-
PDF
- Split View
-
Views
-
Cite
Cite
Navid Dad, Mohamed A Elsawy, Gavin Humphreys, Alain Pluen, Jian R Lu, Andrew J McBain, A critical view of antimicrobial peptides: exploring their potential and the barriers to realization, Journal of Applied Microbiology, Volume 136, Issue 5, May 2025, lxaf087, https://doi.org/10.1093/jambio/lxaf087
- Share Icon Share
Abstract
The global rise of multidrug-resistant infections highlights the urgent need for innovative therapeutic strategies beyond traditional antibiotics. Antimicrobial peptides (AMPs), naturally occurring in all forms of life and synthetically producible, have garnered significant attention for their broad-spectrum antimicrobial properties and diverse mechanisms of action, including membrane disruption, immune modulation, and biofilm formation inhibition and disruption. Despite great potential, the clinical deployment of AMPs faces significant challenges, including cytotoxicity, low chemical stability, high production costs, and stringent regulatory demands. Innovative strategies, such as AMP-antibiotic conjugation, offer potential solutions to some of these challenges by enhancing efficacy, reducing toxicity, and broadening antimicrobial activity. This review critically evaluates the promise and limitations of AMPs as therapeutic antibacterial agents. We also explore the potential of AMP-antibiotic conjugates, highlighting their potential synergistic effects and the obstacles to their clinical application. Antimicrobial self-assembling peptides are also discussed, with their ability to form nanostructures that may disrupt biofilms and inhibit bacterial communication, representing a promising but complex avenue. A critical evaluation of these emerging strategies, grounded in their practical applicability and translational challenges, is essential to drive meaningful progress in combating antimicrobial resistance.
Introduction
Since the widespread use of Penicillin began in the 1940s, antimicrobial resistance (AMR) has been associated with the deployment of every antibiotic, causing loss of effectiveness (Tang et al. 2023). In 2019, it was estimated that AMR infections caused 4.95 million deaths worldwide (Murray et al. 2022; Stanley et al. 2019). It has been predicted that with the current trend, by the year 2050, AMR infections will cause over 10 million annual deaths (Murray et al. 2022). There is an urgent global need to find solutions to this ever-increasing crisis. AMR can occur due to plasmid transfer, gene mutation, and biofilm formation (Boerlin and Reid-Smith 2008, Aleksandrowicz et al. 2023, Darby et al. 2023, Castañeda-Barba et al. 2024). Biofilm formation can increase bacterial tolerance (Hall and Mah 2017) and biofilms often exhibit antimicrobial susceptibility that is several hundred times lower than their planktonic counterparts (Davies 2003). This tolerance to antimicrobials is rooted in various factors such as the matrix structure, reduced metabolism, and quorum sensing (QS) (Mah 2012, Crabbé et al. 2019, Zhao et al. 2020). In addition, biofilm-related infections are usually caused by multi species communities, frequently rendering single antibiotics inappropriate and ineffective (Lee et al. 2014, Yuan et al. 2020, Short et al. 2024).
Developing new antibiotics is probably not a sustainable solution (MacNair et al. 2023). Therefore, in the past few decades, the focus has shifted to developing alternative approaches. For example, bacteriophages also have conisderable potential but have similarly delayed clinical deployment (Romero-Calle et al. 2019, Bae et al. 2024, and Lambris Hajishengallis 2012). Bacteriophages, like many other viruses, act with high host specificity often at species or even specific level. Whilst sometimes an advantage, this can also present a challenge in the clinic (Koskella and Meaden 2013).
Antimicrobial peptides (AMPs) represent a potential alternative treatment modality for AMR infections (Strempel et al. 2015). Resistance development against AMPs has been claimed to be less widespread, and to progress less rapidly than many traditional antibiotics primarily due to the membrane-disrupting mechanism of action (Tajer et al. 2024). Some AMPs have additionally been reported to have biofilm-formation inhibition and degradation features at relatively low concentrations. (Segev-Zarko et al. 2015, Hancock et al. 2021, Ben Hur et al. 2022) (Table 1 ). The efficacy of AMPs can potentially be improved when conjugated with antibiotics (Radek and Gallo 2007). Potentially synergistic effects of AMPs and antibiotic conjugates could offer a dual mechanism of action against resistant pathogens (Selvaraj and Chen 2023). Compared to AMPs alone, antibiotic-AMP conjugates have potential advantages such as reduced toxicity, broader spectrum of activity, better penetration into the biofilm structure, increased stability, and less likelihood of developing resistance (Epand and Vogel 1999, Rodriguez et al. 2014, Shang et al. 2020, Umstätter et al. 2022, Chou et al. 2023). The AMPs discussed in this text are listed in Table 2.
. | Antibiotic . | Bacteriophage . | AMPs . |
---|---|---|---|
Resistance developmenta | High | Medium | Low |
Spectrumb | Narrow/broad | Very narrow | Broad |
Anti-biofilm activity | Yes* | No | Yes* |
Shelflifec | Long | Long | Short |
Toxicityd | Low | Low | High |
Target organisms | Bacteria, fungi, protozoan** | Bacteria | Bacteria, fungi, virus, protozoan, etc. |
. | Antibiotic . | Bacteriophage . | AMPs . |
---|---|---|---|
Resistance developmenta | High | Medium | Low |
Spectrumb | Narrow/broad | Very narrow | Broad |
Anti-biofilm activity | Yes* | No | Yes* |
Shelflifec | Long | Long | Short |
Toxicityd | Low | Low | High |
Target organisms | Bacteria, fungi, protozoan** | Bacteria | Bacteria, fungi, virus, protozoan, etc. |
Antibiotics and bacteriophages have a higher potential to develop resistance than AMPs. AMPs, on the other hand, have a reduced possibility of bacterial resistance due to their generally more rapid lethal action on the target cells.
Bacteriophages demonstrate precise antimicrobial activity, targeting a specific bacterial genus, species, or strain without affecting other bacteria. In contrast, various antibiotics and AMPs, depending on their structure, can exhibit broad or narrow-spectrum activity.
Conventional antibiotics can be stored at room temperature for 2–3 years, while AMPs and bacteriophages have shorter lifespan.
Conventional antibiotics and bacteriophages have negligible to zero cytotoxicity for human cells. AMPs, due to their electric charge, can potentially damage human cell membranes, leading to cytotoxicity.
Some antibiotics (e.g. ceftazidime and ciprofloxacin) exhibit biofilm activity but face limitations like poor matrix penetration and reduced efficacy against dormant cells. In contrast, AMPs disrupt bacterial membranes, interfere with QS, and degrade the biofilm matrix more effectively.35,36
Most antibiotics are specific to bacteria, but some can also target fungi (e.g. Nystatin) and parasites (e.g. Metronidazole and Tetracyclins) due to overlapping biochemical pathways. Antiviral activity is rare among antibiotics.37–39
. | Antibiotic . | Bacteriophage . | AMPs . |
---|---|---|---|
Resistance developmenta | High | Medium | Low |
Spectrumb | Narrow/broad | Very narrow | Broad |
Anti-biofilm activity | Yes* | No | Yes* |
Shelflifec | Long | Long | Short |
Toxicityd | Low | Low | High |
Target organisms | Bacteria, fungi, protozoan** | Bacteria | Bacteria, fungi, virus, protozoan, etc. |
. | Antibiotic . | Bacteriophage . | AMPs . |
---|---|---|---|
Resistance developmenta | High | Medium | Low |
Spectrumb | Narrow/broad | Very narrow | Broad |
Anti-biofilm activity | Yes* | No | Yes* |
Shelflifec | Long | Long | Short |
Toxicityd | Low | Low | High |
Target organisms | Bacteria, fungi, protozoan** | Bacteria | Bacteria, fungi, virus, protozoan, etc. |
Antibiotics and bacteriophages have a higher potential to develop resistance than AMPs. AMPs, on the other hand, have a reduced possibility of bacterial resistance due to their generally more rapid lethal action on the target cells.
Bacteriophages demonstrate precise antimicrobial activity, targeting a specific bacterial genus, species, or strain without affecting other bacteria. In contrast, various antibiotics and AMPs, depending on their structure, can exhibit broad or narrow-spectrum activity.
Conventional antibiotics can be stored at room temperature for 2–3 years, while AMPs and bacteriophages have shorter lifespan.
Conventional antibiotics and bacteriophages have negligible to zero cytotoxicity for human cells. AMPs, due to their electric charge, can potentially damage human cell membranes, leading to cytotoxicity.
Some antibiotics (e.g. ceftazidime and ciprofloxacin) exhibit biofilm activity but face limitations like poor matrix penetration and reduced efficacy against dormant cells. In contrast, AMPs disrupt bacterial membranes, interfere with QS, and degrade the biofilm matrix more effectively.35,36
Most antibiotics are specific to bacteria, but some can also target fungi (e.g. Nystatin) and parasites (e.g. Metronidazole and Tetracyclins) due to overlapping biochemical pathways. Antiviral activity is rare among antibiotics.37–39
A list of the AMPs mentioned in this review, including their names, origins, sequences, and spectrum.
