-
PDF
- Split View
-
Views
-
Cite
Cite
Caoimhe Doyle, Katie Wall, Séamus Fanning, Barry J McMahon, Making sense of sentinels: wildlife as the One Health bridge for environmental antimicrobial resistance surveillance, Journal of Applied Microbiology, Volume 136, Issue 1, January 2025, lxaf017, https://doi.org/10.1093/jambio/lxaf017
- Share Icon Share
Abstract
Antimicrobial resistance (AMR), arising from decades of imprudent anthropogenic use of antimicrobials in healthcare and agriculture, is considered one of the greatest One Health crises facing healthcare globally. Antimicrobial pollutants released from human-associated sources are intensifying resistance evolution in the environment. Due to various ecological factors, wildlife interact with these polluted ecosystems, acquiring resistant bacteria and genes. Although wildlife are recognized reservoirs and disseminators of AMR in the environment, current AMR surveillance systems still primarily focus on clinical and agricultural settings, neglecting this environmental dimension. Wildlife can serve as valuable sentinels of AMR in the environment, reflecting ecosystem health, and the effectiveness of mitigation strategies. This review explores knowledge gaps surrounding the ecological factors influencing AMR acquisition and dissemination in wildlife, and highlights limitations in current surveillance systems and policy instruments that do not sufficiently address the environmental component of AMR. We discuss the underutilized opportunity of using wildlife as sentinel species in a holistic, One Health-centred AMR surveillance system. By better integrating wildlife into systematic AMR surveillance and policy, and leveraging advances in high-throughput technologies, we can track and predict resistance evolution, assess the ecological impacts, and better understand the complex dynamics of environmental transmission of AMR across ecosystems.
This review highlights the potential role of wildlife as sentinel species for antimicrobial resistance (AMR) surveillance and outlines the limitations of current systems. By leveraging ecological knowledge and existing monitoring frameworks, this review offers practical suggestions to inform novel and sustainable research and policy shifts that safeguard wildlife conservation, and public and environmental health under the One Health paradigm.
Introduction
Antimicrobials are considered the most remarkable discovery of the 20th century. They have contributed enormously in settings from clinical treatment of infections in humans and animals to prophylactic use in agriculture, aquaculture, and food preservation (Serwecińska 2020, Salam et al. 2023). However, the imprudent use of antimicrobials, alongside insufficient infection prevention and control measures, have resulted in the emergence and spread of antimicrobial resistance (AMR), causing a multi-faceted global challenge known as a ‘silent pandemic’ (Mitchell 2023, WHO 2023). AMR occurs when bacteria become less susceptible to an antimicrobial compound via the overexpression or duplication of certain genes, chromosomal mutations, or by obtaining resistance genes from other bacteria by horizontal gene transfer (HGT). AMR is now recognized as one of the leading threats facing public and animal healthcare worldwide, hindering the efficacy of common antimicrobial treatments (CDC 2024). It is estimated to be responsible for 35 000 human deaths in the European Union/European Economic Area (EU/EEA) annually (ECDC 2024). In 2019 alone, AMR infections were associated with an estimated 4.95 million deaths globally (Murray et al. 2022). This number is estimated to increase to 10 million per year by 2050 (O’Neill 2016). Regarding the economic impact, it has been estimated that AMR will result in a global cost of 100 trillion USD by 2050. In the EU/EEA, it currently results in extra healthcare expenditure of ∼€1.1 billion per annum due to the additional costs for second- and third-line antimicrobials required for resistant infections, and the longer duration of disease or hospital stays (OECD 2019).
Antimicrobials used in veterinary medicine and agricultural practice are often the same or are of the same classes as those used in human medicine (EFSA and ECDC 2021). This includes those listed by the World Health Organization as ‘critically important’ for humans (WHO 2015, 2019). This overlap raises concern that extensive antimicrobial usage with one antimicrobial in one population could contribute to the development of resistance in another population to an entire related class of antimicrobials (Chantziaras et al. 2014, Tang et al. 2017). In turn, this increased resistance developing in both cohorts, can be returned back to the environment by, e.g. sewage, hospital and pharmaceutical wastewater, or slurry spreading. Whilst the inclination exists amongst stakeholders to compartmentalize this environmental contamination, it would be more appropriate to consider all elements in all environmental compartments in the system, together with their interactions and feedback loops (Arnold et al. 2024), as the strategies and policies enacted in one sector can affect other interconnected sectors (Rahman and Hollis 2023). Hence, a systems-thinking, One Health (OH) approach is crucial to address the situation. With the concept of OH comes an integrative approach that recognizes the necessity for interdisciplinary collaboration to provide sustainable solutions to health challenges, acknowledging the inextricable link between the health of humans, domestic and wild animals, plants, and ecosystems (McEwen and Collignon 2018, Adisasmito et al. 2022, Ahmad et al. 2023). In recent years, a broader reformulation of the classic OH paradigm has emerged, continuing to recognize the interconnectedness of human, animal, and environmental health, while also emphasizing the important role of geographical proximity of ecosystems in the emergence and dissemination of traits of ecosystem health importance (Baquero et al. 2019). AMR is the quintessential OH issue (Robinson et al. 2016). As such, it is crucial that we acknowledge it as an inter-ecosystem problem—a microbiological issue with macroecological causes and consequences that must be viewed through the OH lens.
Given the anthropocentric view we have had in the past, human health and domestic animal health have been considered the most important focus of the AMR crisis. Unsurprisingly, the relationship between the two hosts is relatively well-documented within the context of transmission of zoonoses and antimicrobial resistant bacteria (ARB) (Manyi-Loh et al. 2018, Hayek 2022, Rahman and Hollis 2023). In fact, although AMR is considered one of the greatest threats facing global health security, most AMR research has been based in clinical and agricultural settings (Arnold et al. 2016). Thankfully, however, over the past decade, the environment has gained increasing recognition for its part in the AMR cycle. Given a bacterium’s propensity to move past geographical and species boundaries, the environment must play a role in the evolution and dissemination of resistant microbes (Arnold et al. 2016, Robinson et al. 2016). Although considerable efforts have been made to enhance the understanding of AMR dynamics in humans and domestic animals, wildlife have been given far less attention and remain an overlooked environmental cohort in the AMR transmission cycle (Vittecoq et al. 2016a, Li et al. 2024).
AMR in Wildlife
Wildlife are considered reservoirs, disseminators and even potential melting pots of AMR, contributing to its evolution and transmission across different ecosystems (Guenther et al. 2011, Vittecoq et al. 2016a). Resistant bacteria have been detected across a diverse array of wild animal species, including rodents (Gilliver et al. 1999, Uea-Anuwong et al. 2023), wild birds such as gulls (Carroll et al. 2015) and Passeriformes (Santos et al. 2013), felids such as the Iberian lynx (Lynx pardinus) (Goncalves et al. 2013b), jaguars (Panthera onca) (Cristobal-Azkarate et al. 2014), and canids such as red foxes (Vulpes vulpes) (Radhouani et al. 2012) and Iberian wolves (Canis lupus signatus) (Goncalves et al. 2013a). Granted, the detection of AMR in wild animals could represent natural selection (D’Costa et al. 2011); however, when clinically-relevant antimicrobial resistance genes (ARGs) and ARB are found in untreated, antimicrobial ‘naïve’ wild animals, it is considered a marker of environmental AMR pollution, originating from human or domestic animal waste, rather than natural selection (Martinez et al. 2009, Laborda et al. 2022).
Whilst these records of AMR carriage exist, evidence in the literature regarding longitudinal maintenance in wildlife is scarce, and defining the source and direction of dissemination remains unclear or contradictory at best (Arnold et al. 2016, Vittecoq et al. 2016a, Swift et al. 2019). Although studies are starting to discern the profiles of commensal bacteria and zoonotic pathogens most frequently found in wildlife, and the possible resistance determinants possessed, data are still lacking regarding the holistic picture. In 2017, the WHO published a list of AMR-harbouring pathogens of priority. This list included, among others, carbapenem-resistant Acinetobacter baumannii, Pseudomonas aeruginosa, carbapenem-resistant and extended-spectrum β-lactamase (ESBL)-producing Enterobacteriaceae, fluoroquinolone-resistant Salmonella and Campylobacter, vancomycin-resistant Enterococcus faecium, and methicillin-resistant and vancomycin-intermediate/resistant Staphylococcus aureus (WHO 2017, Li et al. 2024). With this list in mind, it is essential to highlight that the majority of primary research into AMR in wildlife focuses their attention on specific bacterial indicator species (Greig et al. 2015) leaving a need for a comprehensive understanding of the broader environmental context. Escherichia coli is the most frequently targeted bacterial indicator species in AMR investigations in wildlife (Greig et al. 2015, Vittecoq et al. 2016a). It is used to monitor environmental ARGs and resistance elements to clinically-relevant antimicrobials such as extended spectrum β-lactams, carbapenems, and colistin (Anjum et al. 2021). ESBL-producing E. coli, having been first reported in wildlife in Portugal in 2006 (Costa et al. 2006), is now regularly identified in many wildlife species (Guenther et al. 2011) with prevalence ranging 1.7%–54.0% (Li et al. 2024). The most prevalent ESBLs belong to the CTX-M family, of which CTX-M-1, CTX-M-14, and CTX-M-15, alongside TEM and SHV, and CMY-2 in AmpC-producers, and are frequently identified in wild animals (Palmeira et al. 2021, Li et al. 2024). Many carbapenemase-encoding genes have also been detected in wildlife, including blaKPC-2, blaKPC-3, blaNDM-1, blaNDM-5, blaOXA-48, blaOXA-245, and blaVIM-1 (Ahlstrom et al. 2022, Loucif et al. 2022). With regard to colistin-resistance, the mcr-1 and mcr-2 genes are increasingly being identified in wildlife species (Ahmed et al. 2019, Torres et al. 2021, Loucif et al. 2022). These findings are of considerable importance from a public health perspective as these genes confer resistance to antimicrobials categorized as ‘High Priority Critically Important’ or ‘Highest Priority Critically Important’ by the WHO, which are antimicrobials that must be preserved for human healthcare, by reducing their use in the non-human sectors (WHO 2019). For instance, colistin is a polymyxin, which is classed as Highest Priority, and is considered a drug of last resort in human medicine and is usually reserved to treat multi-drug resistant (MDR) bacteria like E. coli.
To date, AMR investigations in wildlife have faced a number of challenges that may hinder the external comparability of the results. These challenges include relatively small sample sizes, the use of point-prevalence and convenience sampling leading to decreased spatial or temporal representation, variation in culture, isolation, and resistance detection methods used, and the heterogeneity of the data reported (Vittecoq et al. 2016a, Li et al. 2024). Therefore, not only is research into wildlife AMR transmission lacking, but the discussion and interpretation of the results we do have are plagued by systematic bias (Li et al. 2024). Additionally, the dynamics of AMR in wildlife remain understudied, including the determining factors for acquiring and transmitting ARB and ARGs, alongside the length of time the animal will harbour and shed ARB in the environment. AMR presence in wildlife has not been observed uniformly amongst species (Torres et al. 2020b), implying that various ecological traits predispose some species to harbouring or disseminating AMR more efficiently (see Table 1) (Allen et al. 2010). This may involve aspects of their feeding strategies and foraging behaviour, social structures and behaviours, habitat usage and environmental interactions, their movement and migration patterns, alongside their proximity to human activity.
The ecological factors that may influence a wild animal species’ propensity to acquire and harbour AMR, and the current knowledge lacking in the literature.
Ecological factor . | Characteristic . | Exposure and acquisition risk . | Current knowledge gaps and needs . |
---|---|---|---|
Feeding strategies and foraging behaviour | Nutrition-type | ● AMR profiles are closely tied to feeding strategies. ● Higher frequency of AMR has been found in gut microbiota of carnivores and omnivores than in herbivores. ● Carnivores are likely more exposed to ARB by consumption of contaminated prey species, and omnivorous species are exposed to a wide variety of ARB, both agricultural and anthropogenic. | There is a dearth of information regarding how nutrition-type specifically influences AMR profiles in wildlife. Detailed investigations into the impact of specific diet-types and feeding patterns on resistance determinants and gene acquisition, alongside the shedding ARB across ecosystems are lacking, which also poses public health concerns with regards to urbanized carnivores. |
Predation and scavenging | ● Predation on peridomestic prey species that may already harbour AMR, could potentiate resistance through trophic levels. ● Predation habits expose species to a wide range of ecosystems, broadening acquisition potential. ● Foraging behaviour is often in proximity to human activity, commonly in refuse and landfills. ● Obligate and facultative scavengers feeding on medicated livestock carcasses or carrion risk exposure to high levels of ARB. ● This behaviour could exert selective pressures on gut microbiota, promoting development and dissemination of novel ARGs. | ● The influence of feeding and hunting strategies on the acquisition of AMR is poorly understood. The question of how scavenging behaviours differ in their contributions to ARB exposure compared to active predation, particularly on live, peridomestic prey is not well-answered. ● Studies could focus on the functional roles of different gut microbes acquired from anthropogenic sources vs those acquired from rural settings such as in proximity to agricultural landscapes, within the context of ARB acquisition, particularly focusing on high-risk clonal lineages that may be more likely acquired from one source over another. | |
Social behaviour | Aggression and territoriality in disease spread | ● The relationships between wildlife behaviour, social systems, and disease transmission mechanisms between territories and individuals, especially in high-contact environments are acknowledged. ● Aggressive interactions and territorial behaviours (such as biting and excrement-marking) of species living or feeding in competitive environments can facilitate disease transmission. | ● No studies currently exist to determine if or how these aggressive or territorial behaviours may be a risk factor to facilitate AMR acquisition and dissemination in the environment. ● Environmental, longitudinal point-prevalence survey studies examining the correlation between territorial behaviours and AMR presence could be informative. ● Aggressive pack animals such as the Tasmanian Devil, could also be assessed for ARB upon disease outbreaks in the environment in comparison to those in captivity. |
The ‘Social Microbiome’ | ● Social species living in close proximity and engaging in behaviours like grooming and communal feeding or nesting exhibit similar microbiomes. ● The ‘social microbiome’ suggests that social behaviour may influence the composition of gut microbiota, potentially increasing bacterial exchange and HGT, increasing the likelihood of AMR transmission within groups via faecal contamination. | Effects of the ‘social microbiome’ in HGT leading to transmission of ARB and ARGs remain under-explored. Research must emphasize ecological dynamics of social interactions, in order to determine inter- and intra-species interactions. Further studies are needed to assess how contact within groups and between species may influence microbial community compositions and facilitate bacterial and gene exchange. | |
Movement and migration | Epidemiological link between habitats | ● Movement of wildlife has been identified as a driver of AMR dissemination in the environment, enabling species to serve as epidemiological links between habitats. ● Wildlife may be transmitting AMR between agricultural, urban, and natural environments by daily movement between these habitats, and contaminating with faeces or direct contact with domestic animals. | ● There is a lack of data surrounding AMR transmission dynamics across ecosystems due to wildlife movement and how these dynamics vary across different species and habitat. There is a need for deeper exploration into how human-induced habitat alteration (e.g. urbanization and intensification of agricultural practices) may be impacting movement patterns and consequently AMR spread. ● Limited information exists regarding reintroduction or translocation of wild animals following antimicrobial treatments in the context of conservation or in cases of wildlife rehabilitation. Research is lacking regarding whether this facilitates the spread of novel ARGs in surrounding wildlife. |
Intercontinental vectors | ● Wildlife species can act as vectors of ARB, carrying it over long distances during migration, through their excreta, facilitating intercontinental spread of AMR. ● Human-associated resistance has been detected in wildlife in very remote regions of the world, implying that migratory wildlife may play a role in AMR transmission, not just to other environments but to other wildlife species in those regions. | ● Most existing research relies on cross-sectional ‘snapshots’ on the presence of AMR in migratory wildlife, which fails to capture long-term trends. Limited longitudinal studies exist that monitor AMR in wildlife throughout migration to understand persistence and transmission dynamics, particularly with regards to external factors such as climate change that may alter migration pathways. ● More comprehensive studies are also required understand the genetic diversity and strain types of ARB acquired and disseminated by wildlife vectors, alongside the extent and duration of ARB shedding. |
Ecological factor . | Characteristic . | Exposure and acquisition risk . | Current knowledge gaps and needs . |
---|---|---|---|
Feeding strategies and foraging behaviour | Nutrition-type | ● AMR profiles are closely tied to feeding strategies. ● Higher frequency of AMR has been found in gut microbiota of carnivores and omnivores than in herbivores. ● Carnivores are likely more exposed to ARB by consumption of contaminated prey species, and omnivorous species are exposed to a wide variety of ARB, both agricultural and anthropogenic. | There is a dearth of information regarding how nutrition-type specifically influences AMR profiles in wildlife. Detailed investigations into the impact of specific diet-types and feeding patterns on resistance determinants and gene acquisition, alongside the shedding ARB across ecosystems are lacking, which also poses public health concerns with regards to urbanized carnivores. |
Predation and scavenging | ● Predation on peridomestic prey species that may already harbour AMR, could potentiate resistance through trophic levels. ● Predation habits expose species to a wide range of ecosystems, broadening acquisition potential. ● Foraging behaviour is often in proximity to human activity, commonly in refuse and landfills. ● Obligate and facultative scavengers feeding on medicated livestock carcasses or carrion risk exposure to high levels of ARB. ● This behaviour could exert selective pressures on gut microbiota, promoting development and dissemination of novel ARGs. | ● The influence of feeding and hunting strategies on the acquisition of AMR is poorly understood. The question of how scavenging behaviours differ in their contributions to ARB exposure compared to active predation, particularly on live, peridomestic prey is not well-answered. ● Studies could focus on the functional roles of different gut microbes acquired from anthropogenic sources vs those acquired from rural settings such as in proximity to agricultural landscapes, within the context of ARB acquisition, particularly focusing on high-risk clonal lineages that may be more likely acquired from one source over another. | |
Social behaviour | Aggression and territoriality in disease spread | ● The relationships between wildlife behaviour, social systems, and disease transmission mechanisms between territories and individuals, especially in high-contact environments are acknowledged. ● Aggressive interactions and territorial behaviours (such as biting and excrement-marking) of species living or feeding in competitive environments can facilitate disease transmission. | ● No studies currently exist to determine if or how these aggressive or territorial behaviours may be a risk factor to facilitate AMR acquisition and dissemination in the environment. ● Environmental, longitudinal point-prevalence survey studies examining the correlation between territorial behaviours and AMR presence could be informative. ● Aggressive pack animals such as the Tasmanian Devil, could also be assessed for ARB upon disease outbreaks in the environment in comparison to those in captivity. |
The ‘Social Microbiome’ | ● Social species living in close proximity and engaging in behaviours like grooming and communal feeding or nesting exhibit similar microbiomes. ● The ‘social microbiome’ suggests that social behaviour may influence the composition of gut microbiota, potentially increasing bacterial exchange and HGT, increasing the likelihood of AMR transmission within groups via faecal contamination. | Effects of the ‘social microbiome’ in HGT leading to transmission of ARB and ARGs remain under-explored. Research must emphasize ecological dynamics of social interactions, in order to determine inter- and intra-species interactions. Further studies are needed to assess how contact within groups and between species may influence microbial community compositions and facilitate bacterial and gene exchange. | |
Movement and migration | Epidemiological link between habitats | ● Movement of wildlife has been identified as a driver of AMR dissemination in the environment, enabling species to serve as epidemiological links between habitats. ● Wildlife may be transmitting AMR between agricultural, urban, and natural environments by daily movement between these habitats, and contaminating with faeces or direct contact with domestic animals. | ● There is a lack of data surrounding AMR transmission dynamics across ecosystems due to wildlife movement and how these dynamics vary across different species and habitat. There is a need for deeper exploration into how human-induced habitat alteration (e.g. urbanization and intensification of agricultural practices) may be impacting movement patterns and consequently AMR spread. ● Limited information exists regarding reintroduction or translocation of wild animals following antimicrobial treatments in the context of conservation or in cases of wildlife rehabilitation. Research is lacking regarding whether this facilitates the spread of novel ARGs in surrounding wildlife. |
Intercontinental vectors | ● Wildlife species can act as vectors of ARB, carrying it over long distances during migration, through their excreta, facilitating intercontinental spread of AMR. ● Human-associated resistance has been detected in wildlife in very remote regions of the world, implying that migratory wildlife may play a role in AMR transmission, not just to other environments but to other wildlife species in those regions. | ● Most existing research relies on cross-sectional ‘snapshots’ on the presence of AMR in migratory wildlife, which fails to capture long-term trends. Limited longitudinal studies exist that monitor AMR in wildlife throughout migration to understand persistence and transmission dynamics, particularly with regards to external factors such as climate change that may alter migration pathways. ● More comprehensive studies are also required understand the genetic diversity and strain types of ARB acquired and disseminated by wildlife vectors, alongside the extent and duration of ARB shedding. |
AMR, antimicrobial resistance; ARB, antimicrobial resistant bacteria; ARG, antimicrobial resistance gene; and HGT, horizontal gene transfer.
