyte associations to future climatic conditions.
SUMMARY AND CONCLUSIONS
Grass-fungal endophyte symbioses have been important historically in pasture systems in the United States, New Zealand, and Australia and have the ability to contribute to pasture sustainability goals. The diversity of fungal endophytes being explored and the information currently being collected regarding the complex biochemical responses that these endophytes elicit in their grass hosts hold promise for the development of new, selected, beneficial grass-endophyte associations that will further contribute to the sustainability and resiliency of these important grass-based agroecosystems. Much remains to be learned about the mechanisms that cause many of the agronomic and ecological effects of the symbioses. As suggested here and elsewhere (Rasmussen et al., 2009), alkaloids are not the only metabolites altered by endophyte infection. Furthermore, although not highlighted in this review, many studies have shown strong host genotype and fungal strain controls on the symbiosis, the nature of which often depends on surrounding environmental conditions (Cheplick and Faeth, 2009). Given the worldwide distribution of these grass-endophyte symbioses and their importance to both economic and environmental sustainability, it is imperative that we develop a better understanding of the genetic controls and physiological mechanisms that create and maintain the numerous and complex agronomic and ecological consequences of the symbioses and how these controls will likely respond to future climate change.
Footnotes
Based on presentations at the Forages and Pastures Symposium titled “Impact of Fungal-Endophytes on Pasture and Environmental Sustainability” at the Joint Annual Meeting, July 15–19, 2012, Phoenix, Arizona.
LITERATURE CITED
Author notes
The authors are grateful for the assistance of A. Popay and T. Stratton (AgResearch) and many forage experts in the United States, New Zealand, and Australia who answered questions and contributed comments, references, ideas, and opinions helpful in the preparation of this paper. We thank Summer Houghton for generating the illustrations used in this paper. Funds from the College of Agriculture at the University of Kentucky, the ARS-USDA Forage Animal Production Research Unit (58-6440-7-135), and the Kentucky Agricultural Experiment Station (KY006045) helped support R. L. McCulley's contribution to this paper, and support was provided by The Samuel Roberts Noble Foundation to C. A. Young and by AgResearch New Zealand to D. E. Hume.
D. E. Hume is coinventor of U.S. patents (6072107, 6111170, 7976857) that describes AR endophytes. C. A. Young is coinventor of a U.S. patent (US2007/0218461 A1) that describes indole diterpene biosynthesis.