AMP . | Origin . | Sequence . | Spectrum of activity . |
---|---|---|---|
HNP1 | Humans, primates, mammals | ACYCRIPACIAGERRYGTCIYQGRLWAFCC | Anti-Gram+ & Gram−, antiviral, antifungal, antiparasitic, anti-HIV |
HNP2 | Humans, primates, mammals | CYCRIPACIAGERRYGTCIYQGRLWAFCC | Anti-Gram+ & Gram−, antiviral, antifungal, antiparasitic, Anti-HIV |
HNP3 | Humans, primates, mammals | DCYCRIPACIAGERRYGTCIYQGRLWAFCC | Anti-Gram+ & Gram−, antiviral, antifungal, antiparasitic, Anti-HIV |
HNP4 | Humans, primates, mammals | VCSCRLVFCRRTELRVGNCLIGGVSFTYCCTRV | Anti-Gram+ & Gram−, antiviral, antifungal, antiparasitic, Anti-HIV |
Lip1 | Marine mammals | RRIRIRPPRLPRPRPRPWFPPRFPIPRIPGKR | Anti-Gram+ & Gram− |
Bac8c | Synthetic | RIWVIWRR-NH2 | Anti-Gram+ & Gram−, antifungal, candidacidal |
WUL10 | Bacteriocin | KVLVKYLGGLLKLAALMV-COOH | Anti-MRSA, antibiofilm |
LL-37 | Humans, primates, mammals | LLGDFFRKSKEKIGKEFKRIVQRIKDFLRN LVPRTES | Anti-Gram+ & Gram−, antiviral, antifungal, candidacidal, Antiparasitic, anti-HIV, anti-TB, antibiofilm |
ACD4356 | Bacteriocin | NPKVAHCASQIGRSTAWGAVSGAA TGTAVGQAVGALGGALFGGSMGVIKGS AACVSYLTRHRHH | Anti-Gram+ & Gram−, antibiofilm |
Subtilosin | Bacteriocin | NKGCATCSIGAACLVDGPIPDFEIAGATGLFGLWG | Anti-Gram+ & Gram−, antiviral |
Melimine | Synthetic | TLISWIKNKRKQRPRVSRRRRRRGGRRRR | Anti-Gram+ & Gram− |
B-Lfcin | Mammals | GRRRRSVQWCAVSQPEATKCFQWQRNMR KVRGPPVSCIKRDSPIQCIQA | Anti-Gram+ & Gram−, antiviral, antifungal, candidacidal, anti-HIV, anticancer |
IDR1018 | Synthetic | VRLIVAVRIWRR-NH2 | Anti-Gram+, antibiofilm |
Pexiganan | Synthetic | GIGKFLKKAKKFGKAFVKILKK | Anti-Gram+ & Gram−, antifungal, candidacidal, anti-inflammatory, anticancer |
Protegrin-1 | Mammals | RGGRLCYCRRRFCVCVGR | Anti-Gram+ & Gram−, antifungal, candidacidal, anticancer, antibiofilm |
Pleurocidin | Fish, marine animals | GWGSFFKKAAHVGKHVGKAALTHYL | Anti-Gram+ & Gram−, antifungal, candidacidal, anticancer, antibiofilm |
D-11 | Bacteriocin | RIVQRIKKWLR-NH2 | Anti-Gram−, antibiofilm |
Ubiquicidin | Humans, primates, mammals | KVHGSLARAGKVRGQTPKVAKQEKKKKK TGRAKRRMQYNRRFVNVVPTFGKKKGPNANS | Anti-Gram+ & Gram− |
Priscilicidin | Synthetic | WWRR-NH2 | Anti-Gram+ & Gram−, antifungal |
P- Priscilicidin | Synthetic | WPWRR-NH2 | Anti-Gram+ & Gram−, antifungal |
Melittin | Insects | GIGAVLKVLTTGLPALISWIKRKRQQ | Anti-Gram+ & Gram-, antiviral, antifungal, candidacidal, antiparasitic |
AMP . | Origin . | Sequence . | Spectrum of activity . |
---|---|---|---|
HNP1 | Humans, primates, mammals | ACYCRIPACIAGERRYGTCIYQGRLWAFCC | Anti-Gram+ & Gram−, antiviral, antifungal, antiparasitic, anti-HIV |
HNP2 | Humans, primates, mammals | CYCRIPACIAGERRYGTCIYQGRLWAFCC | Anti-Gram+ & Gram−, antiviral, antifungal, antiparasitic, Anti-HIV |
HNP3 | Humans, primates, mammals | DCYCRIPACIAGERRYGTCIYQGRLWAFCC | Anti-Gram+ & Gram−, antiviral, antifungal, antiparasitic, Anti-HIV |
HNP4 | Humans, primates, mammals | VCSCRLVFCRRTELRVGNCLIGGVSFTYCCTRV | Anti-Gram+ & Gram−, antiviral, antifungal, antiparasitic, Anti-HIV |
Lip1 | Marine mammals | RRIRIRPPRLPRPRPRPWFPPRFPIPRIPGKR | Anti-Gram+ & Gram− |
Bac8c | Synthetic | RIWVIWRR-NH2 | Anti-Gram+ & Gram−, antifungal, candidacidal |
WUL10 | Bacteriocin | KVLVKYLGGLLKLAALMV-COOH | Anti-MRSA, antibiofilm |
LL-37 | Humans, primates, mammals | LLGDFFRKSKEKIGKEFKRIVQRIKDFLRN LVPRTES | Anti-Gram+ & Gram−, antiviral, antifungal, candidacidal, Antiparasitic, anti-HIV, anti-TB, antibiofilm |
ACD4356 | Bacteriocin | NPKVAHCASQIGRSTAWGAVSGAA TGTAVGQAVGALGGALFGGSMGVIKGS AACVSYLTRHRHH | Anti-Gram+ & Gram−, antibiofilm |
Subtilosin | Bacteriocin | NKGCATCSIGAACLVDGPIPDFEIAGATGLFGLWG | Anti-Gram+ & Gram−, antiviral |
Melimine | Synthetic | TLISWIKNKRKQRPRVSRRRRRRGGRRRR | Anti-Gram+ & Gram− |
B-Lfcin | Mammals | GRRRRSVQWCAVSQPEATKCFQWQRNMR KVRGPPVSCIKRDSPIQCIQA | Anti-Gram+ & Gram−, antiviral, antifungal, candidacidal, anti-HIV, anticancer |
IDR1018 | Synthetic | VRLIVAVRIWRR-NH2 | Anti-Gram+, antibiofilm |
Pexiganan | Synthetic | GIGKFLKKAKKFGKAFVKILKK | Anti-Gram+ & Gram−, antifungal, candidacidal, anti-inflammatory, anticancer |
Protegrin-1 | Mammals | RGGRLCYCRRRFCVCVGR | Anti-Gram+ & Gram−, antifungal, candidacidal, anticancer, antibiofilm |
Pleurocidin | Fish, marine animals | GWGSFFKKAAHVGKHVGKAALTHYL | Anti-Gram+ & Gram−, antifungal, candidacidal, anticancer, antibiofilm |
D-11 | Bacteriocin | RIVQRIKKWLR-NH2 | Anti-Gram−, antibiofilm |
Ubiquicidin | Humans, primates, mammals | KVHGSLARAGKVRGQTPKVAKQEKKKKK TGRAKRRMQYNRRFVNVVPTFGKKKGPNANS | Anti-Gram+ & Gram− |
Priscilicidin | Synthetic | WWRR-NH2 | Anti-Gram+ & Gram−, antifungal |
P- Priscilicidin | Synthetic | WPWRR-NH2 | Anti-Gram+ & Gram−, antifungal |
Melittin | Insects | GIGAVLKVLTTGLPALISWIKRKRQQ | Anti-Gram+ & Gram-, antiviral, antifungal, candidacidal, antiparasitic |
A list of the AMPs mentioned in this review, including their names, origins, sequences, and spectrum.