The ecological factors that may influence a wild animal species’ propensity to acquire and harbour AMR, and the current knowledge lacking in the literature.
Ecological factor . | Characteristic . | Exposure and acquisition risk . | Current knowledge gaps and needs . |
---|---|---|---|
Feeding strategies and foraging behaviour | Nutrition-type | ● AMR profiles are closely tied to feeding strategies. ● Higher frequency of AMR has been found in gut microbiota of carnivores and omnivores than in herbivores. ● Carnivores are likely more exposed to ARB by consumption of contaminated prey species, and omnivorous species are exposed to a wide variety of ARB, both agricultural and anthropogenic. | There is a dearth of information regarding how nutrition-type specifically influences AMR profiles in wildlife. Detailed investigations into the impact of specific diet-types and feeding patterns on resistance determinants and gene acquisition, alongside the shedding ARB across ecosystems are lacking, which also poses public health concerns with regards to urbanized carnivores. |
Predation and scavenging | ● Predation on peridomestic prey species that may already harbour AMR, could potentiate resistance through trophic levels. ● Predation habits expose species to a wide range of ecosystems, broadening acquisition potential. ● Foraging behaviour is often in proximity to human activity, commonly in refuse and landfills. ● Obligate and facultative scavengers feeding on medicated livestock carcasses or carrion risk exposure to high levels of ARB. ● This behaviour could exert selective pressures on gut microbiota, promoting development and dissemination of novel ARGs. | ● The influence of feeding and hunting strategies on the acquisition of AMR is poorly understood. The question of how scavenging behaviours differ in their contributions to ARB exposure compared to active predation, particularly on live, peridomestic prey is not well-answered. ● Studies could focus on the functional roles of different gut microbes acquired from anthropogenic sources vs those acquired from rural settings such as in proximity to agricultural landscapes, within the context of ARB acquisition, particularly focusing on high-risk clonal lineages that may be more likely acquired from one source over another. | |
Social behaviour | Aggression and territoriality in disease spread | ● The relationships between wildlife behaviour, social systems, and disease transmission mechanisms between territories and individuals, especially in high-contact environments are acknowledged. ● Aggressive interactions and territorial behaviours (such as biting and excrement-marking) of species living or feeding in competitive environments can facilitate disease transmission. | ● No studies currently exist to determine if or how these aggressive or territorial behaviours may be a risk factor to facilitate AMR acquisition and dissemination in the environment. ● Environmental, longitudinal point-prevalence survey studies examining the correlation between territorial behaviours and AMR presence could be informative. ● Aggressive pack animals such as the Tasmanian Devil, could also be assessed for ARB upon disease outbreaks in the environment in comparison to those in captivity. |
The ‘Social Microbiome’ | ● Social species living in close proximity and engaging in behaviours like grooming and communal feeding or nesting exhibit similar microbiomes. ● The ‘social microbiome’ suggests that social behaviour may influence the composition of gut microbiota, potentially increasing bacterial exchange and HGT, increasing the likelihood of AMR transmission within groups via faecal contamination. | Effects of the ‘social microbiome’ in HGT leading to transmission of ARB and ARGs remain under-explored. Research must emphasize ecological dynamics of social interactions, in order to determine inter- and intra-species interactions. Further studies are needed to assess how contact within groups and between species may influence microbial community compositions and facilitate bacterial and gene exchange. | |
Movement and migration | Epidemiological link between habitats | ● Movement of wildlife has been identified as a driver of AMR dissemination in the environment, enabling species to serve as epidemiological links between habitats. ● Wildlife may be transmitting AMR between agricultural, urban, and natural environments by daily movement between these habitats, and contaminating with faeces or direct contact with domestic animals. | ● There is a lack of data surrounding AMR transmission dynamics across ecosystems due to wildlife movement and how these dynamics vary across different species and habitat. There is a need for deeper exploration into how human-induced habitat alteration (e.g. urbanization and intensification of agricultural practices) may be impacting movement patterns and consequently AMR spread. ● Limited information exists regarding reintroduction or translocation of wild animals following antimicrobial treatments in the context of conservation or in cases of wildlife rehabilitation. Research is lacking regarding whether this facilitates the spread of novel ARGs in surrounding wildlife. |
Intercontinental vectors | ● Wildlife species can act as vectors of ARB, carrying it over long distances during migration, through their excreta, facilitating intercontinental spread of AMR. ● Human-associated resistance has been detected in wildlife in very remote regions of the world, implying that migratory wildlife may play a role in AMR transmission, not just to other environments but to other wildlife species in those regions. | ● Most existing research relies on cross-sectional ‘snapshots’ on the presence of AMR in migratory wildlife, which fails to capture long-term trends. Limited longitudinal studies exist that monitor AMR in wildlife throughout migration to understand persistence and transmission dynamics, particularly with regards to external factors such as climate change that may alter migration pathways. ● More comprehensive studies are also required understand the genetic diversity and strain types of ARB acquired and disseminated by wildlife vectors, alongside the extent and duration of ARB shedding. |
Ecological factor . | Characteristic . | Exposure and acquisition risk . | Current knowledge gaps and needs . |
---|---|---|---|
Feeding strategies and foraging behaviour | Nutrition-type | ● AMR profiles are closely tied to feeding strategies. ● Higher frequency of AMR has been found in gut microbiota of carnivores and omnivores than in herbivores. ● Carnivores are likely more exposed to ARB by consumption of contaminated prey species, and omnivorous species are exposed to a wide variety of ARB, both agricultural and anthropogenic. | There is a dearth of information regarding how nutrition-type specifically influences AMR profiles in wildlife. Detailed investigations into the impact of specific diet-types and feeding patterns on resistance determinants and gene acquisition, alongside the shedding ARB across ecosystems are lacking, which also poses public health concerns with regards to urbanized carnivores. |
Predation and scavenging | ● Predation on peridomestic prey species that may already harbour AMR, could potentiate resistance through trophic levels. ● Predation habits expose species to a wide range of ecosystems, broadening acquisition potential. ● Foraging behaviour is often in proximity to human activity, commonly in refuse and landfills. ● Obligate and facultative scavengers feeding on medicated livestock carcasses or carrion risk exposure to high levels of ARB. ● This behaviour could exert selective pressures on gut microbiota, promoting development and dissemination of novel ARGs. | ● The influence of feeding and hunting strategies on the acquisition of AMR is poorly understood. The question of how scavenging behaviours differ in their contributions to ARB exposure compared to active predation, particularly on live, peridomestic prey is not well-answered. ● Studies could focus on the functional roles of different gut microbes acquired from anthropogenic sources vs those acquired from rural settings such as in proximity to agricultural landscapes, within the context of ARB acquisition, particularly focusing on high-risk clonal lineages that may be more likely acquired from one source over another. | |
Social behaviour | Aggression and territoriality in disease spread | ● The relationships between wildlife behaviour, social systems, and disease transmission mechanisms between territories and individuals, especially in high-contact environments are acknowledged. ● Aggressive interactions and territorial behaviours (such as biting and excrement-marking) of species living or feeding in competitive environments can facilitate disease transmission. | ● No studies currently exist to determine if or how these aggressive or territorial behaviours may be a risk factor to facilitate AMR acquisition and dissemination in the environment. ● Environmental, longitudinal point-prevalence survey studies examining the correlation between territorial behaviours and AMR presence could be informative. ● Aggressive pack animals such as the Tasmanian Devil, could also be assessed for ARB upon disease outbreaks in the environment in comparison to those in captivity. |
The ‘Social Microbiome’ | ● Social species living in close proximity and engaging in behaviours like grooming and communal feeding or nesting exhibit similar microbiomes. ● The ‘social microbiome’ suggests that social behaviour may influence the composition of gut microbiota, potentially increasing bacterial exchange and HGT, increasing the likelihood of AMR transmission within groups via faecal contamination. | Effects of the ‘social microbiome’ in HGT leading to transmission of ARB and ARGs remain under-explored. Research must emphasize ecological dynamics of social interactions, in order to determine inter- and intra-species interactions. Further studies are needed to assess how contact within groups and between species may influence microbial community compositions and facilitate bacterial and gene exchange. | |
Movement and migration | Epidemiological link between habitats | ● Movement of wildlife has been identified as a driver of AMR dissemination in the environment, enabling species to serve as epidemiological links between habitats. ● Wildlife may be transmitting AMR between agricultural, urban, and natural environments by daily movement between these habitats, and contaminating with faeces or direct contact with domestic animals. | ● There is a lack of data surrounding AMR transmission dynamics across ecosystems due to wildlife movement and how these dynamics vary across different species and habitat. There is a need for deeper exploration into how human-induced habitat alteration (e.g. urbanization and intensification of agricultural practices) may be impacting movement patterns and consequently AMR spread. ● Limited information exists regarding reintroduction or translocation of wild animals following antimicrobial treatments in the context of conservation or in cases of wildlife rehabilitation. Research is lacking regarding whether this facilitates the spread of novel ARGs in surrounding wildlife. |
Intercontinental vectors | ● Wildlife species can act as vectors of ARB, carrying it over long distances during migration, through their excreta, facilitating intercontinental spread of AMR. ● Human-associated resistance has been detected in wildlife in very remote regions of the world, implying that migratory wildlife may play a role in AMR transmission, not just to other environments but to other wildlife species in those regions. | ● Most existing research relies on cross-sectional ‘snapshots’ on the presence of AMR in migratory wildlife, which fails to capture long-term trends. Limited longitudinal studies exist that monitor AMR in wildlife throughout migration to understand persistence and transmission dynamics, particularly with regards to external factors such as climate change that may alter migration pathways. ● More comprehensive studies are also required understand the genetic diversity and strain types of ARB acquired and disseminated by wildlife vectors, alongside the extent and duration of ARB shedding. |
AMR, antimicrobial resistance; ARB, antimicrobial resistant bacteria; ARG, antimicrobial resistance gene; and HGT, horizontal gene transfer.
Ecological factors influencing wildlife as reservoirs and disseminators
Feeding strategies and foraging behaviour
Current literature suggests that AMR profiles differ based on the variety and composition of the animal’s diet, coupled with their foraging strategies, which can influence the diversity and abundance of their gut microbiota (Moy et al. 2023). For instance, carnivores and omnivores, alongside species closely associated with human society are likely most at risk for ARB exposure and transfer (Vittecoq et al. 2016a). Indeed, the frequency of AMR in faecal E. coli isolates from carnivores has been found to be significantly higher than that of isolates from omnivores and herbivores (Bamunusinghage et al. 2022), and a 2021 study found that the highest level of resistant Enterococcus spp. was in omnivores compared to other nutrition-type animals (Hamarova et al. 2021). The latter study also documented differences in resistance profiles between birds and mammals, with a higher prevalence of ampicillin-resistance detected in birds, and vancomycin-resistance only detected in mammals (Hamarova et al. 2021). It has also been suggested that foraging behaviour may alter ARB profiles in wildlife species. For instance, predatory and scavenging species likely consume prey contaminated with resistant bacteria. Indeed, omnivorous gulls and raptors generally exhibit relatively high ARB colonization rates (Poeta et al. 2008, Guenther et al. 2010). Mammalian predators and omnivorous species appear to carry a wide diversity of ARB (Goncalves et al. 2013a, Dias et al. 2022c). Foraging and scavenging behaviour is commonly observed in proximity to anthropogenic environments e.g. in refuse, landfills, and agricultural land. Obligate and facultative scavengers such as vultures are implicated in this cycle as they feed on livestock carcasses that may be contaminated with ARB (Oteo et al. 2018, Blanco and Bautista 2020). It is possible that the ingestion of medicated or contaminated carcasses, peridomestic prey, or human refuse promotes increased antimicrobial exposure and accumulation of ARB through trophic levels (Ramey and Ahlstrom 2020). This may in turn place selective pressures in the gut microbiome of these animals possibly promoting development of novel ARGs (Witte 2000, Allen et al. 2010, Blanco and Bautista 2020). Herbivores may have lower overall AMR rates due to dietary and feeding exposure or microbial community composition; however, this requires further investigation (Bamunusinghage et al. 2022).
Social behaviour
The role of wildlife social behaviour has long been acknowledged in the context of parasite and infectious disease transmission (Møller et al. 1993, Macdonald 2006, Archie and Tung 2015), and the consequences of disregarding this impact have been also recognized. For instance in the case of the many failed attempts at rabies control in European red foxes (V. vulpes), for which the plans largely overlooked the species’ behaviour (Macdonald 1980b, Aubert 1992), and the influence of social systems of the Ethiopian wolf (C. simensis) and African wild dog (Lycaon pictus) on rabies transmission amongst the respective communities (Randall et al. 2006, Vial et al. 2006). Social behaviour such as aggression has also been connected to disease transmission. Devil facial tumour disease, an infectious cancer of the Tasmanian Devil (Sarcophilus harrisii) is transmitted as an allograft, spread via biting behaviour, which is common upon gathering to feed at carcasses and during mating (Storfer et al. 2017). Territorial behaviour could also be considered. Species like red foxes frequently mark their territory with faeces or urine (Macdonald 1980a), which, in principle, could spread resistant bacteria within and between territories. However, to date, no studies exist to determine if these aforementioned behaviours facilitate AMR dissemination in the environment.
Social behaviour is implicated in the composition of wild animal microbiomes. Species that live in close, complex social groupings and engage in social grooming such as meerkats (Suricata suricatta) (Leclaire et al. 2014), some carnivores (Hamede et al. 2009), and non-human primates (Pinacho-Guendulain et al. 2022), can have more frequent physical interactions than solitary-living species, thereby increasing the likelihood of bacterial exchange and HGT. This is an emerging concept known as the ‘social microbiome’ (Sarkar et al. 2020). Interactions between hosts and their external environments are also an important extrinsic factor shaping gut microbiome. For instance, interaction rates amongst wild baboons within cohabitating groups have been connected to variation in the gut microbiome, suggesting that close social interactions of hosts influences gut microbiome composition (Tung et al. 2015). This also extends to living conditions, e.g. communal nesting (Møller et al. 1993, van Veelen et al. 2017).
These studies indicate that wildlife social behaviour can influence the composition of their gut microbiome and therefore could influence the probability of evolution or spread of AMR. However, despite the body of knowledge surrounding the ecology of many wildlife species, there is still a significant knowledge gap regarding the manner through which social behaviour influences AMR acquisition. While evidence exists that close contact facilitates bacterial exchange, the dynamics of how ARGs are transferred or amplified among individuals in social groups, into other populations or whether it facilitates cross-species transmission and subsequently dissemination into other ecosystems, remain poorly understood.
Movement and migration
Movement and migratory behaviour of wildlife has been identified as a driver of AMR dissemination in the environment (Arnold et al. 2016). Insects like cockroaches and flies are important epidemiological links between agricultural land and humans, transmitting ARB between ecosystems (Zurek and Ghosh 2014). For instance, ‘filth flies’, that feed and develop in faecal matter have been implicated as vectors of AMR. Filth flies have been shown to be colonized with clinically-relevant (usually enteric) ARB, including ESBL-, carbapenemase-producing, and colistin-resistant (mcr-1 positive) bacteria, which often share similar genotypes with human and animal-associated bacteria when habitats are shared (Onwugamba et al. 2018). Flies may contribute to the maintenance of ARGs and ARB in the environment as ARB picked up by the fly in one ecosystem can multiply in its digestive tract, mouthparts, and regurgitation spots, hence are capable of disseminating the bacteria to other environments (Onwugamba et al. 2018).
Migratory species can act as efficient vectors of AMR in the natural environment via their excreta (Greig et al. 2015). Bi-annually, 5 billion wild birds migrate across continents, facilitating the spread of zoonotic pathogens globally (Ahmad et al. 2023). This, in particular relates to waterfowl and gull species, that have the ability to undertake long-distance migration and inhabit a wide range of habitats, from agricultural landscapes to beaches and to more remote regions such as mountain lakes, hence potentiating the spread of ARGs and ARB, even intercontinentally (Ahlstrom et al. 2021). For instance, AMR plasmids have been identified in Arctic bird isolates, from a habitat of sparse human interaction that should possess low (if any) antibiotic pollution (Sjolund et al. 2008). Moreover, one study found that E. coli strains isolated from gulls at landfills were identical in genotype to those isolated from wastewaters, indicating the transmission of ARB between anthropogenic waste and wild birds, which they may transport to recreational beaches and waters (Nelson et al. 2008). This is made all the more alarming due to the presence of carbapenemase-producing Enterobacteriaceae in gulls being documented in recent years (Dolejska et al. 2016, Vittecoq et al. 2016b).
Wildlife movement and migration can provide a link between ecosystems and may act as an epidemiological bridge for AMR dissemination in the environment (Agnew et al. 2015, Laborda et al. 2022, Sanchez-Cano et al. 2024). However issues begin to arise when we look at the scope of the current data on AMR in migratory wildlife. It largely consists of a series of brief ‘snapshots’ of sampled wildlife that prove the presence of AMR in the animal at the time of sampling, but do not provide evidence of, for instance, how far and for how long that animal is shedding the ARB into the environment (Arnold et al. 2016). And of course, we have little information on the effects of antimicrobial treatment and subsequent reintroduction or translocation of a wild animal for rehabilitation or conservation purposes. Data are limited or does not exist regarding whether these actions could facilitate environmental emergence and spread of novel ARGs to surrounding wildlife.
Environmental transmission pathways
Despite confirmed evidence of wildlife AMR carriage in the environment, and the consensus that it broadly originates from anthropogenic and agricultural pollution, the environmental transmission pathways at the OH intersection remain poorly understood (Benavides et al. 2024) (see Fig. 1). Only a limited number of studies have directly tested if and how wild animals maintain and disseminate ARB or ARGs between ecosystems (Dolejska and Literak 2019, Zeballos-Gross et al. 2021), nor have studies actually confirmed the concept of ‘spill-back’ from wildlife to humans or domestic animals, nor the public health implications this may hold (Benavides et al. 2024).
