-
PDF
- Split View
-
Views
-
Cite
Cite
Sara Bahrami, Hashem Andishmand, Zahra Pilevar, Fataneh Hashempour-baltork, Mohammadali Torbati, Manouchehr Dadgarnejad, Hossein Rastegar, Seyed Ali Mohammadi, Sodeif Azadmard-Damirchi, Innovative perspectives on bacteriocins: advances in classification, synthesis, mode of action, and food industry applications, Journal of Applied Microbiology, Volume 135, Issue 11, November 2024, lxae274, https://doi.org/10.1093/jambio/lxae274
- Share Icon Share
Abstract
Bacteriocins, natural antimicrobial peptides produced by bacteria, present eco-friendly, non-toxic, and cost-effective alternatives to traditional chemical antimicrobial agents in the food industry. This review provides a comprehensive update on the classification of bacteriocins in food preservation. It highlights the significant industrial potential of pediocin-like and two-peptide bacteriocins, emphasizing chemical synthesis methods like Fmoc-SPPS to meet the demand for bioactive bacteriocins. The review details the mode of action, focusing on mechanisms such as transmembrane potential disruption and pH-dependent effects. Furthermore, it addresses the limitations of bacteriocins in food preservation and explores the potential of nanotechnology-based encapsulation to enhance their antimicrobial efficacy. The benefits of nanoencapsulation, including improved stability, extended antimicrobial spectrum, and enhanced functionality, are underscored. This understanding is crucial for advancing the application of bacteriocins to ensure food safety and quality.
Introduction
In recent years, there has been growing interest in the use of bacteriocins as natural antimicrobial compounds in the food industry (Gaspar et al. 2018, Pilevar et al. 2020a). Bacteriocins are antimicrobial peptides produced by a wide range of bacterial groups. While those produced by lactic acid bacteria (LAB) are the most extensively studied for their applications in food preservation due to their Generally Recognized As Safe (GRAS) status and effective inhibition of foodborne pathogens, many other bacterial groups also produce bacteriocins with significant potential in various applications (Raman et al. 2022). These compounds possess potent antimicrobial activity against various food spoilage and pathogenic microorganisms (Chikindas et al. 2018). Their ability to inhibit the growth of target bacteria while being safe for human consumption has made them attractive alternatives to traditional antimicrobial agents (Hashempour-Baltork et al. 2019, Pilevar et al. 2024a,b). Bacteriocins are antimicrobial peptides that are produced by bacteria and can kill or inhibit different other bacterial strains, and therefore can be used in food preservation, cancer therapy, and pathogen treatment (Yang et al. 2014). Recently, bacterial ribosomally synthesized peptides have raised attention and revolutionized treatments against multidrug-resistant (MDR) pathogens due to their stability, low toxicity, and high antimicrobial activities. Although scientists believe that the activity of bacteriocins is due to their antagonism functions through several signaling molecules, there are many unknowns regarding the ecological function of bacteriocins (Reuben and Torres 2024).
Classification of bacteriocins provides a framework for organizing and categorizing the diverse array of antimicrobial peptides and proteins produced by bacteria. Two-peptide bacteriocins (class IIb) pediocin-like (class IIa) bacteriocins have emerged as important classes of bacteriocins due to their distinctive antimicrobial properties and industrial potential (Radaic et al. 2020). These bacteriocins exhibit unique modes of action; for instance, class IIb tends to form pores with specificity and causes membrane penetrating helix-helix structure, which leads to membrane leakage, and class IIa binds to the membrane-embedded part of the mannose phosphotransferase permease and similar to class IIb bacteriocins lead to membrane leakage and targeting the transmembrane potential of bacterial cells and disrupting their integrity, leading to cell death (Nissen-Meyer et al. 2010, Garcia-Gutierrez et al. 2020).
Advancements in synthesis methods have facilitated the production of bacteriocins through chemical synthesis approaches. Chemical synthesis, particularly using the 9-fluorenylmethoxycarbonyl (Fmoc) solid-phase synthesis (SPPS) method, offers a versatile and efficient means of obtaining bioactive bacteriocins in large quantities (Bédard et al. 2018). Fmoc Solid-Phase Peptide Synthesis (Fmoc-SPPS) is a highly efficient and convenient method for producing small to medium-sized peptides. It involves binding the growing peptide chain to an insoluble polymeric support, which facilitates reaction completion through the use of larger amounts of reagents. The process begins with attaching a Fmoc-protected amino acid to a resin, followed by repeated cycles of deprotection and coupling to elongate the peptide. This method ensures high yield and purity due to the easy separation of the peptide from reagents and solvents at each step. Fmoc-SPPS is widely used in research and pharmaceutical applications for synthesizing peptides for drug development, biochemical studies, and vaccine development, regarding its operational simplicity, flexibility, and ability to produce high-quality peptides efficiently (Bédard and Biron 2018). This capability is of utmost importance for their application in the food industry as potent antimicrobial agents to prevent food spoilage and ensure food safety.