AMP . | Origin . | Sequence . | Spectrum of activity . |
---|---|---|---|
HNP1 | Humans, primates, mammals | ACYCRIPACIAGERRYGTCIYQGRLWAFCC | Anti-Gram+ & Gram−, antiviral, antifungal, antiparasitic, anti-HIV |
HNP2 | Humans, primates, mammals | CYCRIPACIAGERRYGTCIYQGRLWAFCC | Anti-Gram+ & Gram−, antiviral, antifungal, antiparasitic, Anti-HIV |
HNP3 | Humans, primates, mammals | DCYCRIPACIAGERRYGTCIYQGRLWAFCC | Anti-Gram+ & Gram−, antiviral, antifungal, antiparasitic, Anti-HIV |
HNP4 | Humans, primates, mammals | VCSCRLVFCRRTELRVGNCLIGGVSFTYCCTRV | Anti-Gram+ & Gram−, antiviral, antifungal, antiparasitic, Anti-HIV |
Lip1 | Marine mammals | RRIRIRPPRLPRPRPRPWFPPRFPIPRIPGKR | Anti-Gram+ & Gram− |
Bac8c | Synthetic | RIWVIWRR-NH2 | Anti-Gram+ & Gram−, antifungal, candidacidal |
WUL10 | Bacteriocin | KVLVKYLGGLLKLAALMV-COOH | Anti-MRSA, antibiofilm |
LL-37 | Humans, primates, mammals | LLGDFFRKSKEKIGKEFKRIVQRIKDFLRN LVPRTES | Anti-Gram+ & Gram−, antiviral, antifungal, candidacidal, Antiparasitic, anti-HIV, anti-TB, antibiofilm |
ACD4356 | Bacteriocin | NPKVAHCASQIGRSTAWGAVSGAA TGTAVGQAVGALGGALFGGSMGVIKGS AACVSYLTRHRHH | Anti-Gram+ & Gram−, antibiofilm |
Subtilosin | Bacteriocin | NKGCATCSIGAACLVDGPIPDFEIAGATGLFGLWG | Anti-Gram+ & Gram−, antiviral |
Melimine | Synthetic | TLISWIKNKRKQRPRVSRRRRRRGGRRRR | Anti-Gram+ & Gram− |
B-Lfcin | Mammals | GRRRRSVQWCAVSQPEATKCFQWQRNMR KVRGPPVSCIKRDSPIQCIQA | Anti-Gram+ & Gram−, antiviral, antifungal, candidacidal, anti-HIV, anticancer |
IDR1018 | Synthetic | VRLIVAVRIWRR-NH2 | Anti-Gram+, antibiofilm |
Pexiganan | Synthetic | GIGKFLKKAKKFGKAFVKILKK | Anti-Gram+ & Gram−, antifungal, candidacidal, anti-inflammatory, anticancer |
Protegrin-1 | Mammals | RGGRLCYCRRRFCVCVGR | Anti-Gram+ & Gram−, antifungal, candidacidal, anticancer, antibiofilm |
Pleurocidin | Fish, marine animals | GWGSFFKKAAHVGKHVGKAALTHYL | Anti-Gram+ & Gram−, antifungal, candidacidal, anticancer, antibiofilm |
D-11 | Bacteriocin | RIVQRIKKWLR-NH2 | Anti-Gram−, antibiofilm |
Ubiquicidin | Humans, primates, mammals | KVHGSLARAGKVRGQTPKVAKQEKKKKK TGRAKRRMQYNRRFVNVVPTFGKKKGPNANS | Anti-Gram+ & Gram− |
Priscilicidin | Synthetic | WWRR-NH2 | Anti-Gram+ & Gram−, antifungal |
P- Priscilicidin | Synthetic | WPWRR-NH2 | Anti-Gram+ & Gram−, antifungal |
Melittin | Insects | GIGAVLKVLTTGLPALISWIKRKRQQ | Anti-Gram+ & Gram-, antiviral, antifungal, candidacidal, antiparasitic |
AMP . | Origin . | Sequence . | Spectrum of activity . |
---|---|---|---|
HNP1 | Humans, primates, mammals | ACYCRIPACIAGERRYGTCIYQGRLWAFCC | Anti-Gram+ & Gram−, antiviral, antifungal, antiparasitic, anti-HIV |
HNP2 | Humans, primates, mammals | CYCRIPACIAGERRYGTCIYQGRLWAFCC | Anti-Gram+ & Gram−, antiviral, antifungal, antiparasitic, Anti-HIV |
HNP3 | Humans, primates, mammals | DCYCRIPACIAGERRYGTCIYQGRLWAFCC | Anti-Gram+ & Gram−, antiviral, antifungal, antiparasitic, Anti-HIV |
HNP4 | Humans, primates, mammals | VCSCRLVFCRRTELRVGNCLIGGVSFTYCCTRV | Anti-Gram+ & Gram−, antiviral, antifungal, antiparasitic, Anti-HIV |
Lip1 | Marine mammals | RRIRIRPPRLPRPRPRPWFPPRFPIPRIPGKR | Anti-Gram+ & Gram− |
Bac8c | Synthetic | RIWVIWRR-NH2 | Anti-Gram+ & Gram−, antifungal, candidacidal |
WUL10 | Bacteriocin | KVLVKYLGGLLKLAALMV-COOH | Anti-MRSA, antibiofilm |
LL-37 | Humans, primates, mammals | LLGDFFRKSKEKIGKEFKRIVQRIKDFLRN LVPRTES | Anti-Gram+ & Gram−, antiviral, antifungal, candidacidal, Antiparasitic, anti-HIV, anti-TB, antibiofilm |
ACD4356 | Bacteriocin | NPKVAHCASQIGRSTAWGAVSGAA TGTAVGQAVGALGGALFGGSMGVIKGS AACVSYLTRHRHH | Anti-Gram+ & Gram−, antibiofilm |
Subtilosin | Bacteriocin | NKGCATCSIGAACLVDGPIPDFEIAGATGLFGLWG | Anti-Gram+ & Gram−, antiviral |
Melimine | Synthetic | TLISWIKNKRKQRPRVSRRRRRRGGRRRR | Anti-Gram+ & Gram− |
B-Lfcin | Mammals | GRRRRSVQWCAVSQPEATKCFQWQRNMR KVRGPPVSCIKRDSPIQCIQA | Anti-Gram+ & Gram−, antiviral, antifungal, candidacidal, anti-HIV, anticancer |
IDR1018 | Synthetic | VRLIVAVRIWRR-NH2 | Anti-Gram+, antibiofilm |
Pexiganan | Synthetic | GIGKFLKKAKKFGKAFVKILKK | Anti-Gram+ & Gram−, antifungal, candidacidal, anti-inflammatory, anticancer |
Protegrin-1 | Mammals | RGGRLCYCRRRFCVCVGR | Anti-Gram+ & Gram−, antifungal, candidacidal, anticancer, antibiofilm |
Pleurocidin | Fish, marine animals | GWGSFFKKAAHVGKHVGKAALTHYL | Anti-Gram+ & Gram−, antifungal, candidacidal, anticancer, antibiofilm |
D-11 | Bacteriocin | RIVQRIKKWLR-NH2 | Anti-Gram−, antibiofilm |
Ubiquicidin | Humans, primates, mammals | KVHGSLARAGKVRGQTPKVAKQEKKKKK TGRAKRRMQYNRRFVNVVPTFGKKKGPNANS | Anti-Gram+ & Gram− |
Priscilicidin | Synthetic | WWRR-NH2 | Anti-Gram+ & Gram−, antifungal |
P- Priscilicidin | Synthetic | WPWRR-NH2 | Anti-Gram+ & Gram−, antifungal |
Melittin | Insects | GIGAVLKVLTTGLPALISWIKRKRQQ | Anti-Gram+ & Gram-, antiviral, antifungal, candidacidal, antiparasitic |
This review presents a brief introduction to AMPs and then critically assesses their advantages and disadvantages as a therapeutic agents for infections caused by AMR microoganisms. We investigate the potential of conjugating AMPs with antibiotics to provide additional therapeutic options and enhance both safety and efficacy. We also discuss the future of combating AMR infections using short AMPs and self-assembling peptides.
AMPs: multifunctional peptides with varied structures
In general, AMPs are relatively small peptides, some of which have multifaceted activities, including antimicrobial, antiviral, antifungal, antiparasitic, antioxidant, and anti-inflammatory activities (Souness et al. 2000, Melo et al. 2011, Zhao et al. 2011, De Cesare et al. 2020, Loffredo et al. 2024). AMPs play a role in antimicribial defence in many life forms, from bacteria to humans (Nakatsuji and Gallo 2012). AMPs produced by microorganisms probably provide an ecological advantage by inhibiting or killing competing species, thereby reducing competition for resources such as nutrients and space (Meade et al. 2020). AMPs exhibit considerable structural heterogeneity although most commonly share certain features such as cationic charge and amphipathic nature (Laverty 2014). The cationic charge allows them to interact favourably with negatively charged microbial membranes, facilitating their antimicrobial activity. Although most known AMPs have amphipathic structures, some hydrophobic peptides also have bacteriostatic and bactericidal properties (Epand and Vogel 1999).
AMP classification
Classifying AMPs can be challenging due to their diverse nature and characteristics. Based on ‘Antimicrobial Peptide Database (APD3)’ data (https://aps.unmc.edu/), they can be classified based on several factors, such as their origin, biological function, amino acid-rich species, and secondary structure (Fig. 1) (Huan et al. 2020).