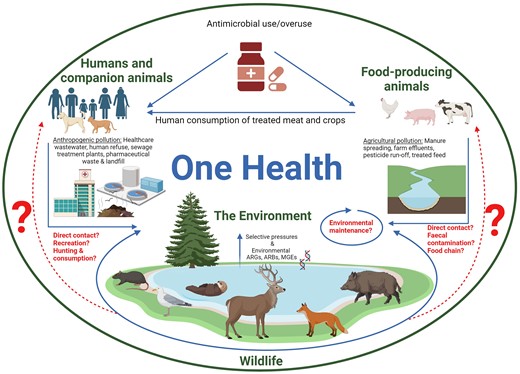
Diagrammatic OH representation of the environmental AMR transmission pathways that may exist (adapted from Ahmad et al. 2023). The over- and misuse of antimicrobials in human and veterinary healthcare leads to AMR. As antimicrobial metabolites are excreted during treatment, they are released into the environment and may also be maintained in that system as humans and companion animals consume treated livestock meat. Anthropogenic pollution through healthcare wastewater, human refuse, sewage treatment and landfill, and agricultural pollution through manure spreading, farm effluent, pesticide run-off, and treated feed enters the environment through various entry points and reaches wildlife. These pollutants pose selective pressures on the environment and animals leading to an increase in ARGs, ARBs, and mobile genetic elements (MGEs). In turn, this increases the likelihood of wildlife acquiring AMR. Major knowledge gaps exist with regards to ‘spill-back’ of AMR back to humans, companion animals or livestock via routes such as direct contact, recreation, hunting, and faecal contamination of farmland. Knowledge is also lacking regarding the capacity for wildlife to maintain AMR cycles in the environment. (Created in BioRender.com; agreement number LV278ZKGBX).
An ancient phenomenon and a modern crisis
The existence of AMR in environmental microbial communities is an ancient phenomenon, likely an evolutionary consequence of competition from natural antimicrobial metabolites produced by soil bacteria and fungi (D’Costa et al. 2011, Larsson and Flach 2022). In fact, most ARGs evolved slowly from genes with other functions (Morar and Wright 2010, Bhullar et al. 2012)—a veritable ‘arms race’ for fitness (Davies and Davies 2010, D’Costa et al. 2011, Gunther et al. 2022). Determinants of AMR have existed naturally for millions of years, likely subject to HGT in the environment long before anthropogenic selective pressures were imposed in the antibiotic era (Allen et al. 2010). In fact, studies have identified collections of microbial ARGs—on plasmids or even with similarity to modern variants—encoding resistance elements to antibiotic compounds that predate the era of anthropogenic mass-production and consumption by millions of years (D’Costa et al. 2011, Larsson and Flach 2022). Additionally, AMR-encoding genes have been found in areas of little to no anthropogenic intervention (Cristobal-Azkarate et al. 2014). These natural resistance mechanisms, e.g. efflux pumps, reduced permeability, enzymatic degradation, and biofilm formation, that result from selective pressures caused by environmental stressors such as antibiotic compounds, heavy metals and pesticides are often referred to as ‘intrinsic’ AMR (Allen et al. 2010, Arnold et al. 2016).
Technically, the historic presence of intrinsic AMR elements in the environment would be inconsistent with the suggestion of modern origin; instead implying that resistance evolution and dissemination is a solely natural phenomenon that predates the modern selective pressure of clinical antibiotic use (Wright 2007). However, a survey of soil samples from the Netherlands spanning the pre-and post-antibiotic eras (1940–2008) showed an increase in the relative abundance of ARGs for major antibiotic families (β-lactams, tetracyclines, and macrolides) in contemporary soil samples in comparison to pre-antibiotic era samples (Knapp et al. 2009). This is consistent with the proposition that, while environmental resistance is a natural phenomenon, its widespread growth is a modern concern linked to the contemporary global amplification of selective pressures by anthropogenic misuse and imprudent consumption and disposal of antimicrobials. This human-mediated pollution is incomparable and has paved the way for the emergence of AMR in the environment (Allen et al. 2010, Wellington et al. 2013, Greig et al. 2015).
The role of the environment in evolution and transmission
While environmental AMR is often associated with anthropogenic contamination (agricultural run-off, pharmaceutical wastes, insufficient sewage infrastructure, etc.) (Allen et al. 2010, Huijbers et al. 2015, Samreen et al. 2021), evidence exists that many of the ARGs observed in pathogens today originate from microbes already thriving in the environment (D’Costa et al. 2011, Wellington et al. 2013). The natural environment can be important for two major AMR-related processes (Bengtsson-Palme et al. 2018). It can serve as a source and reservoir of ARB and ARGs already present in the environment, as well as a facilitator for AMR dissemination (Bengtsson-Palme et al. 2023).
As a source and reservoir, the environment increases microbe exposure to both novel ARGs and other resistance determinants. A microbe can gain resistance via the acquisition of chromosomal gene mutations or gaining exogenous genetic material via HGT which allows for the exchange of MGEs, e.g. plasmids, transposons, and integrons between bacteria. When put under selective pressures, chromosomal ARGs that are present naturally in the environment can undergo evolutionary processes that lead to ‘acquired’ resistance in another environmental microbe (Davies and Davies 2010). The ARG will first gain mobility by, e.g. association with insertion sequences, or the formation of gene cassettes (Partridge et al. 2018), and will then relocate to an MGE for HGT to take place. Following this process, the bacterium carrying the ARG can be transferred to another host species, which may be human, livestock, or a wild animal. It is important to note that MGEs can encode for multiple different resistance genes to antibiotics and also to other environmental stressors, e.g. heavy metals and pesticides. Therefore, exposure to one environmental selective pressure may select for all the co-encoded genes and allow for the emergence of MDR (Davies and Davies 2010, Arnold et al. 2016) in any of these hosts.
When considering the environment as a facilitator for AMR dissemination, one must consider the various entry points through which ARB and other selective agents are released into different compartments of the environment. Importantly, it has been noted that landscapes characterized by livestock farming and dense human-population are more likely to be contaminated with ARB and ARGs (Manyi-Loh et al. 2018, Karkman et al. 2019). These potential hotspots include areas surrounding medical and care facilities, pharmaceutical manufacturing plants, aquaculture facilities and sewage wastewater treatment plants (WWTPs), landfills, and the pasturelands on which manure-based fertilizers are applied (Berendonk et al. 2015, Muurinen et al. 2017). Indeed, high levels of resistance determinants have been detected in various human-associated wastewaters, including municipal and sewage wastewater (Ng et al. 2017). A 2009 study investigating the final effluent from a WWTP found that the majority of ARGs detected encoded for clinically-relevant antimicrobials, including aminoglycosides, β-lactams, chloramphenicol, fluoroquinolones, macrolides, rifampicin, tetracycline, trimethoprim, and sulfonamide, alongside multidrug efflux genes. This demonstrates ongoing genetic inputs from clinical settings to wastewater treatment facilities (Szczepanowski et al. 2009, Abbassi et al. 2022). Moreover, many high-risk clonal lineages (successful, epidemic, or problem clones that share similar or identical phenotypic and genotypic characteristics; Peirano et al. 2020) of MDR pathogens such as P. aeruginosa (Petit et al. 2013) and En. faecium (Oravcová et al. 2017) have been recovered from WWTPs and other aquatic environments. These resistant bacteria and ARGs can then be spread further with wild animal migration (Stedt et al. 2014, Stedt et al. 2015), and even re-enter the human population via the environment, e.g. during recreational swimming, by the ingestion of fresh produce irrigated with contaminated water, consumption of contaminated (shell)fish, and inhalation of bioaerosols (Huijbers et al. 2015, Rahman et al. 2021, Leonard et al. 2022, Stanton et al. 2022). Additionally, humans that trap or hunt wild animals or consume the contaminated meat may be at risk for this transmission route (Magwedere et al. 2015, Vittecoq et al. 2016a).
Overall, limited understanding exists regarding the role of the environment in wildlife-mediated AMR dissemination. For instance, ARGs acquired in the environment have been described as ‘established’ or ‘latent’ (Inda-Diaz et al. 2023), with established ARGs generally already well-characterized in animals and human bacteria (Bengtsson-Palme et al. 2023). However, latent ARGs have not been well-observed in microbes thus far, meaning consequences of their acquisition by wildlife would be currently unknown nor would we identify them through routine environmental surveillance. Certainly more work is needed to interpret these ARBs and ARGs and their transmission dynamics in wildlife. A starting point would involve identifying potential AMR-drivers, classifying specific hotspots and conditions that facilitate HGT in wildlife and the environments, and confirming the extent to which anthropogenic pollution contributes to the evolution, spread, and persistence of AMR in the environment (McMahon et al. 2018, Arnold et al. 2024). Additionally, issues arise when considering how to differentiate between AMR acquired from human-derived environmental pollution, as opposed to the ‘intrinsic’ ARGs that are already present in the wild animal or its environment. A practical suggestion from Arnold et al. (2016) involves monitoring food chain transmission of resistance determinants associated with only human and livestock, and not generally observed in wildlife, such as fluoroquinolone resistance (Arnold et al. 2016, Darwich et al. 2019). Studies could also monitor for the occurrence of clinical- and veterinary-associated high-risk clonal lineages in wildlife, which may assist in elucidating the connections between different ecosystems (Abbassi et al. 2022) and perhaps in deducing direction of transmission in the environment.
Microbial community ecology in evolution and transmission
The dynamics of environmental AMR are complicated by the constant exchange of genetic materials within complex and highly diverse microbial communities (Flint et al. 2007, Arnold et al. 2016). This includes intra- and interspecies interactions that influence species’ evolution in response to selective pressures (Aminov 2011, Bottery et al. 2021). Environmental pollution with constant release of low concentrations of antibiotics and other selective compounds into the water and soil can alter microbial population dynamics and structures in different ecological niches and impact their ability to carry out functions. For example, research has demonstrated that antibiotic soil contamination alters beneficial soil bacteria abundance and diversity, and therefore impacts soil ecosystem functions such as nutrient cycling and plant growth promotion (Serwecińska 2020, Delgado-Baquerizo et al. 2022, Symochko et al. 2023). Interactions in the microbial community can be either directly through effects on, e.g. quorum sensing (Kalia 2013) or indirectly by the fitness selection of strains with resistance (Baquero et al. 1998, Widder et al. 2016, Larsson et al. 2018), which may support the development of AMR (Martínez 2008, Dias et al. 2015).
With regard to wild animals, a seemingly overlooked microbial community that must be taken into consideration is the gastrointestinal (GI) microbiome. The GI microbiome is the assemblage of all microbes and their genomes inhabiting the gut of a host and is an integral part of the host’s health. Its microbial communities carry out a range of functions, including energy homeostasis, metabolism, gut epithelial health, and response to pathogens via immune system activity and production of antibiotic compounds by the beneficial, commensal microbes that protect against pathogen colonization. It is hence heavily involved in host fitness, adaptation and disease resilience (Garcia-Gutierrez et al. 2019, Borrelli et al. 2020). Shifts in the bacterial diversity of the GI community can result in dysbiosis, i.e. a shift in the microbiota showing a depletion of commensal microbes and relative increase in pathogenic microbes (Schippa and Conte 2014, Heni et al. 2023); both of which can have negative effects on host health. Many factors can disrupt or shift diversity of the microbiome and compromise colonization resistance, including diet change, environment change, infection, and antimicrobial and pesticide exposure (Patangia et al. 2022, Heni et al. 2023). Antibiotic exposure has been acknowledged as a major disruptor of gut microbiota (Ramirez et al. 2020). In humans, antibiotic-associated dysbiosis is associated with reduced diversity of gut microbial species and abundance of certain taxa, decreased immunity, and the promotion of growth of ARB strains (Kesavelu and Jog 2023). Additionally, recent studies in both humans and wild animals have investigated disturbances in the gut caused by viral infections which facilitated co-infections and transmission of zoonoses (Wasimuddin et al. 2019, Schmid et al. 2022).
In order to fully understand the evolution and transmission of AMR in the environment, we must investigate it within the context of microbial communities and their ecological conditions, which is often overlooked in the research of environmental resistance (Bottery et al. 2021). It is important to consider that, with increasing human populations, agricultural intensification, and habitat fragmentation, wildlife are moving closer (Jones et al. 2013). This potentially increases human and livestock direct or indirect contact with wildlife microbiota or resistance determinants, thus transferring them among ecosystems (Sousa et al. 2014). It also increases wildlife proximity to the agricultural practices such as the use of potentially consequential chemicals. For example, the use of pesticides such as glyphosate has increasingly been associated with AMR development. Significant evidence has shown that pesticide exposure disrupts the GI microbiome. For instance, in the case of the honey bee (Apis spp.), whose gut bacteria contain the target enzyme for glyphosate. Exposure to glyphosate (at concentrations found in the environment) result in a decrease in relative and absolute abundances of dominant gut phyla, as well as increasing susceptibility to opportunistic pathogens (Motta et al. 2018). Glyphosate inhibits Snodgrasella alvi, which results in the reduction of aromatic amino acids essential for beneficial bee gut microbiota survival. It also inflicts a high mortality rate in bees (Motta et al. 2020, Battisti et al. 2021). Moreover, an in vitro analysis of poultry microbiota showed similar findings whereby pathogenic bacteria such as Clostridium botulinum, Cl. perfringens, Salmonella entritidis, Sa. gallinarum, and Sa. typhimurium were resistant to glyphosate, and commensal bacteria such as Bacillus badius, Bifidobacterium adolescentis, En. faecalis, En. faecium, and Lactobacillus spp. ranged from moderate to highly susceptible to glyphosate. As some of these beneficial bacteria inhibit the growth of Cl. botulinum in a healthy gut, it is thought that glyphosate toxicity might promote Cl. botulinum-type diseases in addition to gut dysbiosis (Shehata et al. 2013). From an OH perspective, this suggests that there is a selective pressure for glyphosate-resistant bacteria that are changing microbial communities and contributing to AMR. However as wildlife microbiomes are largely underexplored (Williams et al. 2018), it is unclear if it may be the case that selective pressure-associated dysbiosis can perpetuate a cycle of AMR in wild animals. The OH paradigm emphasizes the ecological connection between the health of humans, animals and the environment. Employing the OH approach to microbial community ecology allows for investigation into this communication of microbes within and between each compartment of the system, i.e. between humans, animals, and the environment, which may facilitate the spread of disease and AMR (Baquero et al. 2019).
Environmental drivers of wildlife AMR
As mentioned, environments characterized by intensive livestock-farming and dense human-population are more likely to be polluted with ARB and ARGs (Manyi-Loh et al. 2018, Karkman et al. 2019). Across all OH domains, antimicrobial usage is generally considered the primary factor driving the rise in AMR. This includes usage in human clinical settings and agricultural or animal husbandry settings (Vezeau and Kahn 2024). Wildlife are rarely exposed to antimicrobial compounds in a clinical setting but may acquire ARB from agricultural practices and direct human pollution (Radhouani et al. 2014, Carroll et al. 2015). The expanding human population, and ever-declining natural environments have resulted in increasing contact between wild animals and humans, livestock, and domestic animals. It is likely that this is increasing exposure of wildlife to AMR, is consequently increasing the risk of AMR acquisition and possible further transmission between different niches and hosts (Hassell et al. 2019, Plaza-Rodriguez et al. 2020).
Proximity to agricultural land and livestock
Close contact to agricultural landscapes is a driving factor for AMR in wildlife. Antimicrobials used in livestock account for 73% of the annual antimicrobials consumed globally (Torres et al. 2020a). The use of antimicrobials in such quantities in agriculture has been a major contributing factor to AMR development in the intestinal microbiome of livestock (Witte 2000). It is estimated that 75%–90% of antibiotics used in livestock is excreted largely unmetabolized and released into the environment by direct faecal contamination and via the application of manure (Kumar et al. 2005, Marshall and Levy 2011). Livestock manure and soil bacteria may harbour resistance determinants. In fact, as manure carries broad-host-range plasmids, it has been shown to promote HGT in soil, and when spread for fertilizer, its bacteria may mix with soil bacteria facilitating further evolutionary adaptation (Heuer et al. 2011, Woolhouse et al. 2015). Agricultural soils can hence act as hotspots for bacterial exchange of genetic material (Muurinen et al. 2017). These resistant bacteria and genetic elements may then be harvested as feed and cycled back to livestock, thereby increasing the possibility of transmission to humans via direct contact with these animals, the food chain, or the environment (Jones et al. 2008). Additionally, antibiotics may be discharged into the environment via the dispersal of feed, accidental spill of products, and antibiotic-contaminated dust originating from farms (Hamscher et al. 2003, Chee-Sanford et al. 2009). Importantly, the common agricultural practice of pesticide use is implicated in environmental AMR pollution. Pesticides are used to augment the production and health of crops by deterring disease and infestation by pests and disease carriers, such as mice, rats, and insects. Wildlife can be exposed to pesticides through multiple means. Indirect exposure includes contamination/runoff of the pesticides into local water bodies and groundwater, secondary poisoning, and pesticide drift. Direct exposure may be due to the ingestion of plants or prey previously exposed to pesticides, or being directly sprayed by pesticides (Beyond Pesticides.org). Pesticide exposure enhances AMR and promotes the spread of ARGs in the environment, along with microbial community evolution (Qiu et al. 2022). Indeed, it was proven that when strains of P. aeruginosa are exposed to glyphosate, they exhibit a significant resistance to imipenem, a clinically important carbapenem (Hahn et al. 2022). The mechanisms of pesticide-induced AMR have been reported as overexpression of efflux pumps (Kurenbach et al. 2015, Barbosa da Costa et al. 2022) and holistic transcriptional regulation, e.g. biofilm formation and oxidative stress defence (Xing et al. 2020).
Wildlife share the natural environment surrounding these farmlands for inhabiting, hunting, and foraging. For example, metagenomic analyses of wild boars’ intestinal contents have found evidence of crop plant ingestion (Skarzynska et al. 2020). This increases wildlife exposure to agricultural selective pressures such as pesticides, livestock waste, animal feed, or livestock ARB present on crops. It has even been suggested that the European badger (Meles meles) can acquire Salmonella spp. from food sources around poultry or game bird farms (Wilson et al. 2003) and have been shown to excrete vancomycin-resistant enterococci (VRE) [associated with treating poultry with the antibiotic growth promoter avoparcin (Mallon et al. 2002)]. Indeed, wildlife in close proximity to farmland have been found to harbour high levels of AMR. An investigation into the effects of proximity to a pig farm on AMR in E. coli isolates of wild small mammals (mice, voles, and shrews) found that animals residing in the vicinity of farms were five times more likely to carry tetracyline-resistant E. coli than animals living in natural areas. Indeed, tetracycline resistance was the most frequently observed resistance in isolates recovered from the pigs (83%) (Kozak et al. 2009). Additionally, a study in Vietnam found high levels of resistant E. coli with high similarity in profiles found between livestock and synanthropic rats and shrews trapped on the farms, in contrast to the mammals trapped in the wild. The authors also determined the risk of MDR to be ∼8 times greater among isolates from wild mammals trapped on the farms than among those trapped in the wild (Nhung et al. 2015).