While bacteriocins hold significant promise, their application in the food industry faces several challenges. Factors such as the presence of food additives, the chemical composition of food matrices, physical conditions, and the sensitivity of proteolytic enzymes can influence the efficacy of bacteriocins as food preservatives (Oroojalian et al. 2020). Overcoming these limitations requires innovative approaches. Nanotechnology-based encapsulation has emerged as a promising strategy to enhance the antimicrobial activity and stability of bacteriocins in food systems. Encapsulation techniques using nanoparticles such as liposomes, chitosan, proteins, and polysaccharides have shown potential in improving the delivery and performance of bacteriocins. These nanoencapsulated bacteriocins offer advantages such as increased stability, extended antimicrobial spectrum, and reduced risk of toxic side effects (Pinilla et al. 2021).
This paper aims to provide an update on the new generation of bacteriocins, focusing on their classification, synthesis, mode of action, and application in the food industry. Pediocin-like and two-peptide bacteriocins are highlighted in our review due to their distinctive antimicrobial properties, practical applications, and potential for innovation in the food industry. By highlighting these bacteriocins, we aim to provide a comprehensive understanding of their benefits and encourage further research and development in this area. Additionally, the potential of nanotechnology-based encapsulation strategies to enhance the efficacy of bacteriocins as antimicrobial agents and the application of bacteriocins in the food industry will be explored. Understanding these aspects is essential for harnessing the full potential of bacteriocins and advancing their utilization in the food industry to ensure food safety and quality.
Classification of bacteriocins
Classification
LAB bacteriocins have long been used in the fermentation and storage of meat and dairy products (Pilevar et al. 2022). So far, various classifications for bacteriocins have been proposed. According to several studies, such as Reuben and Torres (2024) and Soltani et al. (2021) classification system, bacteriocins can generally be categorized into two primary classes, including class I and class II based on the presence or absence of post-translationally modified peptides (RiPPs) (Fig. 1). Based on this classification, class II bacteriocins contain 3 subclasses, which include pediocin-like bacteriocins (single peptides containing the YGNGV consensus sequence), two-peptides bacteriocins (containing two or more unmodified peptides), and non-pediocin-like bacteriocins (unmodified linear single peptides devoid of the YGNGV) (Reuben and Torres 2024). However, it should be noted that the most usual classification of bacteriocins is based on their physicochemical and structural characteristics, which are classified into three categories as follows: (Fig. 2).
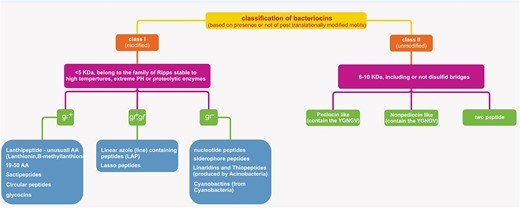
Classification of bacteriocins based on the presence or absence of post-translationally modified peptides (RiPPs).
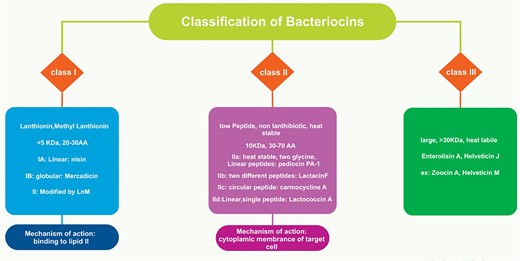
Classification of bacteriocins based on their physicochemical and structural characteristics.
Class I bacteriocins: RiPPs (ribosomally produced and post-translationally modified peptides)
Class I contains all peptides that are enzymatically modified over the biosynthesis course (Alvarez-Sieiro et al. 2016). Class I bacteriocins belong to the group of RiPPs, which are also called ribosomal peptide natural products (RPNPs), and encompass a diverse array of natural products. These peptides are initially synthesized by ribosomes and then undergo enzymatic modifications to attain their final bioactive forms. RiPPs encompass a wide variety of peptides characterized by distinct structures, functions, and biological activities (Li and Rebuffat 2020).
The RiPPs family encompasses a variety of peptide classes beyond bacteriocins, each with unique biosynthetic pathways and post-translational modifications. Examples of RiPPs include lanthipeptides, thiopeptides, lasso peptides, cyanobactins, microcins, etc (Table 1). These peptides exhibit diverse mechanisms of action, ranging from antimicrobial and antiviral activities to enzyme inhibition and receptor modulation (Reuben and Torres 2024).