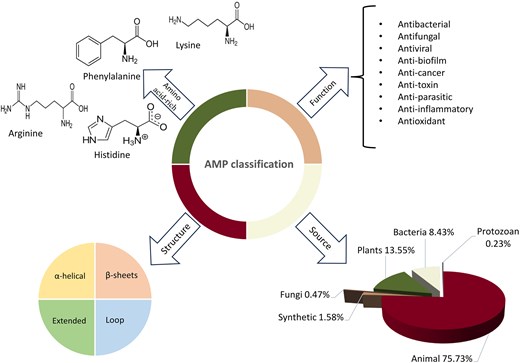
Classification of AMPs based on their structure, origin, function, and amino acid composition. Statistics are derived from the AMP Database (https://aps.unmc.edu).
While AMPs are often regarded as a distinct class of antimicrobials, some, such as polymyxins, gramicidins, bacitracin, and daptomycin, have long been utilized as antibiotics, blurring the line between the two categories (Hancock 1997, Storm et al. 1977). Peptide antibiotics generally target bacterial membranes or cell wall synthesis through distinct mechanisms: polymyxins and gramicidins interact with membrane lipopolysaccharides and disrupt membrane integrity, increasing permeability and causing cell lysis, while bacitracin inhibits peptidoglycan synthesis, and daptomycin induces membrane depolarization, impairing essential cellular functions (Pollock et al. 1994, Miller et al. 2016, Li et al. 2019, Manioglu et al. 2022, Wang et al. 2025). The rapid bactericidal action and comparatively lower propensity for resistance development of some AMPs make them promising candidates for treating multidrug-resistant infections (Stauss-Grabo et al. 2014, Patel and Gallagher 2015, Wenzel et al. 2018, Berditsch et al. 2019, Sun et al. 2024). Additionally, some peptide antibiotics demonstrate useful antibiofilm properties compared to other antibiotics, although effectiveness varies depending on the agent and on biofilm composition and environmental factors (Zaidi et al. 2020, Al-Dulaimi et al. 2021, Gkartziou et al. 2024). Despite their clinical importance, challenges such as compound-specific toxicity limit their broader application, underscoring the need for further optimization and targeted delivery strategies (Ahmed et al. 2017, Roberts et al. 2022, Singh and Mishra 2022, Gappy et al. 2024) (Fig. 2).
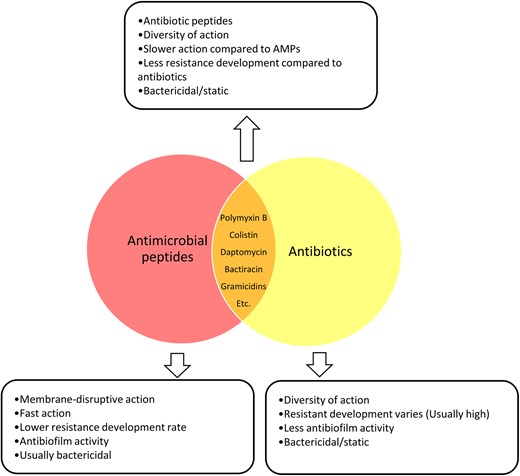
Some AMPs, such as polymyxins, gramicidins, bacitracin, and daptomycin, are considered as antibiotics. These peptides target bacterial membranes or cell wall synthesis. These peptide antibiotics often act rapidly and have a lower tendency for resistance development than conventional antibiotics. Many also exhibit enhanced antibiofilm activity by penetrating biofilms and disrupting their matrix, though efficacy varies. Despite their clinical significance, their use may be limited by compound-specific toxicity.
Pros and Cons of using AMPs as a therapeutic agent
AMPs offer both promising advantages and notable challenges. The benefits of using AMPs as a therapeutic agent are attractive enough to draw attention to them as an alternative way to treat multi-drug resistant (MDR) pathogens (Andersson et al. 2016, Xuan et al. 2023).
Benefits of AMPs
Among the potential advantages of AMPs is their potentially reduced likelihood of inducing bacterial resistance (Rodríguez-Rojas et al. 2015). It has been reported that some AMPs do not trigger bacterial DNA repair mechanisms, a driver of AMR (Yu et al. 2018, Nguyen et al. 2024). Instead, AMPs often directly disrupt bacterial membranes, a process that demands substantial and complex genetic changes for bacteria to counteract. This broad-spectrum mechanism probably reduces the possibility of resistance development. since adopting membrane modifications may be physiologically costly, although it remains possible, particularly with prolonged exposure to sublethal concentrations of antimicrobial agents (Forbes et al. 2014). Furthermore, some AMPs can modulate host immune responses, further complicating bacterial evasion strategies and offering a distinct advantage over antibiotics, which generally target specific bacterial enzymes or pathways,In Staphylococcus aureus, resistance to AMPs such as melittin and pexiganan may reportedly occur through mutations in genes such as pmtR, vraG, atl, and menF. These mutations may lead to changes in the bacterial cell wall, enhanced efflux of AMPs, or other adaptations that diminish peptide effectiveness. While AMP resistance evolves readily in vitro, it is comparatively uncommon in vivo. This is potentially due to the pharmacodynamic properties of AMPs, including steep dose-response curves and narrow mutant selection windows, which limit the development and spread of resistance (El Shazely et al. 2020). Additionally, cationic AMPs interact electrostatically with the anionic phospholipids of the bacterial cytoplasmic membrane. Their amphipathic nature allows them to embed into the bilayer, displacing lipids and ultimately causing cell lysis. These combined properties minimize the risk of resistance evolution, offering a promising alternative to traditional antibiotics (Fjell et al. 2012, Sani et al. 2024).
AMPs frequently exhibit broad spectrum of antimicrobial activity (Suarez-Carmona et al. 2015) with the capability to permeate both Gram-positive and Gram-negative cell membranes (Gong et al. 2021). Human defensins such as α-defensins (HNP1-4) exhibit activity against a range of both Gram-negative and Gram-positive bacteria (Ericksen et al. 2005). Lip1 is reported to have bactericidal and bacteriostatic effects against ESKAPE bacteria (Sola et al. 2020).
Besides broad-spectrum activity, AMPs may demonstrate efficacy against MDR bacteria (Pollini et al. 2017). WUL10, a newly characterized AMP derived from Brevibacillus sp., is reported to show good activity against methicillin-resistant S. aureus (Atipairin et al. 2022). Moreover, AMPs exhibit preventive and remedial capabilities against biofilm formation and degradation (Hirt and Gorr 2013, Peng et al. 2016, Chen et al. 2021, Ben Hur et al. 2022, Cavallo et al. 2024).
AMPs as antibiofilm agents
The tolerance of biofilm to treatment is associated with the physiological implications of growth within the biofilm matrix (often termed the extracellular polymeric material or EPS) which comprises macromolecules such as nucleic acids, lipids, and polysaccharides. The matix helps biofilms attach to surfaces, including implants and host tissues (Di Martino 2018), and can also retard permeation (Singh et al. 2021). Besides the physical barrier, quorum signalling (QS) has been claimed to be an important process in increasing biofilm resilience against antimicrobial agents. QS is associated with cell-to-cell communication in bacterial populations, such as biofilms and may increase the expression of efflux pumps in bacterial cells (Grooters et al. 2024). Furthermore, some accumulated biomolecules in the biofilm matrix, such as eDNA can enhance horizontal transfer of resistance genes to bacteria and also can modify the outermost laters of the bacterial cell due to its electric charge and by concentrating bacterially derived antibiotic-degrading enzymes [including β-lactamases (Dincer et al. 2020)].
AMPs have shown a promising capability in combating biofilm-related infections in relatively low doses compared to antibiotics (Dosler and Karaaslan 2014). LL-37, e.g. has been claimed to have biofilm prevention and degradation properties for the common pathogens S. aureus and Pseudomonas aeruginosa (Ridyard and Overhage 2021). Acidocin 4356 also reportedly has similar activity, towards P. aeruginosa (Modiri et al. 2020, Nadar et al. 2022). Another example of the potential antibiofilm activity of AMPs is subtilosin, which has been reported to inhibit biofilm formation in both Gram-positive and Gram-negative bacteria by blocking QS pathways. Subtilosin is reportedly targets bacterial surface receptors and reduces the production of key signalling molecules, such as Autoinducer-2 (AI-2) in Gram-positive bacteria and violacein in Gram-negative bacteria, without affecting bacterial growth (Algburi et al. 2017).
Factors hindering the therapeutic use of AMPs
Several factors have complicated the clinical application of AMPs. Compared to antibiotics, producing AMPs can be expensive (Hancock and Sahl 2006, Modiri et al. 2021) and AMPs can be susceptible to proteolytic degradation, limiting their application (Svenson et al. 2008). While the mechanism of action of some AMPs is fully understood, much still needs to be learned about the precise mechanisms of action of many other AMPs (Park and Ham 2005, Lin et al. 2020, Shukla et al. 2022; Wang et al. 2023). It is generally agreed that many AMPs interact with bacterial cell membranes due to their cationic and amphipathic nature; this is a simplified definition of their action (Taheri-Araghi and Ha 2010). The exact mechanism varies due to peptide structure, target organism, and other factors (Hale and Hancock 2007, Haney Evan and Mansour 2017).