Although agricultural practices are introducing antimicrobial compounds into the environment, it is unclear if wildlife-livestock interactions increase the risk of AMR dissemination. It is also unclear if this poses a risk to livestock, and in turn if there are potential implications for public health through the food chain (Vezeau and Kahn 2024). Livestock frequently graze on pastures that overlap with ranges shared by wildlife (Lee et al. 2022). This may allow for cross-species transmission of pathogens through either direct (nose-to-nose contact) or indirect (via shared food or water sources) mechanisms (Carrasco-Garcia et al. 2015, Pruvot et al. 2020). Given that wildlife are reservoirs for many livestock and zoonotic diseases, including rabies, bovine tuberculosis, and brucellosis (Wiethoelter et al. 2015), it is not out of the question that livestock sharing grazing space with wildlife may acquire their ARB (Lee et al. 2022). Research has acknowledged that wild mammals and birds can harbour ARGs and ARB, which may have the potential to be transmitted to livestock (Carroll et al. 2015). An Irish study conducted by Carroll et al. (2015) observed resistance to ampicillin, streptomycin, and tetracycline as the most prevalent phenotypes recovered from E. coli isolates from wildlife [herring gulls (Larus argentatus), black-headed gulls (L. ridibundus), lesser black-back gulls (L. fuscus), hybrid deer species (Cervus elaphus x Ce. nippon), and starlings (Sturnus vulgaris)], which were the same as those most commonly identified in retail meats and livestock in Ireland (Karczmarczyk et al. 2011, Carroll et al. 2015). Meanwhile, a study carried out by Lee et al. (2022) investigated the potential transmission of ARB and ARGs at the interface between cattle and wildlife species in the USA. The authors compared the gut microbiota and AMR profiles of the wild boar (Sus scrofa) and coyotes (C. latrans) with grazing cattle (Bos taurus), and environmental microbiota, and found that the wildlife species harboured more abundant ARB and ARGs compared to grazing cattle. Moreover, the gut microbiota of the cattle was significantly more similar to that of the wild boar sampled sharing the same home range. Resistance against clinically important antibiotics were also more prevalent in the wild animals than the cattle, which may suggest that wildlife could be a source of clinically relevant AMR for livestock (Lee et al. 2022).
This in turn, may be posing a risk for public health through the food chain. Empirical evidence supports that a wide range of ARB are present in the microbiome of wild mammals and birds (Greig et al. 2015). These bacteria may be re-entering the food chain through various routes including consumption of contaminated food and direct contact water sources during recreational swimming or by the ingestion of fresh produce irrigated with contaminated water (Rahman et al. 2021, Leonard et al. 2022, Stanton et al. 2022), and by cross-contamination during processing (Verraes et al. 2013). Importantly, water is a major transmission route for ARB. For example pathogens such as S. aureus, E. coli, K. pneumonia, and Salmonella spp strains that have been shown to possess ARGs and can survive in water (Monecke et al. 2016, Vittecoq et al. 2016a, Plaza-Rodriguez et al. 2020, Chiaverini et al. 2022). High risk clonal lineages, e.g. ST258 (CC258) carbapenemase (KPC)-producing K. pneumoniae, are also commonly detected in aquatic environments (Jelic et al. 2019). This increases the potential for wildlife to acquire ARB and ARGs while feeding or bathing. Moreover, wildlife harbouring these resistance determinants may defecate in aquatic environments and in turn contaminate groundwater and enable transport of ARB geographically to other ecosystems (Abbassi et al. 2022) thereby contaminating other landscapes.
Proximity to anthropogenic activity
With ever-expanding human populations, wildlife are increasingly forced to expand and feed on resources contaminated by anthropic ‘pathogen pollution’ (Wiethoelter et al. 2015). It therefore, does not come as a surprise that AMR is often observed in peri-domestic wildlife (Vittecoq et al. 2016a). In fact, evidence exists that proximity to human activity in urban settings increases AMR acquisition risk, and anthropophilic species are likely to be AMR carriers in the environment (Gilliver et al. 1999, Vittecoq et al. 2016a). Anthropogenic-associated environmental sources of AMR generally have a greater richness of ARB and ARGs, and more plasmid-associated ARGs (Rizzo et al. 2013, Berendonk et al. 2015). This is of significance as wildlife harbouring plasmid-associated ARGs are considered to behave as important secondary reservoirs and possible further vectors of resistance (Dolejska and Papagiannitsis 2018).
Urbanized species may behave as important OH bioindicators for AMR in the environment. Some gull species are well-adapted to anthropogenic environments, and commonly nest and loaf near agricultural land or habitats that receive wastewater effluent. These gulls have been shown to harbour higher levels of AMR than gulls associated with unpolluted waters (Allen et al. 2010, Ahlstrom et al. 2021). Studies of many gull species, such as herring gulls (L. argentatus) (Smith et al. 2014), black-headed gulls (L. ridibundus), and lesser black-back gulls (L. fuscus) (Carroll et al. 2015), have found similar evidence. Moreover, studies of microbiomes of wild birds indicate they often possess human-associated bacteria and ARB, including clones common in hospitals (Oteo et al. 2018, Stepien-Pysniak et al. 2019). Additionally, methicillin-resistant S. aureus (MRSA; a prominent nosocomial infection) isolates were recently isolated in 3.8% of faecal samples from Canadia geese (Branta canadensis) in Northeastern Ohio, USA that possessed the high-risk clonal lineages ST5 and ST8 (Thapaliya et al. 2017). The ecological behaviour of nesting near human-inhabited areas allows gulls and waterfowl to acquire and accumulate these clinically-relevant ARB and ARGs (Allen et al. 2010, Dolejska et al. 2016, Vittecoq et al. 2016b, Ahlstrom et al. 2021).
In mammals, the wild boar (Sus scrofa) has been the subject of many investigations as they are known environmentally-acquired AMR-carriers (Navarro-Gonzalez et al. 2018). The Western European population has increased distribution in recent decades and now inhabit many anthropized landscapes. A 2018 study compared the frequency of AMR in isolates of bacterial indicator species from urban and rural wild boars in Spain. The authors found a significantly higher frequency of tetracycline-resistant En. faecium (70.0% vs 36.4%), a higher-level resistance to streptomycin (30.0% vs 4.5%) and E. faecalis isolates were more frequently resistant to trimethoprim in urban compared to rural wild boars. These results suggest that urban wild boars may be more exposed to certain ARB or ARGs in the anthropogenic landscape than their rural counterparts (Navarro-Gonzalez et al. 2018). Furthermore, an investigation into different exposures of anthropogenic AMR in raccoons (Procyon lotor), found that extended-spectrum cephalosporin-resistant E. coli was more likely to be isolated from urban rather than suburban raccoons, and a greater richness of E. coli sequence types found in raccoons near with WWTPs (Worsley-Tonks et al. 2021). There are many other studies that observed similar associations (e.g. Österblad et al. 2001, Gunasekara et al. 2024).
Additionally, for some synanthropic species such as rats, living in close proximity to humans may contribute to the HGT of ARGs among different pathogens in their microbiome. An analysis of the microbiome of Rattus norvegicus from hospital environments revealed a high abundance of vancomycin resistance genes (vancomycin being the preferred antibiotic for the treatment of MRSA) (Hansen et al. 2016). Moreover, it has been shown that microbes within rats’ microbiomes can efficiently exchange ARGs (Jacobsen et al. 2007, Thumu and Halami 2019). Another study using phylogenetic analysis of MDR E. coli isolated from a grey-headed flying fox (Pteropus poliocephalus), a fruit bat endemic to eastern Australia, found E. coli lineages associated with humans, provides evidence of anthropogenic MDR and pathogenic E. coli transmission from humans to wildlife (McDougall et al. 2021). This bat commonly inhabits urban environments and encounters human-associated bacterial pollution.
Moreover, contamination of aquatic systems by pesticides in groundwater and surface water due to anthropogenic misuse of pesticides in urban or domestic gardening and farming may pose a bioaccumulation risk for otters in coastal aquatic ecosystems (Carpenter et al. 2014, Wainstein et al. 2022). As pesticide contamination causes drift, leaching and runoff, more pathways of exposure in marine life, e.g. by ingestion, integumentary exposure, and respiration are created whilst terrestrial animals are exposed primarily through ingestion (Evalen et al. 2024). Hence, aquatic animals such as otters (and fish and other aquatic animals, though outside the scope of this review) may be more at risk for possible AMR-related effects than terrestrial animals. In fact, glyphosate added to lake water in a mesocosm was shown to cause an increase in ARGs and multidrug efflux pumps in the natural freshwater bacteria (Barbosa da Costa et al. 2022). These reports propose that if aquatic wildlife were to be colonized by ARB, that pesticide use could be promoting more AMR in the environment via aquatic systems.
However, the role of wildlife in the spread of AMR may not solely be driven by anthropogenic factors, as resistance can also be found in wildlife living in areas without human interaction (Vezeau and Kahn 2024). For example, resistance to ciprofloxacin, a relatively recently developed, synthetic antimicrobial, was discovered in remote troops of monkeys in Mexico (Cristobal-Azkarate et al. 2014). In contrast, a study in Germany found the prevalence of ARB in wild boar, roe deer, and wild waterfowl—species generally expected to show relatively higher levels of resistance—to be low, possibly due to low antimicrobial or ARB exposure (Plaza-Rodriguez et al. 2020). Additionally, Polar bears (Ursus maritimus) in Arctic Svalbard that would be relatively isolated from human activity, have been found to have low levels of ampicillin resistance in their gut microbiota (Glad et al. 2010). Hence, there are exceptions to the generally positive correlation between proximity to anthropogenic activity or waste and detection of clinically-relevant ARGs in wild species (Arnold et al. 2016).
AMR in wildlife is an important indicator of anthropic pollution (Laborda et al. 2022); however, our understanding is incomplete regarding the drivers of AMR in wildlife and what the significance of any one driver may be. Given the fact that wildlife do not generally receive doses of antimicrobials, it is feasible to conclude they are net recipients of AMR in the environment (Hassell et al. 2019). However, the presence of AMR in wildlife is not solely driven by human activities, suggesting a complex interplay of factors (Swift et al. 2019), and hence transmission routes from wildlife to humans must be elucidated.
Gaps in intervention strategies
While pollution control measures, sewage treatment, and public health education on antimicrobial stewardship are important intervention programmes and likely to continue globally, investigating potential intervention strategies to mitigate AMR spread in wildlife populations must be a priority. Furthermore, gaps and obstacles associated with current intervention strategies must be addressed. These obstacles include the lack of integration of wildlife into AMR OH policy instruments and surveillance frameworks, the need for standardized approaches in utilizing wild animals as sentinel species to monitor environmental AMR trends, and the challenges in implementing high-throughput sequencing technologies for advanced, holistic environmental surveillance. Incorporating wildlife into surveillance efforts ensures a comprehensive strategy for addressing AMR in the environment, as it acknowledges the complex interplay between human, animal, and environmental health—essential to the OH paradigm.
Wildlife in OH AMR policy and surveillance
A number of policy instruments exist globally for the monitoring and control of AMR. In 2015, the WHO, WHO for Animal Health, and Food and Agriculture Organisation of the United Nations developed a Global Action Plan (GAP) on AMR (WHO 2015). The aim of this plan was to provide a comprehensive overview of what AMR is and how it spreads, and to strengthen the knowledge and evidence base through surveillance and research into antimicrobial use in humans, animals and the environment. The WHO GAP for AMR provided guidelines for countries to develop their own AMR national action plans (NAPs) within 2 years to help combat AMR using a OH approach. As of 2022, there are a total of 78 NAPs (70 WHO approved, eight not approved) from the five global regions (Willemsen et al. 2022). However, a study undertaken to investigate countries’ NAPs based on their inclusion of OH and wildlife components, found that only 32 out of the 66 countries studied have NAPs reporting policy objectives aligned with a OH approach, and only seven mentioned wildlife (Benavides et al. 2024). Whilst efforts are underway to understand the epidemiology of AMR, the majority of research and regulations remain focused on humans and livestock (Anjum et al. 2021, Mitchell 2023). The absence of wildlife in AMR policy and surveillance programmes seems imprudent, considering the lessons only recently learned from outbreaks such as the recent COVID-19 pandemic, which demonstrated the significant role wildlife play in transmission and dissemination of microbes (Perveen et al. 2021, Shaheen 2022). This makes it all the more concerning that wildlife and their habitats are rarely considered in AMR action plans and policy on AMR prevention and control nationally and globally (Arnold et al. 2016, Iossa and White 2018, Mitchell 2023).
There is also a deficit of wildlife-related AMR research. In fact, a recent study analysing and mapping 20 years (1999–2018) of global peer-reviewed AMR research using a technique known as structural topic modelling, created a comprehensive thematic overview of the AMR research field, and the theme of wildlife was not recognized within 158 616 articles screened (Luz et al. 2022). Knowledge on the health impacts of AMR colonization and dissemination in wildlife and the environment is also lacking in the literature (McCubbin et al. 2021, Mitchell 2023, Li et al. 2024). Very few studies have investigated, for instance, the extent of clinical treatment with antibiotics in wildlife rehabilitation centres or post-exposure microbiome changes (Arnold et al. 2016, Benavides et al. 2024). This lack of focused research is alarming given that wildlife is a fundamental component of the environment, notwithstanding the increasing contact wildlife have with anthropogenic landscapes (Jones et al. 2013). It will also impede strategy-planning when looking to inform surveillance in settings where longitudinal data on migratory wild animals harbouring and disseminating AMR between wild and domestic regions is scarce. Debatably, the fact that the significance of transmission dynamics and mechanistic drivers of AMR in wildlife are under so underrepresented in the scientific literature may be impeding development of effective, real-world management strategies of AMR in all OH components in the environment (Li et al. 2024).
Sentinel species for surveillance
Surveillance is a core principle of global public health protection, and many national and international systems are in place for infectious disease (Pruden et al. 2021). In the context of AMR, monitoring is routinely implemented in human clinical settings and veterinary (particularly food-producing animal) settings (EFSA and ECDC 2021, WHO 2022). The targeted species are generally faecal indicator bacteria and the ESKAPE (En. faecium, S. aureus, K. pneumoniae, A. baumannii, P. aeruginosa, and Enterobacter spp.) pathogens (Pruden et al. 2021). For instance, the National Antimicrobial Resistance Monitoring System is a system implemented in the USA to compare enteric ARBs detected in food production systems to clinical strains, while the European Antimicrobial Resistance Surveillance network in Veterinary medicine assesses the risk of AMR transmission between diseased animals and humans via routes such as direct contact with companion or food-producing animals, in order to support prudent veterinary use of antimicrobial therapy in Europe (Mader et al. 2021).
Of course, clinical and veterinary surveillance of AMR are crucial for healthcare and food safety. However, comprehensive and comparable monitoring data on AMR in the environment is currently inadequate to understand environmental AMR dynamics (Pruden et al. 2021), hindering our ability to fully interpret resistance trends for effective intervention. As microbiological systems are interconnected and constantly interacting, through human settings, animal settings, and the environment, the OH approach is instrumental in gaining a holistic picture (Bengtsson-Palme et al. 2023). Following a OH framework, existing AMR surveillance strategies could be expanded to include wildlife as sentinels of environmental AMR. Sentinels or bioindicators are species that hold potential to be used as an early warning system for human health risks. The implementation of systematic AMR surveillance using wildlife sentinel species could offer the tools necessary to monitor reservoirs of AMR in the environment, understand and predict environmental transmission dynamics, detail environmental contamination, provide evidence on impacts of antimicrobial misuse, and even provide feedback on positive effects of political interventions (Martel et al. 2001).
Importantly, synanthropic and urbanized wildlife species could hold the key in evaluating the dissemination of AMR within the environment across gradients of anthropogenic inputs. For instance, red fox (V. vulpes), as an anthropophilic species, is of particular interest in the AMR transmission cycle and has been proposed as a sentinel for environmental AMR (Garcês and Pires 2021). It is a particularly adaptable species, adjusting to local environmental conditions in urban, suburban, and rural areas. Studies have shown the occurrence of AMR in foxes fluctuates with habitat (Mo et al. 2018), and their microbiomes showing adaptation to ecological niches (Wang et al. 2022). Foxes are omnivorous, opportunistic predators, and scavengers (Garcês and Pires 2021), often observed feeding on human refuse. This synanthropic behaviour allows them to frequently interact with anthropogenic sources of ARB (Mo et al. 2018), granting opportunities for acquisition and transmission of AMR directly or indirectly through reservoirs, such as food waste, refuse, sewage, and wastewater (Plaza-Rodriguez et al. 2020). Studies of AMR in foxes remain relatively scarce (Garcês and Pires 2021, O’Hagan et al. 2021); however, evidence of carriage does exist. Investigations conducted in Portugal identified AMR in red fox isolates, including VRE (Radhouani et al. 2011) and ESBL-producing E. coli (Radhouani et al. 2012), alongside other studies that identified quinolone-resistance genes (Kaspersen et al. 2020), and mecA-positive staphylococci which confers resistance to methicillin (Carson et al. 2012). In Ireland, the only published study carried out was in Northern Ireland between 2018 and 2019 (O'Hagan et al. 2021). The authors found 11.53% of fox faecal samples carrying ESBL, all presenting MDR.
The red fox, among other potential sentinel species (see Table 2) could be regarded as an epidemiological link between ecosystems. Stakeholders must prioritize the intersections of OH compartments, and utilizing wildlife sentinel species for the monitoring of AMR in the environment may be the best, most cost-effective course of action. In order to develop a sustainable sentinel species monitoring system, the first step will be to identify, prioritize, and specify the species and scenarios of wildlife–human–domestic animal interactions where AMR in wildlife could have public health or environmental impacts. It may be useful to classify the potential species based on their diet and foraging behaviour, behavioural ecology, and movement (e.g. migratory or resident, home range size, etc.) (Benavides et al. 2024). Following this, an evaluation of the cost-effectiveness will need to be conducted. From a cost-efficiency standpoint, sampling could include locations or specific species for which surveillance is already underway. For example, if surveillance at rivers is already in place for water quality testing, a logical decision would be to select otters as the bioindicator species and collect samples simultaneously, or if a culling or monitoring programme is ongoing, such as the Echinococcus multilocularis surveillance scheme for the red fox (EFSA 2023a; see Table 2), to test the faeces of the collected carcasses. Of course, in an ideal scenario, the sampling scheme would be non-invasive, possibly involving the sampling of sites on or near latrines, where the species defecate. As the sampling sites would be static, this would allow for the tracking of changes of AMR transmission longitudinally and potentially link the results to anthropogenic activity nearby (Pesapane et al. 2013, Bengtsson-Palme et al. 2023). However, unfortunately systematic sampling for AMR in this manner is currently underexplored.
Case studies for possible sentinel species highlighting ecological rationale for sentinel use, and ongoing surveillance schemes that hold potential to be utilized for resistance monitoring, with examples of academic studies investigating presence of in the species.