RiPPs peptides . | Producer strain example . | Characterization . | Specific effect or properties . | Mechanism of action . | References . |
---|---|---|---|---|---|
Lanthipeptides | Streptococcus, and Bacillus spp. | Presence of lanthionine or methyllanthionine residues, formed through the cyclization of serine or threonine residues with cysteine residues. | High stability and potency against a variety of pathogens. Such as nisin, which is widely used in food preservation. | Binding to lipid II, which is a crucial precursor in peptidoglycan synthesis, thereby disrupting the formation of cell walls or bacterial ribosomes. | (Repka et al. 2017, Heeney et al. 2019) (Camargo et al. 2018) |
Lasso peptides | Actinobacteria, Streptomyces leeuwenhoekii and Streptomyces sannurensis | Unique lasso-like structure, where the C-terminal tail is threaded through a macrolactam ring formed by the N-terminus. | High stability and resistance to proteolytic degradation. Lasso peptides often exhibit antimicrobial or antiviral activities. | The bactericidal mechanism of lassomycin stimulates the ATPase activity of ClpC1 but uncouples it from ClpP1P2-dependent proteolysis, the exact mechanism of antiviral activity remains unsolved. | (Stariha and McCafferty 2021) |
Cyanobactins | Cyanobacteria spp. | Diverse post-translational modifications, including heterocyclization and prenylation. | Only a few cyanobactins have been reported to possess antibacterial activity. Interesting examples are the kawaguchipeptins A and B isolated from Microcystis aeruginosa NIES-88. | The N-methylation of the phenylalanine in pitipeptolide A (4) was determined as an essential factor for the compound's antibacterial activity. | (Sivonen et al. 2010, Carpine and Sieber 2021) |
Microcins | Enterobacteria spp. | Are non-SOS-inducible, ribosomally synthesized peptides, in some cases only active after post-translational modification. | Small antimicrobial peptides that often target specific bacterial species, making them effective narrow-spectrum antibiotics. | Forming pores in the bacterial membrane inhibiting aspartyl-tRNA synthetase, essential in protein synthesis, inhibiting the DNA gyrase GyrB, resulting in double DNA breaks. | (Baquero et al. 2019, Marković et al. 2022) |
Sactipeptides | Escherichia spp. | Presence of thioether linkages between a cysteine-sulfur and the α-carbon of another residue. | Inhibition and virucidal effects on HSV-1 and HSV-2 at ≤ 100 µg/ml and 200 µg/ml, respectively. | Uncertain. | (Fu et al. 2021) |
Thiopeptides | Bacillus spp. | Typically feature a 6-membered nitrogenous ring that serves as a scaffold for at least one macrocycle and a tail. | In nanomolar concentrations, it suppresses the activity of Gram-positive pathogens and prevents viral entry. | Interfering with viral glycoprotein E2 (putative) and blocking protein translation of Gram-positive pathogens. | (Schwalen et al. 2018) |
Proteusins | Cyanobacteria spp. | Composed of largely genome-predicted bacterial peptides carrying several uncommon post-translational modifcations. | Inhibitory action against Lymphocytic choriomeningitis infection disease in mouse cells at low concentrations without poisonous quality towards the host cell. | Membrane binding and disruptive action. | (Bösch et al. 2020) |
Cyclotides | Violaceae, Solanaceae, Rubiaceae, Cucurbitaceae, and Fabaceae spp. | Having 30 amino acids in size and are categorized by their head-to-tail cyclic backbone and cystine knot motif. | Remarkably stable, with resistance to enzymatic or thermal degradation. | Disruption of membranes by a pore formation mechanism. | (De Veer et al. 2019) |
RiPPs peptides . | Producer strain example . | Characterization . | Specific effect or properties . | Mechanism of action . | References . |
---|---|---|---|---|---|
Lanthipeptides | Streptococcus, and Bacillus spp. | Presence of lanthionine or methyllanthionine residues, formed through the cyclization of serine or threonine residues with cysteine residues. | High stability and potency against a variety of pathogens. Such as nisin, which is widely used in food preservation. | Binding to lipid II, which is a crucial precursor in peptidoglycan synthesis, thereby disrupting the formation of cell walls or bacterial ribosomes. | (Repka et al. 2017, Heeney et al. 2019) (Camargo et al. 2018) |