Immunogenicity is another significant obstacle to using AMPs systemically. Certain AMPs may cause allergic reactions Neudecker et al. 2001, Guryanova and Ovchinnikova 2022) by causing the degranulation of mast cells. These cells release histamine and other mediators, leading to inflammation and allergic symptoms (Subramanian et al. 2013). For example, Some plant AMPs, classified as pathogenesis-related proteins and produced in response to abiotic and biotic stress factors, have been shown to trigger allergic reactions in humans, potentially leading to anaphylactic shock with fatal consequences (Guryanova and Ovchinnikova 2022). AMPs must be determined to be immunogenic and safe before being used as therapeutic agents. Toxicity is another obstacle to the deployment of AMPs. Many AMPs, especially in higher doses, have shown toxicity to eukaryotic cells (Chen and Jiang 2023). The high affinity of AMPs for the negatively charged lipid components of bacterial membranes also means that they can interact with the neutral, zwitterionic phospholipids, and cholesterol in mammalian cell membranes, leading to unwanted haemolytic activity and cytotoxicity (Greco et al. 2020). For instance, a study showed that 4-Leu peptide series, such as 1Kamp-4 L, 2Kamp-4 L, and 3Kamp-4 L, exhibited significant haemolysis (75%–100% haemolysis at 40 μM), indicating high cytotoxicity to mammalian cells (Stone et al. 2019).
Antibiotic-AMP conjugates
Conjugating AMPs to antibiotics can potentially increase the efficacy of both components of the conjugate. For example, resistance to kanamycin is frequently encountered in ESKAPE pathogens (Brezden et al. 2016). However, a study by Mohamed et al. reported that the conjugation of kanamycin and broad spectrum AMP P14LRR resulted in activity against ESKAPE pathogens without showing cytotoxicity. The conjugate, named P14KanS, was reported to exhibit a more than 128-fold increase in antimicrobial activity than kanamycin alone and also showed biofilm degradation properties (Mohamed et al. 2017). In a study by Desgranges et al., the cephalosporin antibiotic cephalothin, was conjugated to the AMP, Bac8c. The cephalothin-Bac8c conjugate showed antimicrobial activity in the penicillin-resistant Escherichia coli ATCC 35218 and different strains of MRSA, all of which are β-lactamase positive strains (Desgranges et al. 2012). In 2022, Umstätter et al. investigated vancomycin-resistant Enterococci and developed ‘highly active polycationic peptide-vancomycin conjugates’ known as VAN: PEG1-3. These conjugates are PEGylated derivatives of FU002, a conjugate of vancomycin with an AMP called hexa-arginine. VAN: PEG1, VAN: PEG2, and VAN: PEG3 differ in the length of their polyethylene glycol (PEG) chains. The conjugates effectively overcame vancomycin resistance and demonstrated enhanced antimicrobial activity and pharmacokinetics against VanB and VanC vancomycin-resistant Enterococci.
The attachment of hexa-arginine to vancomycin has been reported to improve the binding of the antibiotic to bacterial cell walls, including in resistant strains, while retaining or enhancing antimicrobial activity. Furthermore, The addition of PEG chains reportedly further improved pharmacokinetic properties by increasing stability, reducing proteolytic degradation, and extending the conjugates’ half-life in the bloodstream (Umstätter et al. 2022).
AMPs can be conjugated to antibiotics in several ways, including chemical conjugation, genetic fusion, and bioconjugation (Feng et al. 2012, Umstätter et al. 2022).
Advantages of conjugating AMPs with antibiotics
Conjugating AMPs with antibiotics has the potential to produce a synergistic effect and enhance antibiofilm activity. Furthermore, this conjugation may result in reduced cytotoxicity compared to using AMPs alone.
Antimicrobial synergy
Synergy refers to the cumulative impact of two or more different agents producing a greater effect than the sum of their individual effects. Synergy can be measured and expressed as a fractional inhibitory concentration (FIC). The FIC indicates the concentration at which the drugs work together to inhibit the growth of a microorganism and serves as an index for quantifying drug interactions across three distinct domains: ≤0.5 denotes synergy, 0.5–4 signifies no interaction between two agents, and >4 indicates antagonism (Odds 2003). AMPs can show synergistic effects in combination with commercial antibiotics (Fig. 3)

FIC calculation equation. ΣFIC is the sum of the FICs. FICA is the FIC of drug A. FICB is the FIC of drug B. CA and CB are the concentrations of drugs A and B used in a combination treatment, respectively, required to inhibit bacterial growth. MICA and MICB are the minimum inhibitory concentrations of drugs A and B, respectively. FIC ≤ 0.5 denotes synergy, 0.5 < FIC < 4 signifies no interaction between two agents, and FIC > 4 indicates antagonism.
Mechanisms behind AMP-antibiotic synergistic effects
The mechanisms of synergy between AMPs and antibiotics include the high membrane activity of AMPs such as ACD4356 and hBD-3 (Böhling et al. 2006, Modiri et al. 2020) combined with the intracellular targets of many antibiotics (e.g. azithromycin and kanamycin) (Urbancic and Grayson 2017, Heidary et al. 2022). Several factors, such as the selective permeability of bacterial cell membranes, can retard cytoplasmic access to antibiotics. Such reduced permeability can be attributed to the outer membrane structure of Gram-negative bacteria and mycobacteria, which act as physical barriers, and the activity of efflux pumps, which actively expel antibiotics from the cell, reducing their intracellular concentrations and further enhancing the permeability barrier (Edelstein 2004, Fair and Tor 2014, Wilson 2014).
MDR P. aeruginosa can develop by overexpressing efflux pumps such as MexCD-OprJ and MexEF-OprN, which actively expel antibiotics such as ciprofloxacin. This reduces the intracellular accumulation od ciprofloxacin, limiting its permeability and contributing to selective permeability by maintaining a barrier against harmful substances (Rehman et al. 2019). It has been reported that bovine lactoferricin (B-Lfcin) can increase cell membrane permeability by acting as an efflux pump inhibitor facililitating the access of ciprofloxacin to intracellular targets of MDR P. aeruginosa (Oo et al. 2010). Table 3 presents a list of AMPs with synergistic effects with antibiotics.
Combination and conjugations of AMPs and conventional antibiotics and their range of activity.