Species . | Ecological aspects for sentinel use . | Monitoring feasibility . | Potential synergistic schemes . | Publications . |
---|---|---|---|---|
Mammals | ||||
Red fox (V. vulpes) | Habitat and ecology: Urban or anthropized environments, peri-urban areas, rural or agricultural landscapes, and natural forestland. Opportunistic omnivores and scavengers, moving between natural, agricultural, and urban environments, where they feed on both synanthropic prey species and scavenge in human refuse.Transmission: Their role as generalists means they encounter diverse bacteria from different sources, including those from human waste and agricultural runoff, increasing exposure to antibiotic residues and ARB from humans and the food chain.Dissemination: Daily movement across landscapes may spread ARB between different suburban and urban spaces, and agricultural land, facilitating AMR transfer between these systems. Commonly come into contact with domestic pets and their food, hence indirectly with humans. ARB can thus enter the household, posing a potential public health risk. | Sampling: Highly mobile animals, making live capture more challenging. However, due to their widespread geographic distribution and human proximity, non-invasive faecal sampling is relatively easy, allowing for straightforward, broad spatial monitoring coverage. Carcass sampling from roadkill is also suitable as tissue sampling (e.g. liver/kidney) can reveal accumulated antimicrobial compounds or ARB.Bioindicator: Foxes forage in urban, peri-urban, and farming landscapes, where antimicrobials are often present. Their interaction with these human-modified habitats contributes to their suitability as relevant bioindicators of AMR in anthropogenic and agricultural environments. | Ongoing schemes: The European Food Safety Authority conducts annual monitoring for Ec. multilocularis in red foxes to assess zoonotic risk across Europe (EFSA 2023a). The EU programme for the eradication of rabies is ongoing, in which the effects of the vaccination programme are evaluated by laboratory examinations of randomly hunted foxes (EC 2015). Additionally, the UK Centre for Ecology and Hydrology runs a number of partner schemes such as the Garden Wildlife Health scheme in which the public can send samples of sick or dying wildlife in their garden.Potential for AMR integration: These existing surveillance frameworks could be expanded to include AMR screening as faecal sampling and carcass collection are already involved. | (Asai et al. 2022a); (Asai et al. 2022b); (Botti et al. 2013); (Carella et al. 2022); (Carson et al. 2012); (Chiaverini et al. 2022); (Costa et al. 2006); (Cristovao et al. 2017); (Dec et al. 2020); (Dias et al. 2022a); (Dias et al. 2022b); (Gambino et al. 2021); (Garcia et al. 2020); (Garcia et al. 2022); (Haenni et al. 2020); (Kaspersen et al. 2018); (Kaspersen et al. 2020); (Literak et al. 2009); (Maksimovic et al. 2022); (Mo et al. 2018); (Monecke et al. 2016); (Nowakiewicz et al. 2016); (O’Hagan et al. 2021); (Osinska et al. 2022); (Radhouani et al. 2011); (Radhouani et al. 2012); (Radhouani et al. 2013); (Skarzynska et al. 2020); (Turchi et al. 2019); (Uelze et al. 2021). |
Eurasian otter (Lutra lutra) | Habitat and ecology: Aquatic environments such as freshwater rivers and lakes, often in suburban landscapes, and coastal estuaries, where they feed and bathe.Transmission: These aquatic habitats are common endpoints for agricultural runoff, sewage discharge, and industrial waste, hence are reservoirs for antibiotic metabolites, ARB and ARGs.Additionally, as apex predators, prey consists mainly of fish, crustaceans, and amphibians, meaning otters bioaccumulate contaminants, including heavy metals, pesticides, and ARB over time.Dissemination: Often found in interconnected waterways and are a highly mobile species. This potentially leads to direct faecal contamination into previously unpolluted water bodies, sediment, and surrounding soil, facilitating the movement of different strains of ARB across habitats | Sampling: Spraint collection near rivers and waterways is relatively easy. The otter’s high dependence on aquatic ecosystems also makes them suitable for water quality monitoring. Tissue sampling can also be carried out from roadkill or dead individuals found during routine conservation monitoring, offering an effective non-invasive method.Bioindicator: They could be effective bioindicators of long-term trends in environmental contamination and AMR presence and prevalence temporally and spatially across trophic levels in the aquatic ecosystem. | Ongoing schemes: Otter spraints are often monitored for conservation purposes and for contaminants, including heavy metals and organic pollutants, through national and European conservation programmes, such as the Irish National Otter Survey 2023–2024, and the Otter Project, run by Cardiff University, UK. Otters’ spraint and roadkill carcass collection is already a common non-invasive method to assess population health.Potential for AMR integration: These existing schemes can be expanded to include AMR surveillance in aquatic environments. Adding AMR testing to spraint samples from riverbanks and other aquatic habitats could provide non-invasive insights into waterborne ARB. | (Alonso et al. 2017); (Dias et al. 2022b); (Dias et al. 2022c); (Garcia et al. 2020); (Garcia et al. 2022); (Kumari et al. 2022); (Loncaric et al. 2013); (Mengistu et al. 2022); (Oliveira et al. 2010); (Semedo-Lemsaddek et al. 2013); (Semedo-Lemsaddek et al. 2018). |
Rats (Rattus spp.) | Habitat and ecology: Urban or anthropized environments, peri-urban areas, rural, or agricultural landscapes. Behave as omnivorous scavengers, accessing animal feed and feeding on human refuse.Transmission: Often exposed to high levels of ARB and antibiotic metabolites in agricultural run-off, sewage systems, and landfills.Dissemination: Known carriers of zoonotic pathogens and resistant bacteria. Could potentially disseminate anthropogenic-associated resistant bacteria through their faeces, urine, and direct contact with humans, livestock, and domestic animals. | Sampling: Near-global distribution and thrive in human-modified environments. They are easy to trap, and their faeces are abundant, meaning sampling can be done frequently in areas of interest, allowing for extensive surveillance in cities, peri-urban areas, and agricultural settings.Bioindicator: Given their close association with human settlements and their scavenging behaviour, rats may be the ideal sentinel for urban, human-associated AMR. | Ongoing schemes: Urban rat populations are frequently monitored for zoonotic diseases, particularly in large cities by public health bodies. The CDC, EPA and local municipal bodies run rodent control programmes that often include pathogen surveillance (Brown and Laco 2015, EPA 2024)Potential for AMR integration: Rat faeces could be routinely tested for ARB in urban monitoring programmes that already track rodent-borne diseases like strains of Rickettsia and Bartonella. | (Asai et al. 2020); (Azimi et al. 2021); (Bamunusinghage et al. 2022); (Blanco Crivelli et al. 2018); (Desvars-Larrive et al. 2019); (Dominguez et al. 2023) (Ge et al. 2019); (Gomez et al. 2014); (Guenther et al. 2012); (Guyomard-Rabenirina et al. 2020); (Hansen et al. 2016); (Himsworth et al. 2014); (Himsworth et al. 2015); (Himsworth et al. 2016); (LeHuy et al. 2020); (Lozano et al. 2015); (Nhung et al. 2015); (Raafat et al. 2020) (Sano et al. 2023); (Schaufler et al. 2018); (van de Giessen et al. 2009). |
Deer species (Cervidae) | Habitat and ecology: Reside in forests, grasslands, peri-domestic areas, and agricultural landscapes. They are herbivorous, feeding on crops, silage, and animal feed.Transmission: Potentially exposed to agricultural ARB through consumption of treated crops or contaminated water.Dissemination: May transfer resistant bacteria from peri-domestic areas to agricultural soils and water sources and vice-versa, through their faeces. | Sampling: Large, common, and widespread, hence relatively easy to monitor. Often hunted for recreation and routine wildlife management, offering opportunity for the creation collection schemes for faeces, tissue or blood from hunted game carcasses, creating a passive means of tracking resistance trends.Bioindicator: Deer’s frequent foraging near agricultural land make them valuable sentinels for AMR in areas where runoff and antibiotics may be present. | Ongoing schemes: Deer populations are controlled by national deer culling and carcass collection programmes (e.g. Irish Deer Management Strategy Group 2023). Deer populations are also regularly monitored in Europe and North America for diseases such as chronic wasting disease and bTB (e.g. EFSA 2023b), and are commonly monitored in academic studies, to provide empirical bases for deer management (e.g. Keenan et al. 2023, SMARTDEER project).Potential for AMR integration: Routine population management, including culling, hunting seasons, and wildlife health assessments, provides distinct, straightforward opportunities for sampling and AMR monitoring using faeces or tissue samples. | (Alonso et al. 2016); (Alonso et al. 2017); (Asai et al. 2020); (Ballash et al. 2021); (Ballash et al. 2022); (Carroll et al. 2015); (Chiaverini et al. 2022); (Dias et al. 2015); (Dias et al. 2022b); (Dias et al. 2022d); (Dias et al. 2022e); (Elsby et al. 2022); (Gambino et al. 2021); (Garcia et al. 2020); (Homeier-Bachmann et al. 2022); (Plaza-Rodriguez et al. 2020); (Ramos et al. 2022); (Rogers et al. 2018); (Sarker et al. 2019); (Smith et al. 2014); (Smoglica et al. 2023); (Torres et al. 2021). |
Wild boar (Sus scrofa) | Habitat and ecology: Agricultural landscapes, peri-urban areas, forest, and landfills. An omnivorous and highly adaptable diet, eating vegetation, insects, small mammals, ground-nesting birds, amphibians, and carrion. They scavenge and forage in both natural and agricultural environments.Transmission: They are referred to as ecosystem engineers as they are often seen rooting in soil for food. This rooting behaviour can disturb soil and soil processes, leading to bacterial exchange and thereby increasing their exposure to ARB in manure-fertilized fields. They are also feeding on animals residing in the agricultural and peri-urban landscape, and therefore may be acquiring and maintaining ARB through trophic levels.Dissemination: They have the potential for direct and indirect contact with humans and livestock which can facilitate the exchange of ARB, making them effective indicators of AMR in areas impacted by human activity, particularly in soil. | Sampling: Widespread across Europe and increasingly encroach on agricultural and urban areas, providing opportunities to monitor AMR in various environments. Often hunted for management, providing opportunities to collect biological samples such as tissues, blood, and faeces from carcasses for AMR surveillance.Bioindicator: As culling schemes are ongoing and as faecal sampling can also be done non-invasively in areas where they forage, they are a practical choice for longitudinal monitoring programmes. | Ongoing schemes: Wild boar are considered invasive in some countries hence populations are culled for management (e.g. DEFRA 2008). They are also included in general zoonoses monitoring programmes based on Directive 2003/99/EC (e.g. the German National Zoonoses Monitoring Programme, Plaza-Rodriguez et al. 2020).Wild boar are also subject to specific wildlife disease surveillance programmes, particularly for African swine fever (e.g. EFSA 2018, Guberti et al. 2019) where urine, faeces, and carcasses are routinely tested.Potential for AMR integration: These monitoring programmes, which involve tissue and blood sampling during routine hunting and culling, could be expanded to include AMR testing. | (Asai et al. 2020); (Bachiri et al. 2018); (Chiaverini et al. 2022); (Cristovao et al. 2017); (Dias et al. 2022b); (Dias et al. 2022f); (Gambino et al. 2021); (Garcia et al. 2020); (Garcia et al. 2022); (Gunther et al. 2022); (Homeier-Bachmann et al. 2022); (Lee et al. 2022); (Literak et al. 2009); (Mercato et al. 2022) (Navarro-Gonzalez et al. 2018); (Plaza-Rodriguez et al. 2020); (Ramos et al. 2022); (Rega et al. 2022); (Skarzynska et al. 2020); (Torres et al. 2020b); (Wasyl et al. 2018). |
Birds | ||||
Gulls (Laridae) | Habitat and ecology: Wetlands, agricultural lands, coastal regions, and urban areas. They are opportunistic scavengers and often feed on human waste in city centres, landfills, sewage plants, alongside agricultural fields.Transmission: Feeding in these diverse environments places them in direct contact with ARB and antimicrobial metabolites, thereby increasing their risk of acquiring AMR.Dissemination: Migratory birds can acquire ARB in one region and transport to another, potentially introducing new ARGs across continents, linking distant ecosystems. With this, their faeces can contaminate recreational waters, beaches, and into densely human-populated urban areas, posing a risk to public health. Moreover, as they congregate in large flocks during migration and at breeding grounds, the exchange of ARB and ARGs to resident bird species and aquatic organisms is accelerated. | Sampling: Gulls are highly mobile, making faecal collection from loafing sites and feeding areas a straightforward, efficient and non-invasive method to sample for resistant bacteria.Bioindicator: They are common in coastal and agricultural environments, and frequent interaction with humans and human waste, which makes them important for bioindicators of AMR in urban areas affected by human activities and water pollution. | Ongoing schemes: Gulls are included in avian influenza surveillance programmes (EFSA et al. 2024), involving faecal and water sampling to assess disease presence in migratory bird populations. Moreover, the Predatory Bird Monitoring Scheme is ongoing in the UK, in which the general public can send in bird carcasses to analyse for pollutants and contaminants. As the infrastructure is already available, gull monitoring would be relatively straightforward to include in this scheme.Potential for AMR integration: Faeces collected for avian influenza could be screened for AMR. This would be particularly useful if results were integrated into tracking data for the birds (e.g. BirdWatch Ireland gull tracking programmes), allowing for higher resolution spatial tracking of ARB spread over time. | (Ahlstrom et al. 2018); (Ahlstrom et al. 2021); (Ahlstrom et al. 2022); (Atterby et al. 2017); (Bonnedahl et al. 2009); (Bonnedahl et al. 2010); (Carroll et al. 2015); (Dolejska et al. 2007); (Dolejska et al. 2016); (Gambino et al. 2021); (Guenther et al. 2010); (Hernandez et al. 2010); (Jarma et al. 2021); (Liakopoulos et al. 2016); (Literak et al. 2010); (Nelson et al. 2008); (Poeta et al. 2008); (Simoes et al. 2010); (Sjolund et al. 2008); (Smith et al. 2014); (Stedt et al. 2014); (Stedt et al. 2015); (Vittecoq et al. 2016b). |
Waterfowl (Anseriformes) | Habitat and ecology: Wetlands, relying on them for food, shelter, and breeding. Many species have adapted to, and thrive in anthropogenic and agricultural environments, where they interact with urban and farming wastewater systems. Opportunistic feeders, including aquatic plants and invertebrates, and forage in agricultural fields especially during migration or winter.Transmission: Wetlands can act as reservoirs for ARB, which waterfowl may acquire. They may also move between habitats, exposing them to other anthropogenic sources of AMR, such as manure runoff, antibiotic metabolites, and pesticides. Some waterfowl species nest near human activities, such as in ponds or reservoirs near wastewater discharge, increasing their exposure to pollutants and resistant bacteria. Waterfowl are social, and form large, occasionally mixed-species flocks (other waterbirds such as gulls or waders), particularly during migration, wintering, and at feeding sites. This behaviour may increase the likelihood of (cross-species) ARB transmission through direct contact or faecal contamination.Dissemination: They migrate large distances, during which large flocks will use stopover sites that can become hotspots for ARB exchange within the flock and to resident bird colonies and aquatic organisms. This in turn contributes to the local spread of ARB in a previously naïve ecosystem. | Sampling: Faecal sampling, along with agricultural and urban water and sediment sampling from wetlands, lakes, and rivers where waterfowl congregate can be used to monitor AMR in aquatic environments. Water and sediment samples from these areas can also be tested for resistant bacteria.Bioindicator: Waterfowl are widespread, particularly in freshwater wetlands and coastal areas, providing extensive coverage for monitoring AMR in aquatic systems. Their movement between natural and agricultural environments exposes them to antibiotics from agricultural runoff, making them good bioindicators of AMR in aquatic systems. | Ongoing schemes: Waterfowl are included in avian influenza surveillance programmes (EFSA et al. 2024), involving faecal and water sampling to assess disease presence in bird populations. They are also included in zoonoses monitoring programmes based on Directive 2003/99/EC (e.g. the German National Zoonoses Monitoring Programme).Potential for AMR integration: AMR monitoring can be integrated into the existing avian influenza waterfowl surveillance by simply incorporating AMR testing into their current disease tracking schemes. Monitoring can also be integrated into existing wildlife and wetland conservation and tracking programs [e.g. British Trust for Ornithology Wetland Bird Survey (WeBS)] which would be feasible and cost-effective and allow for higher resolution and more meaningful spatial surveillance of ARB spread over time. | (Agnew et al. 2015); (Ahmed et al. 2019); (Cole et al. 2005); (Gambino et al. 2021); (Guenther et al. 2010); (Jarma et al. 2021); (Literak et al. 2010); (Plaza-Rodriguez et al. 2020); (Sjolund et al. 2008); (Stepien-Pysniak et al. 2019), (Thapaliya et al. 2017). |
Species . | Ecological aspects for sentinel use . | Monitoring feasibility . | Potential synergistic schemes . | Publications . |
---|---|---|---|---|
Mammals | ||||
Red fox (V. vulpes) | Habitat and ecology: Urban or anthropized environments, peri-urban areas, rural or agricultural landscapes, and natural forestland. Opportunistic omnivores and scavengers, moving between natural, agricultural, and urban environments, where they feed on both synanthropic prey species and scavenge in human refuse.Transmission: Their role as generalists means they encounter diverse bacteria from different sources, including those from human waste and agricultural runoff, increasing exposure to antibiotic residues and ARB from humans and the food chain.Dissemination: Daily movement across landscapes may spread ARB between different suburban and urban spaces, and agricultural land, facilitating AMR transfer between these systems. Commonly come into contact with domestic pets and their food, hence indirectly with humans. ARB can thus enter the household, posing a potential public health risk. | Sampling: Highly mobile animals, making live capture more challenging. However, due to their widespread geographic distribution and human proximity, non-invasive faecal sampling is relatively easy, allowing for straightforward, broad spatial monitoring coverage. Carcass sampling from roadkill is also suitable as tissue sampling (e.g. liver/kidney) can reveal accumulated antimicrobial compounds or ARB.Bioindicator: Foxes forage in urban, peri-urban, and farming landscapes, where antimicrobials are often present. Their interaction with these human-modified habitats contributes to their suitability as relevant bioindicators of AMR in anthropogenic and agricultural environments. | Ongoing schemes: The European Food Safety Authority conducts annual monitoring for Ec. multilocularis in red foxes to assess zoonotic risk across Europe (EFSA 2023a). The EU programme for the eradication of rabies is ongoing, in which the effects of the vaccination programme are evaluated by laboratory examinations of randomly hunted foxes (EC 2015). Additionally, the UK Centre for Ecology and Hydrology runs a number of partner schemes such as the Garden Wildlife Health scheme in which the public can send samples of sick or dying wildlife in their garden.Potential for AMR integration: These existing surveillance frameworks could be expanded to include AMR screening as faecal sampling and carcass collection are already involved. | (Asai et al. 2022a); (Asai et al. 2022b); (Botti et al. 2013); (Carella et al. 2022); (Carson et al. 2012); (Chiaverini et al. 2022); (Costa et al. 2006); (Cristovao et al. 2017); (Dec et al. 2020); (Dias et al. 2022a); (Dias et al. 2022b); (Gambino et al. 2021); (Garcia et al. 2020); (Garcia et al. 2022); (Haenni et al. 2020); (Kaspersen et al. 2018); (Kaspersen et al. 2020); (Literak et al. 2009); (Maksimovic et al. 2022); (Mo et al. 2018); (Monecke et al. 2016); (Nowakiewicz et al. 2016); (O’Hagan et al. 2021); (Osinska et al. 2022); (Radhouani et al. 2011); (Radhouani et al. 2012); (Radhouani et al. 2013); (Skarzynska et al. 2020); (Turchi et al. 2019); (Uelze et al. 2021). |
Eurasian otter (Lutra lutra) | Habitat and ecology: Aquatic environments such as freshwater rivers and lakes, often in suburban landscapes, and coastal estuaries, where they feed and bathe.Transmission: These aquatic habitats are common endpoints for agricultural runoff, sewage discharge, and industrial waste, hence are reservoirs for antibiotic metabolites, ARB and ARGs.Additionally, as apex predators, prey consists mainly of fish, crustaceans, and amphibians, meaning otters bioaccumulate contaminants, including heavy metals, pesticides, and ARB over time.Dissemination: Often found in interconnected waterways and are a highly mobile species. This potentially leads to direct faecal contamination into previously unpolluted water bodies, sediment, and surrounding soil, facilitating the movement of different strains of ARB across habitats | Sampling: Spraint collection near rivers and waterways is relatively easy. The otter’s high dependence on aquatic ecosystems also makes them suitable for water quality monitoring. Tissue sampling can also be carried out from roadkill or dead individuals found during routine conservation monitoring, offering an effective non-invasive method.Bioindicator: They could be effective bioindicators of long-term trends in environmental contamination and AMR presence and prevalence temporally and spatially across trophic levels in the aquatic ecosystem. | Ongoing schemes: Otter spraints are often monitored for conservation purposes and for contaminants, including heavy metals and organic pollutants, through national and European conservation programmes, such as the Irish National Otter Survey 2023–2024, and the Otter Project, run by Cardiff University, UK. Otters’ spraint and roadkill carcass collection is already a common non-invasive method to assess population health.Potential for AMR integration: These existing schemes can be expanded to include AMR surveillance in aquatic environments. Adding AMR testing to spraint samples from riverbanks and other aquatic habitats could provide non-invasive insights into waterborne ARB. | (Alonso et al. 2017); (Dias et al. 2022b); (Dias et al. 2022c); (Garcia et al. 2020); (Garcia et al. 2022); (Kumari et al. 2022); (Loncaric et al. 2013); (Mengistu et al. 2022); (Oliveira et al. 2010); (Semedo-Lemsaddek et al. 2013); (Semedo-Lemsaddek et al. 2018). |
Rats (Rattus spp.) | Habitat and ecology: Urban or anthropized environments, peri-urban areas, rural, or agricultural landscapes. Behave as omnivorous scavengers, accessing animal feed and feeding on human refuse.Transmission: Often exposed to high levels of ARB and antibiotic metabolites in agricultural run-off, sewage systems, and landfills.Dissemination: Known carriers of zoonotic pathogens and resistant bacteria. Could potentially disseminate anthropogenic-associated resistant bacteria through their faeces, urine, and direct contact with humans, livestock, and domestic animals. | Sampling: Near-global distribution and thrive in human-modified environments. They are easy to trap, and their faeces are abundant, meaning sampling can be done frequently in areas of interest, allowing for extensive surveillance in cities, peri-urban areas, and agricultural settings.Bioindicator: Given their close association with human settlements and their scavenging behaviour, rats may be the ideal sentinel for urban, human-associated AMR. | Ongoing schemes: Urban rat populations are frequently monitored for zoonotic diseases, particularly in large cities by public health bodies. The CDC, EPA and local municipal bodies run rodent control programmes that often include pathogen surveillance (Brown and Laco 2015, EPA 2024)Potential for AMR integration: Rat faeces could be routinely tested for ARB in urban monitoring programmes that already track rodent-borne diseases like strains of Rickettsia and Bartonella. | (Asai et al. 2020); (Azimi et al. 2021); (Bamunusinghage et al. 2022); (Blanco Crivelli et al. 2018); (Desvars-Larrive et al. 2019); (Dominguez et al. 2023) (Ge et al. 2019); (Gomez et al. 2014); (Guenther et al. 2012); (Guyomard-Rabenirina et al. 2020); (Hansen et al. 2016); (Himsworth et al. 2014); (Himsworth et al. 2015); (Himsworth et al. 2016); (LeHuy et al. 2020); (Lozano et al. 2015); (Nhung et al. 2015); (Raafat et al. 2020) (Sano et al. 2023); (Schaufler et al. 2018); (van de Giessen et al. 2009). |
Deer species (Cervidae) | Habitat and ecology: Reside in forests, grasslands, peri-domestic areas, and agricultural landscapes. They are herbivorous, feeding on crops, silage, and animal feed.Transmission: Potentially exposed to agricultural ARB through consumption of treated crops or contaminated water.Dissemination: May transfer resistant bacteria from peri-domestic areas to agricultural soils and water sources and vice-versa, through their faeces. | Sampling: Large, common, and widespread, hence relatively easy to monitor. Often hunted for recreation and routine wildlife management, offering opportunity for the creation collection schemes for faeces, tissue or blood from hunted game carcasses, creating a passive means of tracking resistance trends.Bioindicator: Deer’s frequent foraging near agricultural land make them valuable sentinels for AMR in areas where runoff and antibiotics may be present. | Ongoing schemes: Deer populations are controlled by national deer culling and carcass collection programmes (e.g. Irish Deer Management Strategy Group 2023). Deer populations are also regularly monitored in Europe and North America for diseases such as chronic wasting disease and bTB (e.g. EFSA 2023b), and are commonly monitored in academic studies, to provide empirical bases for deer management (e.g. Keenan et al. 2023, SMARTDEER project).Potential for AMR integration: Routine population management, including culling, hunting seasons, and wildlife health assessments, provides distinct, straightforward opportunities for sampling and AMR monitoring using faeces or tissue samples. | (Alonso et al. 2016); (Alonso et al. 2017); (Asai et al. 2020); (Ballash et al. 2021); (Ballash et al. 2022); (Carroll et al. 2015); (Chiaverini et al. 2022); (Dias et al. 2015); (Dias et al. 2022b); (Dias et al. 2022d); (Dias et al. 2022e); (Elsby et al. 2022); (Gambino et al. 2021); (Garcia et al. 2020); (Homeier-Bachmann et al. 2022); (Plaza-Rodriguez et al. 2020); (Ramos et al. 2022); (Rogers et al. 2018); (Sarker et al. 2019); (Smith et al. 2014); (Smoglica et al. 2023); (Torres et al. 2021). |
Wild boar (Sus scrofa) | Habitat and ecology: Agricultural landscapes, peri-urban areas, forest, and landfills. An omnivorous and highly adaptable diet, eating vegetation, insects, small mammals, ground-nesting birds, amphibians, and carrion. They scavenge and forage in both natural and agricultural environments.Transmission: They are referred to as ecosystem engineers as they are often seen rooting in soil for food. This rooting behaviour can disturb soil and soil processes, leading to bacterial exchange and thereby increasing their exposure to ARB in manure-fertilized fields. They are also feeding on animals residing in the agricultural and peri-urban landscape, and therefore may be acquiring and maintaining ARB through trophic levels.Dissemination: They have the potential for direct and indirect contact with humans and livestock which can facilitate the exchange of ARB, making them effective indicators of AMR in areas impacted by human activity, particularly in soil. | Sampling: Widespread across Europe and increasingly encroach on agricultural and urban areas, providing opportunities to monitor AMR in various environments. Often hunted for management, providing opportunities to collect biological samples such as tissues, blood, and faeces from carcasses for AMR surveillance.Bioindicator: As culling schemes are ongoing and as faecal sampling can also be done non-invasively in areas where they forage, they are a practical choice for longitudinal monitoring programmes. | Ongoing schemes: Wild boar are considered invasive in some countries hence populations are culled for management (e.g. DEFRA 2008). They are also included in general zoonoses monitoring programmes based on Directive 2003/99/EC (e.g. the German National Zoonoses Monitoring Programme, Plaza-Rodriguez et al. 2020).Wild boar are also subject to specific wildlife disease surveillance programmes, particularly for African swine fever (e.g. EFSA 2018, Guberti et al. 2019) where urine, faeces, and carcasses are routinely tested.Potential for AMR integration: These monitoring programmes, which involve tissue and blood sampling during routine hunting and culling, could be expanded to include AMR testing. | (Asai et al. 2020); (Bachiri et al. 2018); (Chiaverini et al. 2022); (Cristovao et al. 2017); (Dias et al. 2022b); (Dias et al. 2022f); (Gambino et al. 2021); (Garcia et al. 2020); (Garcia et al. 2022); (Gunther et al. 2022); (Homeier-Bachmann et al. 2022); (Lee et al. 2022); (Literak et al. 2009); (Mercato et al. 2022) (Navarro-Gonzalez et al. 2018); (Plaza-Rodriguez et al. 2020); (Ramos et al. 2022); (Rega et al. 2022); (Skarzynska et al. 2020); (Torres et al. 2020b); (Wasyl et al. 2018). |
Birds | ||||
Gulls (Laridae) | Habitat and ecology: Wetlands, agricultural lands, coastal regions, and urban areas. They are opportunistic scavengers and often feed on human waste in city centres, landfills, sewage plants, alongside agricultural fields.Transmission: Feeding in these diverse environments places them in direct contact with ARB and antimicrobial metabolites, thereby increasing their risk of acquiring AMR.Dissemination: Migratory birds can acquire ARB in one region and transport to another, potentially introducing new ARGs across continents, linking distant ecosystems. With this, their faeces can contaminate recreational waters, beaches, and into densely human-populated urban areas, posing a risk to public health. Moreover, as they congregate in large flocks during migration and at breeding grounds, the exchange of ARB and ARGs to resident bird species and aquatic organisms is accelerated. | Sampling: Gulls are highly mobile, making faecal collection from loafing sites and feeding areas a straightforward, efficient and non-invasive method to sample for resistant bacteria.Bioindicator: They are common in coastal and agricultural environments, and frequent interaction with humans and human waste, which makes them important for bioindicators of AMR in urban areas affected by human activities and water pollution. | Ongoing schemes: Gulls are included in avian influenza surveillance programmes (EFSA et al. 2024), involving faecal and water sampling to assess disease presence in migratory bird populations. Moreover, the Predatory Bird Monitoring Scheme is ongoing in the UK, in which the general public can send in bird carcasses to analyse for pollutants and contaminants. As the infrastructure is already available, gull monitoring would be relatively straightforward to include in this scheme.Potential for AMR integration: Faeces collected for avian influenza could be screened for AMR. This would be particularly useful if results were integrated into tracking data for the birds (e.g. BirdWatch Ireland gull tracking programmes), allowing for higher resolution spatial tracking of ARB spread over time. | (Ahlstrom et al. 2018); (Ahlstrom et al. 2021); (Ahlstrom et al. 2022); (Atterby et al. 2017); (Bonnedahl et al. 2009); (Bonnedahl et al. 2010); (Carroll et al. 2015); (Dolejska et al. 2007); (Dolejska et al. 2016); (Gambino et al. 2021); (Guenther et al. 2010); (Hernandez et al. 2010); (Jarma et al. 2021); (Liakopoulos et al. 2016); (Literak et al. 2010); (Nelson et al. 2008); (Poeta et al. 2008); (Simoes et al. 2010); (Sjolund et al. 2008); (Smith et al. 2014); (Stedt et al. 2014); (Stedt et al. 2015); (Vittecoq et al. 2016b). |
Waterfowl (Anseriformes) | Habitat and ecology: Wetlands, relying on them for food, shelter, and breeding. Many species have adapted to, and thrive in anthropogenic and agricultural environments, where they interact with urban and farming wastewater systems. Opportunistic feeders, including aquatic plants and invertebrates, and forage in agricultural fields especially during migration or winter.Transmission: Wetlands can act as reservoirs for ARB, which waterfowl may acquire. They may also move between habitats, exposing them to other anthropogenic sources of AMR, such as manure runoff, antibiotic metabolites, and pesticides. Some waterfowl species nest near human activities, such as in ponds or reservoirs near wastewater discharge, increasing their exposure to pollutants and resistant bacteria. Waterfowl are social, and form large, occasionally mixed-species flocks (other waterbirds such as gulls or waders), particularly during migration, wintering, and at feeding sites. This behaviour may increase the likelihood of (cross-species) ARB transmission through direct contact or faecal contamination.Dissemination: They migrate large distances, during which large flocks will use stopover sites that can become hotspots for ARB exchange within the flock and to resident bird colonies and aquatic organisms. This in turn contributes to the local spread of ARB in a previously naïve ecosystem. | Sampling: Faecal sampling, along with agricultural and urban water and sediment sampling from wetlands, lakes, and rivers where waterfowl congregate can be used to monitor AMR in aquatic environments. Water and sediment samples from these areas can also be tested for resistant bacteria.Bioindicator: Waterfowl are widespread, particularly in freshwater wetlands and coastal areas, providing extensive coverage for monitoring AMR in aquatic systems. Their movement between natural and agricultural environments exposes them to antibiotics from agricultural runoff, making them good bioindicators of AMR in aquatic systems. | Ongoing schemes: Waterfowl are included in avian influenza surveillance programmes (EFSA et al. 2024), involving faecal and water sampling to assess disease presence in bird populations. They are also included in zoonoses monitoring programmes based on Directive 2003/99/EC (e.g. the German National Zoonoses Monitoring Programme).Potential for AMR integration: AMR monitoring can be integrated into the existing avian influenza waterfowl surveillance by simply incorporating AMR testing into their current disease tracking schemes. Monitoring can also be integrated into existing wildlife and wetland conservation and tracking programs [e.g. British Trust for Ornithology Wetland Bird Survey (WeBS)] which would be feasible and cost-effective and allow for higher resolution and more meaningful spatial surveillance of ARB spread over time. | (Agnew et al. 2015); (Ahmed et al. 2019); (Cole et al. 2005); (Gambino et al. 2021); (Guenther et al. 2010); (Jarma et al. 2021); (Literak et al. 2010); (Plaza-Rodriguez et al. 2020); (Sjolund et al. 2008); (Stepien-Pysniak et al. 2019), (Thapaliya et al. 2017). |
AMR, antimicrobial resistance; ARB, antimicrobial resistant bacteria; ARGs, antimicrobial resistance gene; and bTB, bovine tuberculosis.
Case studies for possible sentinel species highlighting ecological rationale for sentinel use, and ongoing surveillance schemes that hold potential to be utilized for resistance monitoring, with examples of academic studies investigating presence of in the species.