AMP . | Antibiotic . | Type . | Organism . | Reference . |
---|---|---|---|---|
B-Lfcin | Ciprofloxacin | Combination | P. aeruginosa | (Oo et al. 2010) |
Ceftazidime | ||||
Pexiganan | Colistin | Combination | Acinetobacter baumannii | (Cirioni et al. 2016) |
Protegrin-1 | Colistin | Combination | MDR Klebsiella pneumoniae | Mhlongo et al. 2023 |
Fosfomycin | ||||
Meropenem | ||||
Tigecycline | ||||
Pleurocidin | Ampicillin | Combination | P. aeruginosa ATCC 27 853 | (Choi and Lee 2012) |
Chloramphenicol | P. aeruginosa ATCC 27 853 | |||
Erythromycin | Propionibacterium acnes ATCC 6919 | |||
Pr. acnes ATCC 6919 | ||||
S. aureus ATCC 25 923 | ||||
S. aureus ATCC 25 923 | ||||
E. coli ATCC 25 922 | ||||
E. coli O-157 ATCC 43 895 | ||||
E. coli ATCC 25 922 | ||||
E. coli O-157 ATCC 43 895 | ||||
Enterococcus faecium ATCC 19 434 | ||||
P14LRR | Kanamycin | Conjugation | En. faecium | (Mohamed et al. 2017) |
K. pneumoniae | ||||
A. baumannii | ||||
MRSA | ||||
Methicillin-resistant S. epidermidis (MRSE) | ||||
Vancomycin-resistant En. faecium | ||||
Colistin-resistant P. aeruginosa | ||||
Mycobacterium tuberculosis | ||||
Bac8c | Cephalosporin | Conjugation | B-lactam resistant E. coli | (Desgranges et al. 2012) |
MRSA | ||||
PEG1-3 | Vancomycin | Conjugation | vancomycin-resistant enterococci spp. | (Umstätter et al. 2022) |
WW-158 | Kanamycin | S. aureus ATCC 6538 | (Salama 2023) | |
MRSA | ||||
E. coli ATCC 8739 | ||||
ESBL E. coli BAA-3054 | ||||
Melimine | Ciprofloxacin | Combination | MDR P. aeruginosa | (Yasir et al. 2021) |
MRSA | ||||
LL-37 | Polymyxin B | Combination | E. coli | (Ridyard et al. 2023) |
P. aeruginosa PAO1 | ||||
D-11 | macrolide azithromycin | Combination | P. aeruginosa | (Xia et al. 2021) |
IDR1018 | Vancomycin | Conjugation | MRSA | (Etayash et al. 2021) |
S. epidermidis | ||||
En. faecium | ||||
Ubiquicidin | Chloramphenicol | Conjugation | E. coli | (Chen et al.2015) |
S. aureus | ||||
P. aeruginosa | ||||
AMP . | Antibiotic . | Type . | Organism . | Reference . |
---|---|---|---|---|
B-Lfcin | Ciprofloxacin | Combination | P. aeruginosa | (Oo et al. 2010) |
Ceftazidime | ||||
Pexiganan | Colistin | Combination | Acinetobacter baumannii | (Cirioni et al. 2016) |
Protegrin-1 | Colistin | Combination | MDR Klebsiella pneumoniae | Mhlongo et al. 2023 |
Fosfomycin | ||||
Meropenem | ||||
Tigecycline | ||||
Pleurocidin | Ampicillin | Combination | P. aeruginosa ATCC 27 853 | (Choi and Lee 2012) |
Chloramphenicol | P. aeruginosa ATCC 27 853 | |||
Erythromycin | Propionibacterium acnes ATCC 6919 | |||
Pr. acnes ATCC 6919 | ||||
S. aureus ATCC 25 923 | ||||
S. aureus ATCC 25 923 | ||||
E. coli ATCC 25 922 | ||||
E. coli O-157 ATCC 43 895 | ||||
E. coli ATCC 25 922 | ||||
E. coli O-157 ATCC 43 895 | ||||
Enterococcus faecium ATCC 19 434 | ||||
P14LRR | Kanamycin | Conjugation | En. faecium | (Mohamed et al. 2017) |
K. pneumoniae | ||||
A. baumannii | ||||
MRSA | ||||
Methicillin-resistant S. epidermidis (MRSE) | ||||
Vancomycin-resistant En. faecium | ||||
Colistin-resistant P. aeruginosa | ||||
Mycobacterium tuberculosis | ||||
Bac8c | Cephalosporin | Conjugation | B-lactam resistant E. coli | (Desgranges et al. 2012) |
MRSA | ||||
PEG1-3 | Vancomycin | Conjugation | vancomycin-resistant enterococci spp. | (Umstätter et al. 2022) |
WW-158 | Kanamycin | S. aureus ATCC 6538 | (Salama 2023) | |
MRSA | ||||
E. coli ATCC 8739 | ||||
ESBL E. coli BAA-3054 | ||||
Melimine | Ciprofloxacin | Combination | MDR P. aeruginosa | (Yasir et al. 2021) |
MRSA | ||||
LL-37 | Polymyxin B | Combination | E. coli | (Ridyard et al. 2023) |
P. aeruginosa PAO1 | ||||
D-11 | macrolide azithromycin | Combination | P. aeruginosa | (Xia et al. 2021) |
IDR1018 | Vancomycin | Conjugation | MRSA | (Etayash et al. 2021) |
S. epidermidis | ||||
En. faecium | ||||
Ubiquicidin | Chloramphenicol | Conjugation | E. coli | (Chen et al.2015) |
S. aureus | ||||
P. aeruginosa | ||||
Combination and conjugations of AMPs and conventional antibiotics and their range of activity.
AMP . | Antibiotic . | Type . | Organism . | Reference . |
---|---|---|---|---|
B-Lfcin | Ciprofloxacin | Combination | P. aeruginosa | (Oo et al. 2010) |
Ceftazidime | ||||
Pexiganan | Colistin | Combination | Acinetobacter baumannii | (Cirioni et al. 2016) |
Protegrin-1 | Colistin | Combination | MDR Klebsiella pneumoniae | Mhlongo et al. 2023 |
Fosfomycin | ||||
Meropenem | ||||
Tigecycline | ||||
Pleurocidin | Ampicillin | Combination | P. aeruginosa ATCC 27 853 | (Choi and Lee 2012) |
Chloramphenicol | P. aeruginosa ATCC 27 853 | |||
Erythromycin | Propionibacterium acnes ATCC 6919 | |||
Pr. acnes ATCC 6919 | ||||
S. aureus ATCC 25 923 | ||||
S. aureus ATCC 25 923 | ||||
E. coli ATCC 25 922 | ||||
E. coli O-157 ATCC 43 895 | ||||
E. coli ATCC 25 922 | ||||
E. coli O-157 ATCC 43 895 | ||||
Enterococcus faecium ATCC 19 434 | ||||
P14LRR | Kanamycin | Conjugation | En. faecium | (Mohamed et al. 2017) |
K. pneumoniae | ||||
A. baumannii | ||||
MRSA | ||||
Methicillin-resistant S. epidermidis (MRSE) | ||||
Vancomycin-resistant En. faecium | ||||
Colistin-resistant P. aeruginosa | ||||
Mycobacterium tuberculosis | ||||
Bac8c | Cephalosporin | Conjugation | B-lactam resistant E. coli | (Desgranges et al. 2012) |
MRSA | ||||
PEG1-3 | Vancomycin | Conjugation | vancomycin-resistant enterococci spp. | (Umstätter et al. 2022) |
WW-158 | Kanamycin | S. aureus ATCC 6538 | (Salama 2023) | |
MRSA | ||||
E. coli ATCC 8739 | ||||
ESBL E. coli BAA-3054 | ||||
Melimine | Ciprofloxacin | Combination | MDR P. aeruginosa | (Yasir et al. 2021) |
MRSA | ||||
LL-37 | Polymyxin B | Combination | E. coli | (Ridyard et al. 2023) |
P. aeruginosa PAO1 | ||||
D-11 | macrolide azithromycin | Combination | P. aeruginosa | (Xia et al. 2021) |
IDR1018 | Vancomycin | Conjugation | MRSA | (Etayash et al. 2021) |
S. epidermidis | ||||
En. faecium | ||||
Ubiquicidin | Chloramphenicol | Conjugation | E. coli | (Chen et al.2015) |
S. aureus | ||||
P. aeruginosa | ||||
AMP . | Antibiotic . | Type . | Organism . | Reference . |
---|---|---|---|---|
B-Lfcin | Ciprofloxacin | Combination | P. aeruginosa | (Oo et al. 2010) |
Ceftazidime | ||||
Pexiganan | Colistin | Combination | Acinetobacter baumannii | (Cirioni et al. 2016) |
Protegrin-1 | Colistin | Combination | MDR Klebsiella pneumoniae | Mhlongo et al. 2023 |
Fosfomycin | ||||
Meropenem | ||||
Tigecycline | ||||
Pleurocidin | Ampicillin | Combination | P. aeruginosa ATCC 27 853 | (Choi and Lee 2012) |
Chloramphenicol | P. aeruginosa ATCC 27 853 | |||
Erythromycin | Propionibacterium acnes ATCC 6919 | |||
Pr. acnes ATCC 6919 | ||||
S. aureus ATCC 25 923 | ||||
S. aureus ATCC 25 923 | ||||
E. coli ATCC 25 922 | ||||
E. coli O-157 ATCC 43 895 | ||||
E. coli ATCC 25 922 | ||||
E. coli O-157 ATCC 43 895 | ||||
Enterococcus faecium ATCC 19 434 | ||||
P14LRR | Kanamycin | Conjugation | En. faecium | (Mohamed et al. 2017) |
K. pneumoniae | ||||
A. baumannii | ||||
MRSA | ||||
Methicillin-resistant S. epidermidis (MRSE) | ||||
Vancomycin-resistant En. faecium | ||||
Colistin-resistant P. aeruginosa | ||||
Mycobacterium tuberculosis | ||||
Bac8c | Cephalosporin | Conjugation | B-lactam resistant E. coli | (Desgranges et al. 2012) |
MRSA | ||||
PEG1-3 | Vancomycin | Conjugation | vancomycin-resistant enterococci spp. | (Umstätter et al. 2022) |
WW-158 | Kanamycin | S. aureus ATCC 6538 | (Salama 2023) | |
MRSA | ||||
E. coli ATCC 8739 | ||||
ESBL E. coli BAA-3054 | ||||
Melimine | Ciprofloxacin | Combination | MDR P. aeruginosa | (Yasir et al. 2021) |
MRSA | ||||
LL-37 | Polymyxin B | Combination | E. coli | (Ridyard et al. 2023) |
P. aeruginosa PAO1 | ||||
D-11 | macrolide azithromycin | Combination | P. aeruginosa | (Xia et al. 2021) |
IDR1018 | Vancomycin | Conjugation | MRSA | (Etayash et al. 2021) |
S. epidermidis | ||||
En. faecium | ||||
Ubiquicidin | Chloramphenicol | Conjugation | E. coli | (Chen et al.2015) |
S. aureus | ||||
P. aeruginosa | ||||
AMP-antibiotic conjugates and antibiofilm activity
It has been claimed that more than 80% of bacteria on Earth—including those associated with the human body occur principally in biofilm form (Flemming and Wuertz 2019). The US National Institutes of Health proposed that ∼70% of infectious diseases relate to biofilms (Jamal et al. 2018). Biofilms can exacerbate severe chronic diseases such as chronic wounds, periodontitis, osteomyelitis, chronic urinary tract infections, and device/implant-related infections and pose a threat to human health (Kovach et al. 2017, Khatoon et al. 2018, Masters et al. 2019, Johnston et al. 2021). They are hard to treat and are usually not sensitive to conventional antibiotics. Studies have shown that biofilm can show up to 1000-fold resistance to treatment compared to planktonic cells (Davies 2003, Wolfmeier et al. 2018). However, most microbiological research on antibiotics and AMPs has focused on planktonic bacteria or inhibiting biofilm formation rather than eradication (Perry and Tan 2023).