Species . | Ecological aspects for sentinel use . | Monitoring feasibility . | Potential synergistic schemes . | Publications . |
---|---|---|---|---|
Mammals | ||||
Red fox (V. vulpes) | Habitat and ecology: Urban or anthropized environments, peri-urban areas, rural or agricultural landscapes, and natural forestland. Opportunistic omnivores and scavengers, moving between natural, agricultural, and urban environments, where they feed on both synanthropic prey species and scavenge in human refuse.Transmission: Their role as generalists means they encounter diverse bacteria from different sources, including those from human waste and agricultural runoff, increasing exposure to antibiotic residues and ARB from humans and the food chain.Dissemination: Daily movement across landscapes may spread ARB between different suburban and urban spaces, and agricultural land, facilitating AMR transfer between these systems. Commonly come into contact with domestic pets and their food, hence indirectly with humans. ARB can thus enter the household, posing a potential public health risk. | Sampling: Highly mobile animals, making live capture more challenging. However, due to their widespread geographic distribution and human proximity, non-invasive faecal sampling is relatively easy, allowing for straightforward, broad spatial monitoring coverage. Carcass sampling from roadkill is also suitable as tissue sampling (e.g. liver/kidney) can reveal accumulated antimicrobial compounds or ARB.Bioindicator: Foxes forage in urban, peri-urban, and farming landscapes, where antimicrobials are often present. Their interaction with these human-modified habitats contributes to their suitability as relevant bioindicators of AMR in anthropogenic and agricultural environments. | Ongoing schemes: The European Food Safety Authority conducts annual monitoring for Ec. multilocularis in red foxes to assess zoonotic risk across Europe (EFSA 2023a). The EU programme for the eradication of rabies is ongoing, in which the effects of the vaccination programme are evaluated by laboratory examinations of randomly hunted foxes (EC 2015). Additionally, the UK Centre for Ecology and Hydrology runs a number of partner schemes such as the Garden Wildlife Health scheme in which the public can send samples of sick or dying wildlife in their garden.Potential for AMR integration: These existing surveillance frameworks could be expanded to include AMR screening as faecal sampling and carcass collection are already involved. | (Asai et al. 2022a); (Asai et al. 2022b); (Botti et al. 2013); (Carella et al. 2022); (Carson et al. 2012); (Chiaverini et al. 2022); (Costa et al. 2006); (Cristovao et al. 2017); (Dec et al. 2020); (Dias et al. 2022a); (Dias et al. 2022b); (Gambino et al. 2021); (Garcia et al. 2020); (Garcia et al. 2022); (Haenni et al. 2020); (Kaspersen et al. 2018); (Kaspersen et al. 2020); (Literak et al. 2009); (Maksimovic et al. 2022); (Mo et al. 2018); (Monecke et al. 2016); (Nowakiewicz et al. 2016); (O’Hagan et al. 2021); (Osinska et al. 2022); (Radhouani et al. 2011); (Radhouani et al. 2012); (Radhouani et al. 2013); (Skarzynska et al. 2020); (Turchi et al. 2019); (Uelze et al. 2021). |
Eurasian otter (Lutra lutra) | Habitat and ecology: Aquatic environments such as freshwater rivers and lakes, often in suburban landscapes, and coastal estuaries, where they feed and bathe.Transmission: These aquatic habitats are common endpoints for agricultural runoff, sewage discharge, and industrial waste, hence are reservoirs for antibiotic metabolites, ARB and ARGs.Additionally, as apex predators, prey consists mainly of fish, crustaceans, and amphibians, meaning otters bioaccumulate contaminants, including heavy metals, pesticides, and ARB over time.Dissemination: Often found in interconnected waterways and are a highly mobile species. This potentially leads to direct faecal contamination into previously unpolluted water bodies, sediment, and surrounding soil, facilitating the movement of different strains of ARB across habitats | Sampling: Spraint collection near rivers and waterways is relatively easy. The otter’s high dependence on aquatic ecosystems also makes them suitable for water quality monitoring. Tissue sampling can also be carried out from roadkill or dead individuals found during routine conservation monitoring, offering an effective non-invasive method.Bioindicator: They could be effective bioindicators of long-term trends in environmental contamination and AMR presence and prevalence temporally and spatially across trophic levels in the aquatic ecosystem. | Ongoing schemes: Otter spraints are often monitored for conservation purposes and for contaminants, including heavy metals and organic pollutants, through national and European conservation programmes, such as the Irish National Otter Survey 2023–2024, and the Otter Project, run by Cardiff University, UK. Otters’ spraint and roadkill carcass collection is already a common non-invasive method to assess population health.Potential for AMR integration: These existing schemes can be expanded to include AMR surveillance in aquatic environments. Adding AMR testing to spraint samples from riverbanks and other aquatic habitats could provide non-invasive insights into waterborne ARB. | (Alonso et al. 2017); (Dias et al. 2022b); (Dias et al. 2022c); (Garcia et al. 2020); (Garcia et al. 2022); (Kumari et al. 2022); (Loncaric et al. 2013); (Mengistu et al. 2022); (Oliveira et al. 2010); (Semedo-Lemsaddek et al. 2013); (Semedo-Lemsaddek et al. 2018). |
Rats (Rattus spp.) | Habitat and ecology: Urban or anthropized environments, peri-urban areas, rural, or agricultural landscapes. Behave as omnivorous scavengers, accessing animal feed and feeding on human refuse.Transmission: Often exposed to high levels of ARB and antibiotic metabolites in agricultural run-off, sewage systems, and landfills.Dissemination: Known carriers of zoonotic pathogens and resistant bacteria. Could potentially disseminate anthropogenic-associated resistant bacteria through their faeces, urine, and direct contact with humans, livestock, and domestic animals. | Sampling: Near-global distribution and thrive in human-modified environments. They are easy to trap, and their faeces are abundant, meaning sampling can be done frequently in areas of interest, allowing for extensive surveillance in cities, peri-urban areas, and agricultural settings.Bioindicator: Given their close association with human settlements and their scavenging behaviour, rats may be the ideal sentinel for urban, human-associated AMR. | Ongoing schemes: Urban rat populations are frequently monitored for zoonotic diseases, particularly in large cities by public health bodies. The CDC, EPA and local municipal bodies run rodent control programmes that often include pathogen surveillance (Brown and Laco 2015, EPA 2024)Potential for AMR integration: Rat faeces could be routinely tested for ARB in urban monitoring programmes that already track rodent-borne diseases like strains of Rickettsia and Bartonella. | (Asai et al. 2020); (Azimi et al. 2021); (Bamunusinghage et al. 2022); (Blanco Crivelli et al. 2018); (Desvars-Larrive et al. 2019); (Dominguez et al. 2023) (Ge et al. 2019); (Gomez et al. 2014); (Guenther et al. 2012); (Guyomard-Rabenirina et al. 2020); (Hansen et al. 2016); (Himsworth et al. 2014); (Himsworth et al. 2015); (Himsworth et al. 2016); (LeHuy et al. 2020); (Lozano et al. 2015); (Nhung et al. 2015); (Raafat et al. 2020) (Sano et al. 2023); (Schaufler et al. 2018); (van de Giessen et al. 2009). |
Deer species (Cervidae) | Habitat and ecology: Reside in forests, grasslands, peri-domestic areas, and agricultural landscapes. They are herbivorous, feeding on crops, silage, and animal feed.Transmission: Potentially exposed to agricultural ARB through consumption of treated crops or contaminated water.Dissemination: May transfer resistant bacteria from peri-domestic areas to agricultural soils and water sources and vice-versa, through their faeces. | Sampling: Large, common, and widespread, hence relatively easy to monitor. Often hunted for recreation and routine wildlife management, offering opportunity for the creation collection schemes for faeces, tissue or blood from hunted game carcasses, creating a passive means of tracking resistance trends.Bioindicator: Deer’s frequent foraging near agricultural land make them valuable sentinels for AMR in areas where runoff and antibiotics may be present. | Ongoing schemes: Deer populations are controlled by national deer culling and carcass collection programmes (e.g. Irish Deer Management Strategy Group 2023). Deer populations are also regularly monitored in Europe and North America for diseases such as chronic wasting disease and bTB (e.g. EFSA 2023b), and are commonly monitored in academic studies, to provide empirical bases for deer management (e.g. Keenan et al. 2023, SMARTDEER project).Potential for AMR integration: Routine population management, including culling, hunting seasons, and wildlife health assessments, provides distinct, straightforward opportunities for sampling and AMR monitoring using faeces or tissue samples. | (Alonso et al. 2016); (Alonso et al. 2017); (Asai et al. 2020); (Ballash et al. 2021); (Ballash et al. 2022); (Carroll et al. 2015); (Chiaverini et al. 2022); (Dias et al. 2015); (Dias et al. 2022b); (Dias et al. 2022d); (Dias et al. 2022e); (Elsby et al. 2022); (Gambino et al. 2021); (Garcia et al. 2020); (Homeier-Bachmann et al. 2022); (Plaza-Rodriguez et al. 2020); (Ramos et al. 2022); (Rogers et al. 2018); (Sarker et al. 2019); (Smith et al. 2014); (Smoglica et al. 2023); (Torres et al. 2021). |
Wild boar (Sus scrofa) | Habitat and ecology: Agricultural landscapes, peri-urban areas, forest, and landfills. An omnivorous and highly adaptable diet, eating vegetation, insects, small mammals, ground-nesting birds, amphibians, and carrion. They scavenge and forage in both natural and agricultural environments.Transmission: They are referred to as ecosystem engineers as they are often seen rooting in soil for food. This rooting behaviour can disturb soil and soil processes, leading to bacterial exchange and thereby increasing their exposure to ARB in manure-fertilized fields. They are also feeding on animals residing in the agricultural and peri-urban landscape, and therefore may be acquiring and maintaining ARB through trophic levels.Dissemination: They have the potential for direct and indirect contact with humans and livestock which can facilitate the exchange of ARB, making them effective indicators of AMR in areas impacted by human activity, particularly in soil. | Sampling: Widespread across Europe and increasingly encroach on agricultural and urban areas, providing opportunities to monitor AMR in various environments. Often hunted for management, providing opportunities to collect biological samples such as tissues, blood, and faeces from carcasses for AMR surveillance.Bioindicator: As culling schemes are ongoing and as faecal sampling can also be done non-invasively in areas where they forage, they are a practical choice for longitudinal monitoring programmes. | Ongoing schemes: Wild boar are considered invasive in some countries hence populations are culled for management (e.g. DEFRA 2008). They are also included in general zoonoses monitoring programmes based on Directive 2003/99/EC (e.g. the German National Zoonoses Monitoring Programme, Plaza-Rodriguez et al. 2020).Wild boar are also subject to specific wildlife disease surveillance programmes, particularly for African swine fever (e.g. EFSA 2018, Guberti et al. 2019) where urine, faeces, and carcasses are routinely tested.Potential for AMR integration: These monitoring programmes, which involve tissue and blood sampling during routine hunting and culling, could be expanded to include AMR testing. | (Asai et al. 2020); (Bachiri et al. 2018); (Chiaverini et al. 2022); (Cristovao et al. 2017); (Dias et al. 2022b); (Dias et al. 2022f); (Gambino et al. 2021); (Garcia et al. 2020); (Garcia et al. 2022); (Gunther et al. 2022); (Homeier-Bachmann et al. 2022); (Lee et al. 2022); (Literak et al. 2009); (Mercato et al. 2022) (Navarro-Gonzalez et al. 2018); (Plaza-Rodriguez et al. 2020); (Ramos et al. 2022); (Rega et al. 2022); (Skarzynska et al. 2020); (Torres et al. 2020b); (Wasyl et al. 2018). |
Birds | ||||
Gulls (Laridae) | Habitat and ecology: Wetlands, agricultural lands, coastal regions, and urban areas. They are opportunistic scavengers and often feed on human waste in city centres, landfills, sewage plants, alongside agricultural fields.Transmission: Feeding in these diverse environments places them in direct contact with ARB and antimicrobial metabolites, thereby increasing their risk of acquiring AMR.Dissemination: Migratory birds can acquire ARB in one region and transport to another, potentially introducing new ARGs across continents, linking distant ecosystems. With this, their faeces can contaminate recreational waters, beaches, and into densely human-populated urban areas, posing a risk to public health. Moreover, as they congregate in large flocks during migration and at breeding grounds, the exchange of ARB and ARGs to resident bird species and aquatic organisms is accelerated. | Sampling: Gulls are highly mobile, making faecal collection from loafing sites and feeding areas a straightforward, efficient and non-invasive method to sample for resistant bacteria.Bioindicator: They are common in coastal and agricultural environments, and frequent interaction with humans and human waste, which makes them important for bioindicators of AMR in urban areas affected by human activities and water pollution. | Ongoing schemes: Gulls are included in avian influenza surveillance programmes (EFSA et al. 2024), involving faecal and water sampling to assess disease presence in migratory bird populations. Moreover, the Predatory Bird Monitoring Scheme is ongoing in the UK, in which the general public can send in bird carcasses to analyse for pollutants and contaminants. As the infrastructure is already available, gull monitoring would be relatively straightforward to include in this scheme.Potential for AMR integration: Faeces collected for avian influenza could be screened for AMR. This would be particularly useful if results were integrated into tracking data for the birds (e.g. BirdWatch Ireland gull tracking programmes), allowing for higher resolution spatial tracking of ARB spread over time. | (Ahlstrom et al. 2018); (Ahlstrom et al. 2021); (Ahlstrom et al. 2022); (Atterby et al. 2017); (Bonnedahl et al. 2009); (Bonnedahl et al. 2010); (Carroll et al. 2015); (Dolejska et al. 2007); (Dolejska et al. 2016); (Gambino et al. 2021); (Guenther et al. 2010); (Hernandez et al. 2010); (Jarma et al. 2021); (Liakopoulos et al. 2016); (Literak et al. 2010); (Nelson et al. 2008); (Poeta et al. 2008); (Simoes et al. 2010); (Sjolund et al. 2008); (Smith et al. 2014); (Stedt et al. 2014); (Stedt et al. 2015); (Vittecoq et al. 2016b). |
Waterfowl (Anseriformes) | Habitat and ecology: Wetlands, relying on them for food, shelter, and breeding. Many species have adapted to, and thrive in anthropogenic and agricultural environments, where they interact with urban and farming wastewater systems. Opportunistic feeders, including aquatic plants and invertebrates, and forage in agricultural fields especially during migration or winter.Transmission: Wetlands can act as reservoirs for ARB, which waterfowl may acquire. They may also move between habitats, exposing them to other anthropogenic sources of AMR, such as manure runoff, antibiotic metabolites, and pesticides. Some waterfowl species nest near human activities, such as in ponds or reservoirs near wastewater discharge, increasing their exposure to pollutants and resistant bacteria. Waterfowl are social, and form large, occasionally mixed-species flocks (other waterbirds such as gulls or waders), particularly during migration, wintering, and at feeding sites. This behaviour may increase the likelihood of (cross-species) ARB transmission through direct contact or faecal contamination.Dissemination: They migrate large distances, during which large flocks will use stopover sites that can become hotspots for ARB exchange within the flock and to resident bird colonies and aquatic organisms. This in turn contributes to the local spread of ARB in a previously naïve ecosystem. | Sampling: Faecal sampling, along with agricultural and urban water and sediment sampling from wetlands, lakes, and rivers where waterfowl congregate can be used to monitor AMR in aquatic environments. Water and sediment samples from these areas can also be tested for resistant bacteria.Bioindicator: Waterfowl are widespread, particularly in freshwater wetlands and coastal areas, providing extensive coverage for monitoring AMR in aquatic systems. Their movement between natural and agricultural environments exposes them to antibiotics from agricultural runoff, making them good bioindicators of AMR in aquatic systems. | Ongoing schemes: Waterfowl are included in avian influenza surveillance programmes (EFSA et al. 2024), involving faecal and water sampling to assess disease presence in bird populations. They are also included in zoonoses monitoring programmes based on Directive 2003/99/EC (e.g. the German National Zoonoses Monitoring Programme).Potential for AMR integration: AMR monitoring can be integrated into the existing avian influenza waterfowl surveillance by simply incorporating AMR testing into their current disease tracking schemes. Monitoring can also be integrated into existing wildlife and wetland conservation and tracking programs [e.g. British Trust for Ornithology Wetland Bird Survey (WeBS)] which would be feasible and cost-effective and allow for higher resolution and more meaningful spatial surveillance of ARB spread over time. | (Agnew et al. 2015); (Ahmed et al. 2019); (Cole et al. 2005); (Gambino et al. 2021); (Guenther et al. 2010); (Jarma et al. 2021); (Literak et al. 2010); (Plaza-Rodriguez et al. 2020); (Sjolund et al. 2008); (Stepien-Pysniak et al. 2019), (Thapaliya et al. 2017). |
Species . | Ecological aspects for sentinel use . | Monitoring feasibility . | Potential synergistic schemes . | Publications . |
---|---|---|---|---|
Mammals | ||||
Red fox (V. vulpes) | Habitat and ecology: Urban or anthropized environments, peri-urban areas, rural or agricultural landscapes, and natural forestland. Opportunistic omnivores and scavengers, moving between natural, agricultural, and urban environments, where they feed on both synanthropic prey species and scavenge in human refuse.Transmission: Their role as generalists means they encounter diverse bacteria from different sources, including those from human waste and agricultural runoff, increasing exposure to antibiotic residues and ARB from humans and the food chain.Dissemination: Daily movement across landscapes may spread ARB between different suburban and urban spaces, and agricultural land, facilitating AMR transfer between these systems. Commonly come into contact with domestic pets and their food, hence indirectly with humans. ARB can thus enter the household, posing a potential public health risk. | Sampling: Highly mobile animals, making live capture more challenging. However, due to their widespread geographic distribution and human proximity, non-invasive faecal sampling is relatively easy, allowing for straightforward, broad spatial monitoring coverage. Carcass sampling from roadkill is also suitable as tissue sampling (e.g. liver/kidney) can reveal accumulated antimicrobial compounds or ARB.Bioindicator: Foxes forage in urban, peri-urban, and farming landscapes, where antimicrobials are often present. Their interaction with these human-modified habitats contributes to their suitability as relevant bioindicators of AMR in anthropogenic and agricultural environments. | Ongoing schemes: The European Food Safety Authority conducts annual monitoring for Ec. multilocularis in red foxes to assess zoonotic risk across Europe (EFSA 2023a). The EU programme for the eradication of rabies is ongoing, in which the effects of the vaccination programme are evaluated by laboratory examinations of randomly hunted foxes (EC 2015). Additionally, the UK Centre for Ecology and Hydrology runs a number of partner schemes such as the Garden Wildlife Health scheme in which the public can send samples of sick or dying wildlife in their garden.Potential for AMR integration: These existing surveillance frameworks could be expanded to include AMR screening as faecal sampling and carcass collection are already involved. | (Asai et al. 2022a); (Asai et al. 2022b); (Botti et al. 2013); (Carella et al. 2022); (Carson et al. 2012); (Chiaverini et al. 2022); (Costa et al. 2006); (Cristovao et al. 2017); (Dec et al. 2020); (Dias et al. 2022a); (Dias et al. 2022b); (Gambino et al. 2021); (Garcia et al. 2020); (Garcia et al. 2022); (Haenni et al. 2020); (Kaspersen et al. 2018); (Kaspersen et al. 2020); (Literak et al. 2009); (Maksimovic et al. 2022); (Mo et al. 2018); (Monecke et al. 2016); (Nowakiewicz et al. 2016); (O’Hagan et al. 2021); (Osinska et al. 2022); (Radhouani et al. 2011); (Radhouani et al. 2012); (Radhouani et al. 2013); (Skarzynska et al. 2020); (Turchi et al. 2019); (Uelze et al. 2021). |
Eurasian otter (Lutra lutra) | Habitat and ecology: Aquatic environments such as freshwater rivers and lakes, often in suburban landscapes, and coastal estuaries, where they feed and bathe.Transmission: These aquatic habitats are common endpoints for agricultural runoff, sewage discharge, and industrial waste, hence are reservoirs for antibiotic metabolites, ARB and ARGs.Additionally, as apex predators, prey consists mainly of fish, crustaceans, and amphibians, meaning otters bioaccumulate contaminants, including heavy metals, pesticides, and ARB over time.Dissemination: Often found in interconnected waterways and are a highly mobile species. This potentially leads to direct faecal contamination into previously unpolluted water bodies, sediment, and surrounding soil, facilitating the movement of different strains of ARB across habitats | Sampling: Spraint collection near rivers and waterways is relatively easy. The otter’s high dependence on aquatic ecosystems also makes them suitable for water quality monitoring. Tissue sampling can also be carried out from roadkill or dead individuals found during routine conservation monitoring, offering an effective non-invasive method.Bioindicator: They could be effective bioindicators of long-term trends in environmental contamination and AMR presence and prevalence temporally and spatially across trophic levels in the aquatic ecosystem. | Ongoing schemes: Otter spraints are often monitored for conservation purposes and for contaminants, including heavy metals and organic pollutants, through national and European conservation programmes, such as the Irish National Otter Survey 2023–2024, and the Otter Project, run by Cardiff University, UK. Otters’ spraint and roadkill carcass collection is already a common non-invasive method to assess population health.Potential for AMR integration: These existing schemes can be expanded to include AMR surveillance in aquatic environments. Adding AMR testing to spraint samples from riverbanks and other aquatic habitats could provide non-invasive insights into waterborne ARB. | (Alonso et al. 2017); (Dias et al. 2022b); (Dias et al. 2022c); (Garcia et al. 2020); (Garcia et al. 2022); (Kumari et al. 2022); (Loncaric et al. 2013); (Mengistu et al. 2022); (Oliveira et al. 2010); (Semedo-Lemsaddek et al. 2013); (Semedo-Lemsaddek et al. 2018). |
Rats (Rattus spp.) | Habitat and ecology: Urban or anthropized environments, peri-urban areas, rural, or agricultural landscapes. Behave as omnivorous scavengers, accessing animal feed and feeding on human refuse.Transmission: Often exposed to high levels of ARB and antibiotic metabolites in agricultural run-off, sewage systems, and landfills.Dissemination: Known carriers of zoonotic pathogens and resistant bacteria. Could potentially disseminate anthropogenic-associated resistant bacteria through their faeces, urine, and direct contact with humans, livestock, and domestic animals. | Sampling: Near-global distribution and thrive in human-modified environments. They are easy to trap, and their faeces are abundant, meaning sampling can be done frequently in areas of interest, allowing for extensive surveillance in cities, peri-urban areas, and agricultural settings.Bioindicator: Given their close association with human settlements and their scavenging behaviour, rats may be the ideal sentinel for urban, human-associated AMR. | Ongoing schemes: Urban rat populations are frequently monitored for zoonotic diseases, particularly in large cities by public health bodies. The CDC, EPA and local municipal bodies run rodent control programmes that often include pathogen surveillance (Brown and Laco 2015, EPA 2024)Potential for AMR integration: Rat faeces could be routinely tested for ARB in urban monitoring programmes that already track rodent-borne diseases like strains of Rickettsia and Bartonella. | (Asai et al. 2020); (Azimi et al. 2021); (Bamunusinghage et al. 2022); (Blanco Crivelli et al. 2018); (Desvars-Larrive et al. 2019); (Dominguez et al. 2023) (Ge et al. 2019); (Gomez et al. 2014); (Guenther et al. 2012); (Guyomard-Rabenirina et al. 2020); (Hansen et al. 2016); (Himsworth et al. 2014); (Himsworth et al. 2015); (Himsworth et al. 2016); (LeHuy et al. 2020); (Lozano et al. 2015); (Nhung et al. 2015); (Raafat et al. 2020) (Sano et al. 2023); (Schaufler et al. 2018); (van de Giessen et al. 2009). |