Whilst some AMPs show promising antibiofilm activity, this can potentially be enhanced further by combination with antibiotics (Yasir et al. 2021). In a study by Ridyard et al., a combination of LL-37 and Polymyxin B markedly inhibited biofilm formation in P. aeruginosa and E. coli (Ridyard et al. 2023). In a separate report, a cathelicidin-derived short AMP, D-11, increased the permeability of the outer membrane of Gram-negative bacteria. Combined with other antibiotics, including the macrolide azithromycin, it has excellent antibiofilm activity against P. aeruginosa (Xia et al. 2021).
In 2021, Etayash et al. conjugated the novel synthetic AMP IDR1018, to vancomycin. Compared to vancomycin alone, the V-IDR1018 conjugate was reported to show a significant bactericidal effect on MRSA in 30 min showing 16-fold higher antibiofilm activity than vancomycin and 2-fold higher antibiofilm activity than the peptide alone. It reportedly eradicated ∼90% of the formed biofilm without showing any toxicity in a murine model. Interestingly, V-IDR1018 also reportedly showed activity against En. faecium, which is not susceptible to vancomycin (Etayash et al. 2021). It should be noted that studies of conjugates that have progressed to in vivo testing are uncommon, moreso for clinical trials in humans.
Challenges of AMP-antibiotic conjugates
Whilst AMP-antibiotic conjugates offer promising benefits, they also present challenges which include production costs and structural complexity, which can complicate treatment.
Challenge: cost, complexity, and accessibility
Although existing AMP-antibiotic conjugates show promising antimicrobial and antibiofilm activity, synthesising such conjugates can be challenging. A comprehensive study on the structure of the peptide and antibiotic, their modes of action, and their compatibility are needed for designing a functional conjugate (Ghaffar et al. 2015). On the other hand, designing and producing a conjugate can be expensive, potentially driving up costs. The precise cost of producing AMP-antibiotic conjugates remains uncertain, but studies have provided some insight. Synthesizing a solid-based peptide alone can range between $100 and $600 per gram (Chaudhary et al. 2023). Additional expenses for conjugation with antibiotics—such as linker synthesis, antibiotic production, and conjugation—would need to be factored in on top of this initial price point. This issue is particularly significant for deployment in underdeveloped countries. This is likely to exacerbate the challenge of access to effective treatments (Boutayeb 2010).
Challenges of multi-species biofilm infections
Although AMPs and AMP-antibiotic conjugates have shown promising antibiofilm potential in vitro and in vivo, the situation differs regarding an actual infection (Dosler and Karaaslan 2014, Algburi et al. 2017). Biofilm-related conditions, such as diabetic foot ulcers or vaginosis, consists of a mixture of microorganisms, such as Gram-positive and Gram-negative bacteria, and different species of fungi, such as Candida albicans (Dilhari et al. 2020, Johnson et al. 2023).
This particular characteristic and other attributes of biofilm infection render traditional therapies ineffective. Conventional therapeutic agents, such as antibiotics, typically lack the expansive spectrum needed to combat multi-species biofilm infections comprehensively (Sedlacek and Walker 2007, She et al. 2019, Alvim et al. 2022, Yan et al. 2024). However, AMPs and AMP-antibiotic conjugates offer a partial solution to this challenge with their broader range of activity (Choi and Lee 2012, Mohamed et al. 2017). Nonetheless, these approaches have unequal effectiveness against various microorganisms within the multi-species biofilm, which is a drawback (Segev-Zarko et al. 2015). Consequently, utilizing AMP-antibiotic conjugates may disrupt the initial structure of biofilm, potentially leading to the emergence of a secondary, more resilient infection post-treatment (Kwiecińska-Piróg et al. 2018).
The future of AMPs as therapeutic agents
As we face increasing challenges from infectious diseases, the potential of AMPs to combat MDR and biofilm-related infections is a beacon of hope. However, their journey from the laboratory to clinical application is riddled with challenges that must be overcome before AMPs can fully realize their potential in the market.
As of January 2024, 3940 AMPs are listed in antimicrobial peptide database, APD3, reflecting considerable diversity and potential within this class of molecules. Yet, only <10 AMPs, including Colistin, Nisin, Gramicidin, Polymyxin, and Daptomycin, have been approved by the FDA for clinical use (Chen and Lu 2020); many more AMPs are, however, currently at different stages of clinical and preclinical evaluation (Mazurkiewicz-Pisarek et al. 2023). For instance, a clinical trial (NCT02225366, accessed via https://clinicaltrials.gov) explored the use of intra-tumoral LL-37 injections for melanoma treatment and reported it as safe for human use (Grönberg et al. 2014). In another clinical trial, the antiviral activity of AMPs against the vaccinia virus was investigated (NCT00407069) (Yang et al. 2023). Another ongoing clinical trial (NCT05530252) is focusing on assessing the effects of different AMPs on stage III Grade B periodontitis (Xiang et al. 2024). However, further clinical studies are needed to assess their safety and efficacy more comprehensively.
Some clinical trials involving AMPs have been discontinued or terminated for various reasons. For instance, the Phase I clinical trial of Friulimicin B in healthy volunteers was halted reportedly due to its unfavourable pharmacokinetic profile. Another AMP, murepavadin (POL7080), unexpectedly failed in advanced clinical trials. However, it was reported to be safe in Phase I clinical trials (NCT03409679) involving both healthy volunteers and subjects with impaired renal function. Additionally, Phase II trials (NCT03582007) demonstrated the safety and efficacy of murepavadin in patients with acute exacerbation of non-CF bronchiectasis or ventilator-associated bacterial pneumonia caused by P. aeruginosa. However, the Phase III clinical trial (NCT03409679) in patients with nosocomial pneumonia was prematurely terminated due to an unexpectedly high incidence of acute kidney injuries—56% in the murepavadin plus ertapenem treatment group, compared to 25%–40% in the meropenem-treated control group (Elmassry et al. 2023).
However, the road to regulatory approval is paved with hurdles that demand careful examinations and substantial resources. From preclinical trials to clinical validation, each step in the approval process examines the safety, efficacy, and pharmacokinetic profile of AMPs. Despite their inherent antimicrobial activity, many candidates fail at different stages of preclinical and clinical trials due to concerns surrounding cytotoxicity, stability, and pharmacokinetic parameters (Dijksteel et al. 2021). Overcoming these challenges needs a multifactorial approach, including innovative drug design, advanced formulation strategies, and robust preclinical evaluation methodologies. Strategies to mitigate cytotoxicity while enhancing selectivity towards microbial targets are imperative for optimising the therapeutic index of AMPs. Moreover, innovative delivery systems and formulation technologies are critical for improving the stability and bioavailability of AMPs, thereby enhancing their therapeutic efficacy. Designing conjugates with cleavable linkers represents a promising approach in this regard. These linkers can be engineered to cleave specifically at the target site, minimizing off-target effects and reducing systemic toxicity and allergic reactions (Boyce et al. 2020, Bellucci et al. 2024). Additionally, cleavable linkers can enhance the stability of AMPs and further mitigate cytotoxicity by lowering the required therapeutic dose, thereby maximizing their clinical potential (Cui et al. 2021).
Alongside these scientific endeavours, substantial investment in research and development are required to facilitate the translation of AMPs from bench to bedside. Collaborative efforts between academia, industry, and regulatory agencies are needed to streamline the regulatory pathways and accelerate the approval process for AMP-based therapeutics.
Looking ahead, the future of AMPs as therapeutic agents holds immense promise, driven by advancements in peptide engineering, formulation science, and regulatory facilitation. With concerted efforts and solid commitment, AMPs are poised to emerge as useful drugs against MDR and biofilm-associated infections, perhaps heralding a new era in antimicrobial therapy.
Alternative strategies: the potential of self-assembling peptides in combating biofilm-related infections
Amidst the complexities surrounding the use of antibiotics, AMPs, and AMP-antibiotic conjugates in combating biofilm-related infections, there is a gradual pivot towards exploring alternative strategies to address this urgent challenge. While ongoing research on these treatment modalities persists, alternative plans are also being explored. Notably, there has been an increasing interest in the antimicrobial potential of self-assembling peptides in recent years. Self-assembling peptides are short sequences of amino acids that have the intrinsic ability to spontaneously organize into well-defined structures or supramolecular assemblies through non-covalent interactions such as hydrogen bonding, hydrophobic interactions, electrostatic forces, and π-π stacking, which will lead to forming nanostructures such as nanofibres, tubes, micelles, or hydrogels under certain circumstances (Marchesan et al. 2013, Adak et al. 2024, Soliman et al. 2025). Some self-assembling peptides exhibited antibacterial and antibiofilm activities (Porter et al. 2018, Castelletto et al. 2020, Adak et al. 2024). This emerging field holds promise for developing innovative treatments for biofilm-related infections, potentially revolutionizing the approach to managing these persistent and challenging conditions. The amphiphilic nature of these nanostructures can be used to insert hydrophobic and hydrophilic antimicrobial agents into their structure to release them at the target site (Marchesan et al. 2013, Hansda et al. 2023). Moreover, due to their viscosity, self-assembling peptides can be used to treat chronic wounds or as a coating for medical equipment, such as catheters, effectively preventing biofilm formation without being easily washed away (Salick et al. 2009).
Various studies have demonstrated antibacterial and antibiofilm activity of Diphenylalanine (FF) peptide nanotubes. FF peptide nanotubes showed antibacterial and biofilm eradication activity against S. aureus and bactericidal activity against E. coli. Nanotubes are formed through intermolecular interactions between neighbouring phenyl groups, hydrophobic interactions, and hydrogen bonding with the solvent (Schnaider et al. 2017, Porter et al. 2018). Cardoso and colleagues additionally focused on two ultrashort, self-assembling peptides: WWRR-NH2, termed priscilicidin, and WPWRR-NH2, termed P-Priscilicidin (Cardoso et al. 2023). These two peptides demonstrated strong antifungal and antibacterial activity against Gram-positive bacteria, while also showing some effectiveness against Gram-negative bacteria although antibiofilm activity was not reported. The amino acid composition of Priscilicidin includes two aromatic residues (tryptophan) and two positively charged amino acids (arginine). The self-assembly of Priscilicidin into extended chains is stabilized by continuous intermolecular π-stacking of the aromatic and hydrophobic Fmoc groups in the central core. Additionally, the arginine side chains embedded within the hydrophobic core contribute to the structural organization and self-assembling properties of the peptide.
P-Priscilicidin is a modified version of Priscilicidin, where an additional proline residue is inserted between the two tryptophan residues. Proline is a unique amino acid that introduces a kink or turn in the peptide chain due to its cyclic structure. This modification is expected to disrupt the supramolecular assembly of the peptide into nanofibrils while retaining its antimicrobial activity.
The exploration of self-assembling peptides presents a promising avenue for innovative treatments against biofilm-related infections. With their unique ability to form nanostructures and demonstrated antibacterial and antibiofilm activities, these peptides could revolutionize current therapeutic approaches, offering effective solutions where traditional treatments fall short.
Despite their potential as therapeutic agents, self-assembling peptides require further research before they can be commercially used. Some self-assembling peptides exhibit cytotoxicity, haemolytic activity, limited spectrum of activity, or low antimicrobial and antibiofilm properties (Lombardi et al. 2019). Additionally, the self-assembling process occurs under specific physical and chemical conditions, which may differ within the body, potentially compromising the stability of the nanostructures. These issues must be thoroughly addressed before clinical application (Castelletto et al. 2020).
Conclusion and suggestions
AMR infections have emerged as a formidable global threat in recent years, imperilling decades of progress in combating infectious diseases (Murray et al. 2022). Biofilm-related infections are an additional challenge, being associated with substantuial proportion of bacterial infections globally (Jamal et al. 2018). While AMPs represent a promising avenue in the fight against multidrug-resistant infections, several barriers must be addressed to optimize their clinical application. Future research should focus on several key strategiesThe following is a non-exhaustive list:
Enhancing stability and bioavailability: The design and synthesis of modified AMPs that demonstrate increased stability in physiological environments is an important objective. Techniques such as cyclization or incorporating d-amino acids may improve resistance to enzymatic degradation, thereby extending their therapeutic window. However, challenges such as higher production costs associated with d-amino acids must be carefully considered before pursuing this approach (Jia et al. 2017).
Reducing cytotoxicity: Conducting detailed studies on the structure–activity relationship of AMPs can lead to the design of peptides with selective antibacterial properties that minimize damage to human cells. Exploring delivery systems, such as liposomes or nanoparticles, may also help target AMPs specifically to infected tissues (Nyembe et al. 2023).
Targeted delivery and formulation: Developing advanced drug delivery systems that enable localized delivery of AMPs may enhance their therapeutic potential. Exploring formulations that leverage smart materials or controlled release mechanisms can be beneficial in ensuring sustained exposure to pathogens.
Synergistic combinations: One promising modification involves conjugating or combining AMPs with other antimicrobial agents, such as antibiotics. This strategy offers potential benefits, including enhanced activity through synergistic effects and improved stability via covalent bond formation. Moreover, conjugation with antibiotics can mitigate the toxicity associated with AMPs. Further research is needed to evaluate the efficacy of such conjugates and identify effective combinations that can improve outcomes against a range of resistant pathogens (Fair and Tor 2014, Mohamed et al. 2017, Mahesh et al. 2018).
Several challenges persist in adopting AMP-antibiotic conjugates. These include high production costs, compatibility issues, and the inactivity of specific conjugates (Ghaffar et al. 2015, Chaudhary et al. 2023). As a result, researchers are actively investigating novel alternatives, such as ultrashort self-assembling peptides. These peptides exhibit antimicrobial and antibiofilm activities and offer improved stability over traditional AMPs. Notably, ultrashort peptides possess self-assembly properties. These nanostructures enhance their efficacy by preventing easy washout. This distinctive property makes them suitable for applications such as coating medical equipment or treating chronic wounds. Combining these peptides with modified AMPs presents a promising avenue for further improvement (Wychowaniec et al. 2020).
Although using ultrashort self-assembling peptides can partially solve some problems, such as the cost of production due to their small size, they also present certain challenges. For instance, their stability and solubility can vary in different physiological conditions, which may affect their performance in specific applications. Additionally, the limited structural diversity of ultrashort peptides may restrict their functional capabilities compared to longer, more complex peptides. There are several avenues for further improving ultrashort peptides.
Chemical modifications: Altering the amino acid sequence or incorporating non-standard amino acids can enhance stability and efficacy. Modifications such as methylation or acylation can also improve their properties (Wychowaniec et al. 2020). Also, exploring different structural variants of these peptides may reveal candidates with superior properties, such as enhanced surface adhesion or improved biocompatibility.
Hybridization with other molecules: Combining ultrashort self-assembling peptides with AMPs or other bioactive agents can create synergistic effects, enhancing their biological activity and broadening their applications (Huo et al. 2023).
Targeting and delivery systems: developing targeted delivery systems, such as nanoparticles or liposomes, can help ensure that the ultrashort peptides reach their intended site of action more effectively (Das and Gavel 2020).
Further research is essential to validate the hypotheses surrounding both AMPs and ultrashort self-assembling peptides, as well as to evaluate their efficacy and safety in diverse applications. This involves conducting extensive in vitro and in vivo studies to understand the mechanisms of action, optimal dosing, and long-term effects of these peptides. Establishing regulatory frameworks and collaborative efforts among researchers, clinicians, and industry stakeholders will be crucial to ensure compliance and safety for potential therapeutic applications. Ultimately, a thorough investigation of both AMPs and ultrashort self-assembling peptides can lead to innovative solutions in areas such as drug delivery, infection management, and tissue regeneration, significantly impacting public health.
Author contributions
Navid Dad (Conceptualization, Formal analysis, Investigation, Methodology, Writing – original draft, Writing – review & editing), Mohamed A. Elsawy (Formal analysis, Investigation, Writing – original draft, Writing – review & editing), Gavin Humphreys (Supervision, Writing – original draft, Writing – review & editing), Alain Pluen (Conceptualization, Supervision, Writing – original draft, Writing – review & editing), Jian R. Lu (Formal analysis, Methodology, Writing – review & editing), and Andrew J. McBain (Conceptualization, Formal analysis, Funding acquisition, Methodology, Project administration, Resources, Supervision, Writing – original draft, Writing – review & editing)
Conflict of interest
None declared.
Funding
N.D. receives funding for graduate studies through a personal grant.
Data availability
This article is a review of existing literature.