Deer species (Cervidae) | Habitat and ecology: Reside in forests, grasslands, peri-domestic areas, and agricultural landscapes. They are herbivorous, feeding on crops, silage, and animal feed.Transmission: Potentially exposed to agricultural ARB through consumption of treated crops or contaminated water.Dissemination: May transfer resistant bacteria from peri-domestic areas to agricultural soils and water sources and vice-versa, through their faeces. | Sampling: Large, common, and widespread, hence relatively easy to monitor. Often hunted for recreation and routine wildlife management, offering opportunity for the creation collection schemes for faeces, tissue or blood from hunted game carcasses, creating a passive means of tracking resistance trends.Bioindicator: Deer’s frequent foraging near agricultural land make them valuable sentinels for AMR in areas where runoff and antibiotics may be present. | Ongoing schemes: Deer populations are controlled by national deer culling and carcass collection programmes (e.g. Irish Deer Management Strategy Group 2023). Deer populations are also regularly monitored in Europe and North America for diseases such as chronic wasting disease and bTB (e.g. EFSA 2023b), and are commonly monitored in academic studies, to provide empirical bases for deer management (e.g. Keenan et al. 2023, SMARTDEER project).Potential for AMR integration: Routine population management, including culling, hunting seasons, and wildlife health assessments, provides distinct, straightforward opportunities for sampling and AMR monitoring using faeces or tissue samples. | (Alonso et al. 2016); (Alonso et al. 2017); (Asai et al. 2020); (Ballash et al. 2021); (Ballash et al. 2022); (Carroll et al. 2015); (Chiaverini et al. 2022); (Dias et al. 2015); (Dias et al. 2022b); (Dias et al. 2022d); (Dias et al. 2022e); (Elsby et al. 2022); (Gambino et al. 2021); (Garcia et al. 2020); (Homeier-Bachmann et al. 2022); (Plaza-Rodriguez et al. 2020); (Ramos et al. 2022); (Rogers et al. 2018); (Sarker et al. 2019); (Smith et al. 2014); (Smoglica et al. 2023); (Torres et al. 2021). |
Wild boar (Sus scrofa) | Habitat and ecology: Agricultural landscapes, peri-urban areas, forest, and landfills. An omnivorous and highly adaptable diet, eating vegetation, insects, small mammals, ground-nesting birds, amphibians, and carrion. They scavenge and forage in both natural and agricultural environments.Transmission: They are referred to as ecosystem engineers as they are often seen rooting in soil for food. This rooting behaviour can disturb soil and soil processes, leading to bacterial exchange and thereby increasing their exposure to ARB in manure-fertilized fields. They are also feeding on animals residing in the agricultural and peri-urban landscape, and therefore may be acquiring and maintaining ARB through trophic levels.Dissemination: They have the potential for direct and indirect contact with humans and livestock which can facilitate the exchange of ARB, making them effective indicators of AMR in areas impacted by human activity, particularly in soil. | Sampling: Widespread across Europe and increasingly encroach on agricultural and urban areas, providing opportunities to monitor AMR in various environments. Often hunted for management, providing opportunities to collect biological samples such as tissues, blood, and faeces from carcasses for AMR surveillance.Bioindicator: As culling schemes are ongoing and as faecal sampling can also be done non-invasively in areas where they forage, they are a practical choice for longitudinal monitoring programmes. | Ongoing schemes: Wild boar are considered invasive in some countries hence populations are culled for management (e.g. DEFRA 2008). They are also included in general zoonoses monitoring programmes based on Directive 2003/99/EC (e.g. the German National Zoonoses Monitoring Programme, Plaza-Rodriguez et al. 2020).Wild boar are also subject to specific wildlife disease surveillance programmes, particularly for African swine fever (e.g. EFSA 2018, Guberti et al. 2019) where urine, faeces, and carcasses are routinely tested.Potential for AMR integration: These monitoring programmes, which involve tissue and blood sampling during routine hunting and culling, could be expanded to include AMR testing. | (Asai et al. 2020); (Bachiri et al. 2018); (Chiaverini et al. 2022); (Cristovao et al. 2017); (Dias et al. 2022b); (Dias et al. 2022f); (Gambino et al. 2021); (Garcia et al. 2020); (Garcia et al. 2022); (Gunther et al. 2022); (Homeier-Bachmann et al. 2022); (Lee et al. 2022); (Literak et al. 2009); (Mercato et al. 2022) (Navarro-Gonzalez et al. 2018); (Plaza-Rodriguez et al. 2020); (Ramos et al. 2022); (Rega et al. 2022); (Skarzynska et al. 2020); (Torres et al. 2020b); (Wasyl et al. 2018). |
Birds | ||||
Gulls (Laridae) | Habitat and ecology: Wetlands, agricultural lands, coastal regions, and urban areas. They are opportunistic scavengers and often feed on human waste in city centres, landfills, sewage plants, alongside agricultural fields.Transmission: Feeding in these diverse environments places them in direct contact with ARB and antimicrobial metabolites, thereby increasing their risk of acquiring AMR.Dissemination: Migratory birds can acquire ARB in one region and transport to another, potentially introducing new ARGs across continents, linking distant ecosystems. With this, their faeces can contaminate recreational waters, beaches, and into densely human-populated urban areas, posing a risk to public health. Moreover, as they congregate in large flocks during migration and at breeding grounds, the exchange of ARB and ARGs to resident bird species and aquatic organisms is accelerated. | Sampling: Gulls are highly mobile, making faecal collection from loafing sites and feeding areas a straightforward, efficient and non-invasive method to sample for resistant bacteria.Bioindicator: They are common in coastal and agricultural environments, and frequent interaction with humans and human waste, which makes them important for bioindicators of AMR in urban areas affected by human activities and water pollution. | Ongoing schemes: Gulls are included in avian influenza surveillance programmes (EFSA et al. 2024), involving faecal and water sampling to assess disease presence in migratory bird populations. Moreover, the Predatory Bird Monitoring Scheme is ongoing in the UK, in which the general public can send in bird carcasses to analyse for pollutants and contaminants. As the infrastructure is already available, gull monitoring would be relatively straightforward to include in this scheme.Potential for AMR integration: Faeces collected for avian influenza could be screened for AMR. This would be particularly useful if results were integrated into tracking data for the birds (e.g. BirdWatch Ireland gull tracking programmes), allowing for higher resolution spatial tracking of ARB spread over time. | (Ahlstrom et al. 2018); (Ahlstrom et al. 2021); (Ahlstrom et al. 2022); (Atterby et al. 2017); (Bonnedahl et al. 2009); (Bonnedahl et al. 2010); (Carroll et al. 2015); (Dolejska et al. 2007); (Dolejska et al. 2016); (Gambino et al. 2021); (Guenther et al. 2010); (Hernandez et al. 2010); (Jarma et al. 2021); (Liakopoulos et al. 2016); (Literak et al. 2010); (Nelson et al. 2008); (Poeta et al. 2008); (Simoes et al. 2010); (Sjolund et al. 2008); (Smith et al. 2014); (Stedt et al. 2014); (Stedt et al. 2015); (Vittecoq et al. 2016b). |
Waterfowl (Anseriformes) | Habitat and ecology: Wetlands, relying on them for food, shelter, and breeding. Many species have adapted to, and thrive in anthropogenic and agricultural environments, where they interact with urban and farming wastewater systems. Opportunistic feeders, including aquatic plants and invertebrates, and forage in agricultural fields especially during migration or winter.Transmission: Wetlands can act as reservoirs for ARB, which waterfowl may acquire. They may also move between habitats, exposing them to other anthropogenic sources of AMR, such as manure runoff, antibiotic metabolites, and pesticides. Some waterfowl species nest near human activities, such as in ponds or reservoirs near wastewater discharge, increasing their exposure to pollutants and resistant bacteria. Waterfowl are social, and form large, occasionally mixed-species flocks (other waterbirds such as gulls or waders), particularly during migration, wintering, and at feeding sites. This behaviour may increase the likelihood of (cross-species) ARB transmission through direct contact or faecal contamination.Dissemination: They migrate large distances, during which large flocks will use stopover sites that can become hotspots for ARB exchange within the flock and to resident bird colonies and aquatic organisms. This in turn contributes to the local spread of ARB in a previously naïve ecosystem. | Sampling: Faecal sampling, along with agricultural and urban water and sediment sampling from wetlands, lakes, and rivers where waterfowl congregate can be used to monitor AMR in aquatic environments. Water and sediment samples from these areas can also be tested for resistant bacteria.Bioindicator: Waterfowl are widespread, particularly in freshwater wetlands and coastal areas, providing extensive coverage for monitoring AMR in aquatic systems. Their movement between natural and agricultural environments exposes them to antibiotics from agricultural runoff, making them good bioindicators of AMR in aquatic systems. | Ongoing schemes: Waterfowl are included in avian influenza surveillance programmes (EFSA et al. 2024), involving faecal and water sampling to assess disease presence in bird populations. They are also included in zoonoses monitoring programmes based on Directive 2003/99/EC (e.g. the German National Zoonoses Monitoring Programme).Potential for AMR integration: AMR monitoring can be integrated into the existing avian influenza waterfowl surveillance by simply incorporating AMR testing into their current disease tracking schemes. Monitoring can also be integrated into existing wildlife and wetland conservation and tracking programs [e.g. British Trust for Ornithology Wetland Bird Survey (WeBS)] which would be feasible and cost-effective and allow for higher resolution and more meaningful spatial surveillance of ARB spread over time. | (Agnew et al. 2015); (Ahmed et al. 2019); (Cole et al. 2005); (Gambino et al. 2021); (Guenther et al. 2010); (Jarma et al. 2021); (Literak et al. 2010); (Plaza-Rodriguez et al. 2020); (Sjolund et al. 2008); (Stepien-Pysniak et al. 2019), (Thapaliya et al. 2017). |
AMR, antimicrobial resistance; ARB, antimicrobial resistant bacteria; ARGs, antimicrobial resistance gene; and bTB, bovine tuberculosis.
Given the magnitude of knowledge gaps that exist in this field, an array of high-throughput tools and techniques will be necessary to identify and characterize transmission routes of AMR in wildlife to complement the understanding of the ecology of the reservoir species (Arnold et al. 2016). When studying AMR and microbial ecology, these high-throughput culture-independent methods are essential as non-cultivable microbes represent the vast majority of the microbial biodiversity (Enard and Petrov 2018). In fact, conservatively, <1% of environmental bacteria are culturable by standard laboratory techniques (Allen et al. 2010). Despite this cultivation bias, culture methods are common in wildlife AMR studies (Greig et al. 2015, Vittecoq et al. 2016a). Indeed, a review conducted by Abbassi et al. (2022) found that the majority of publications reporting AMR in wildlife utilized classical microbiological techniques of bacterial indicator species of human clinical relevance, and very few focused on the whole resistome. This strategy is likely overlooking species capable of harbouring ARGs, and spreading it by HGT. Broadly speaking, monitoring and surveillance of wildlife for factors that predispose them to AMR carriage could be carried out by applying an analysis pipeline—integrating ecological, biological, and life-history data with culture-independent metagenomic data (Fondi et al. 2016). However, while this would be effective, we would only have access to previously identified resistance genes. In fact, it is likely that the ARGs responsible for phenotypic resistance in wild animals are not detectable when using standard polymerase chain reaction studies set to target common clinical ARGs, implying that there are far more ARGs present in wildlife than we have currently identifiable using current molecular protocols (Arnold et al. 2016).
In order to by-pass this, the future may lie in the field of microbiomics. Microbiomics identifies the constituents of the microbiome, analyses microbial genomes, and determines their influence on disease (Barko et al. 2018). It can be carried out using deeper, metagenomic sequencing techniques such as shotgun metagenomic sequencing. Shotgun metagenomics is the untargeted sequencing of all DNA a sample. It is a valuable technique that can profile the composition of microbial communities to characterize diversity, taxonomic composition, and functional potential, as well as identify potential pathogens and ARGs in a sample (Bengtsson-Palme 2017, Quince et al. 2017, Gand et al. 2024). This offers a broad examination of a range of ARGs genes across taxa of host bacteria within the sample. From a wildlife perspective, shotgun metagenomics could allow researchers to determine differences in AMR profiles of different species and help to elucidate likely causes such as diet, habitat, exposure levels, and behaviour (Skarzynska et al. 2020).
However, the field of metagenomics is not without its practical limitations. Beyond the financial cost of the technology, exploratory shotgun sequencing researchers are faced with a large volume of sequence data needed to adequately garner information on rare ARGs in the sample, meaning data storage and distribution become an issue. The complexity of the bioinformatics may also pose an analysis and interpretation obstacle to researchers unfamiliar with the field (Sharpton 2014). Another obstacle faced by researchers with regards to the bioinformatic analysis are the generally incomplete reference databases that currently exist. These databases may not cover all known ARGs or microbial species. As the accuracy of sequencing relies on comparing sequences to reference databases, incomplete databases may limit detection of low abundance, novel, or rare resistance mechanisms, and hinder strain-level identification (Sharpton 2014, Quince et al. 2017). Despite these current limitations, this shotgun sequencing technology is improving and decreasing in cost constantly. It is a powerful technique that should and ideally will contribute to our understanding of microbiomics and AMR movement in the environment.
OH for a holistic surveillance system
Evidently, there is a need for more interdisciplinary research when gathering data on AMR in wildlife for surveillance purposes. Of course, the ideal global strategy would involve all countries with OH NAPs in place identifying the wildlife and environmental gaps in their strategies and designing and implementing an expanded AMR surveillance system (Benavides et al. 2024). However, no comprehensive OH surveillance system is currently in place in any region to integrate AMR data across humans, livestock, wildlife, and the environment in a single unified framework (OHHLEP—One Health High-Level Expert Panel 2023). Developing such a system would help monitoring bodies to track environmental AMR acquisition and transmission dynamics. We the authors would suggest a holistic and multi-faceted monitoring system for environmental AMR surveillance that employs a OH approach to integrate ecological, landscape, and molecular monitoring perspectives, using wildlife as environmental bioindicators.
First, we would propose AMR surveillance systems that include wildlife bioindicators. These dedicated surveillance programmes should be designed with key sentinel species in mind and prioritize the rational selection of the species based on cost-effectiveness, feasibility, and ecological factors such as habitat, feeding strategies, social behaviour, and migratory patterns (see Table 2). Species that interact with both anthropogenic and agricultural landscapes, such as red foxes, wild boar, gulls, and waterfowl, which already have dedicated surveillance systems in place, are ideal candidate bioindicators, as they can represent the effects of anthropogenic selective pressures across a broad gradient, without adding unnecessary financial and practicality issues to sampling. Undoubtedly, however, a significant hurdle to overcome in this context is the underrepresentation of wildlife in contemporary policy instruments enacted to monitor and mitigate AMR across different environmental compartments. We would suggest that wildlife be explicitly included in existing AMR policy at the national and international level, such as those developed by the WHO, Food and Agriculture Organization, and World Organisation for Animal Health. Nationally, the interdisciplinary OH committees with members representing interests of the environment, agriculture, veterinary, and public health could oversee the policy implementation, and collaborate for broader AMR mitigation strategies.
From an ecological perspective, we propose that environmental impact assessments for anthropogenic land-use changes (e.g. habitat fragmentation, human encroachment, urban expansion, and agricultural intensification) should include risk assessments regarding the AMR risk posed by—and indeed—to wildlife (Mitchell 2023, Nolan et al. 2023). In this regard, policy instruments should also address the use of antimicrobial treatment in wildlife rehabilitation centres. For instance, antimicrobials should only be administered following explicit advice from a veterinarian, and compounds that are deemed critically important for human treatment should not be used. Given the potential for these interventions to drive resistance in the environment, it would be prudent to keep the animals captive following treatment, for a pre-determined timeline to ensure active metabolites are not being excreted before release. Moreover, we would recommend that governmental bodies provide increased resources and funding for research groups to conduct longitudinal sampling and tracking across seasons and migratory routes in order to monitor the spread of AMR geographically. While costly, the use of remote sensing technologies and Global Positioning System (GPS) tracking could help researchers to correlate AMR prevalence with migration data, adding an over-arching spatial and temporal component to AMR surveillance. This would enable researchers to track dissemination routes intercontinentally, across geographic and indeed political borders. Additionally, public-gathered, citizen science sampling strategies could be developed. This data, while interspersed geographically could be useful to track AMR prevalence in areas of close proximity to humans. This data would then be fed into a large-scale, integrated database (see below).
Our third recommendation involves the design of standardized methodologies for surveillance that can be applied across species and environmental compartments to ensure that results are comparable globally. Regarding sampling strategies, practical and non-invasive methods should be employed, ideally utilizing systematic surveillance schemes that are already ongoing (see Table 2). Faecal sampling or environmental DNA analysis can provide insights into how AMR is disseminated via different ecological transmission pathways without harming or interfering with wildlife. Moreover, in order to ensure representative samples and avoid sampling bias (ECDC 2023), the population size of the target species in that country/region should also be estimated, and subsequently the sample size calculated. This will allow for accurate determination of true prevalence and distribution of AMR within wildlife populations, thereby enhancing the comparability of the results, and the overall effectiveness of the monitoring programme. Ideally, these strategies would also include the development of a centralized and standardized reporting platform across geographic regions that could inform future AMR policies and mitigation strategies (OHHLEP—One Health High-Level Expert Panel 2023), and could even serve as an open-access database for academic research to both contribute to and utilize for future proposals and research. An excellent example of this in practice is Wildlife Health Australia’s Sentinel Surveillance Program (Wildlife Health Australia 2023), which integrates data from zoos, veterinary clinics, and universities to monitor wildlife disease cases directly into their co-ordinated national electronic Wildlife Health Information System database.
Finally, with regards to ‘big-picture’ holistic surveillance, shotgun metagenomic sequencing should be integrated. Combining this technique with traditional culture-dependent methods would place researchers in a unique position to gather high-resolution data on host microbial taxa and ARGs present in a wildlife sample, while also enabling investigation of the broader environmental resistome. Shotgun could also offer insights into reservoirs and vectors of AMR, environmental hotspots of HGT, clonal strain evolution and spread, and even co-occurrence of virulence genes for zoonotic disease risk monitoring. However, given the cost of the technique, alongside the volume and complexity of the sequencing data generated, artificial intelligence (AI) technologies will likely be required for efficient analysis and interpretation of results. AI technologies, such as bioinformatic algorithms and machine learning, could identify ARGs and predict resistance trends by integrating broader ecological and environmental factors such as host behaviour and climate change into the datasets supplied. Optimistically, employing AI would decrease the cost and labour involved in data analysis while increasing reliability and comparability of results long-term. This would offer fast, detailed insights that could underpin improved analysis and decision-making with regards to intervention efforts and policy design (Wheeler et al. 2023).
Conclusion
Globally, AMR poses a significant, multi-faceted threat to healthcare for humans and domestic animals. Addressing this complex issue requires design of a holistic OH approach to expand existing surveillance systems to incorporate wildlife. Due to various ecological factors explored in this review, wildlife interact with anthropogenically polluted ecosystems and acquire resistance determinants. However, the dynamics of AMR in wildlife remain understudied, and significant limitations remain, regarding our understanding of the determining ecological factors. Although wildlife are recognized reservoirs and disseminators of AMR in the environment, current AMR surveillance systems still primarily focus on clinical and agricultural settings. Hence, a paradigm shift in surveillance is needed to encompass all OH compartments by integrating wildlife as sentinels of AMR. By detailing and accounting for species-specific ecological factors, we can choose non-invasive, practical, and cost-effective wildlife species to monitor that reside at key OH intersections along the AMR transmission chain. Implementing this strategy will require comparable sampling methodologies that accurately represent the species’ role in environmental AMR dynamics. By integrating wildlife into AMR surveillance and policy, and leveraging continuous advances in high-throughput technologies, we can track and predict pollution and resistance evolution, assess ecological impacts and public health risks, and better understand complex transmission dynamics across ecosystems. In this way, wildlife sentinels can bridge existing gaps in environmental AMR surveillance to create a holistic and comprehensive response to AMR that aligns with the OH paradigm.
Author contributions
Caoimhe Doyle (Conceptualization, Funding acquisition, Writing – original draft, Writing - review & editing), Katie Wall (Writing – original draft, Writing - review & editing), Seamus Fanning (Conceptualization, Supervision, Writing – review & editing), and Barry J. McMahon (Conceptualization, Funding acquisition, Supervision, Writing – review & editing)
Conflict of interest
None declared.
Funding
This work has been supported by Taighde Éireann—Research Ireland (Grant Numbers: GOIPG/2022/506 and GOIPG/2019/2608).
Data availability
No new data were generated or analysed in support of this research. All data mentioned is available from the original source papers cited within this review.