Lasso peptides | Actinobacteria, Streptomyces leeuwenhoekii and Streptomyces sannurensis | Unique lasso-like structure, where the C-terminal tail is threaded through a macrolactam ring formed by the N-terminus. | High stability and resistance to proteolytic degradation. Lasso peptides often exhibit antimicrobial or antiviral activities. | The bactericidal mechanism of lassomycin stimulates the ATPase activity of ClpC1 but uncouples it from ClpP1P2-dependent proteolysis, the exact mechanism of antiviral activity remains unsolved. | (Stariha and McCafferty 2021) |
Cyanobactins | Cyanobacteria spp. | Diverse post-translational modifications, including heterocyclization and prenylation. | Only a few cyanobactins have been reported to possess antibacterial activity. Interesting examples are the kawaguchipeptins A and B isolated from Microcystis aeruginosa NIES-88. | The N-methylation of the phenylalanine in pitipeptolide A (4) was determined as an essential factor for the compound's antibacterial activity. | (Sivonen et al. 2010, Carpine and Sieber 2021) |
Microcins | Enterobacteria spp. | Are non-SOS-inducible, ribosomally synthesized peptides, in some cases only active after post-translational modification. | Small antimicrobial peptides that often target specific bacterial species, making them effective narrow-spectrum antibiotics. | Forming pores in the bacterial membrane inhibiting aspartyl-tRNA synthetase, essential in protein synthesis, inhibiting the DNA gyrase GyrB, resulting in double DNA breaks. | (Baquero et al. 2019, Marković et al. 2022) |
Sactipeptides | Escherichia spp. | Presence of thioether linkages between a cysteine-sulfur and the α-carbon of another residue. | Inhibition and virucidal effects on HSV-1 and HSV-2 at ≤ 100 µg/ml and 200 µg/ml, respectively. | Uncertain. | (Fu et al. 2021) |
Thiopeptides | Bacillus spp. | Typically feature a 6-membered nitrogenous ring that serves as a scaffold for at least one macrocycle and a tail. | In nanomolar concentrations, it suppresses the activity of Gram-positive pathogens and prevents viral entry. | Interfering with viral glycoprotein E2 (putative) and blocking protein translation of Gram-positive pathogens. | (Schwalen et al. 2018) |
Proteusins | Cyanobacteria spp. | Composed of largely genome-predicted bacterial peptides carrying several uncommon post-translational modifcations. | Inhibitory action against Lymphocytic choriomeningitis infection disease in mouse cells at low concentrations without poisonous quality towards the host cell. | Membrane binding and disruptive action. | (Bösch et al. 2020) |
Cyclotides | Violaceae, Solanaceae, Rubiaceae, Cucurbitaceae, and Fabaceae spp. | Having 30 amino acids in size and are categorized by their head-to-tail cyclic backbone and cystine knot motif. | Remarkably stable, with resistance to enzymatic or thermal degradation. | Disruption of membranes by a pore formation mechanism. | (De Veer et al. 2019) |
RiPPs peptides . | Producer strain example . | Characterization . | Specific effect or properties . | Mechanism of action . | References . |
---|---|---|---|---|---|
Lanthipeptides | Streptococcus, and Bacillus spp. | Presence of lanthionine or methyllanthionine residues, formed through the cyclization of serine or threonine residues with cysteine residues. | High stability and potency against a variety of pathogens. Such as nisin, which is widely used in food preservation. | Binding to lipid II, which is a crucial precursor in peptidoglycan synthesis, thereby disrupting the formation of cell walls or bacterial ribosomes. | (Repka et al. 2017, Heeney et al. 2019) (Camargo et al. 2018) |
Lasso peptides | Actinobacteria, Streptomyces leeuwenhoekii and Streptomyces sannurensis | Unique lasso-like structure, where the C-terminal tail is threaded through a macrolactam ring formed by the N-terminus. | High stability and resistance to proteolytic degradation. Lasso peptides often exhibit antimicrobial or antiviral activities. | The bactericidal mechanism of lassomycin stimulates the ATPase activity of ClpC1 but uncouples it from ClpP1P2-dependent proteolysis, the exact mechanism of antiviral activity remains unsolved. | (Stariha and McCafferty 2021) |
Cyanobactins | Cyanobacteria spp. | Diverse post-translational modifications, including heterocyclization and prenylation. | Only a few cyanobactins have been reported to possess antibacterial activity. Interesting examples are the kawaguchipeptins A and B isolated from Microcystis aeruginosa NIES-88. | The N-methylation of the phenylalanine in pitipeptolide A (4) was determined as an essential factor for the compound's antibacterial activity. | (Sivonen et al. 2010, Carpine and Sieber 2021) |
Microcins | Enterobacteria spp. | Are non-SOS-inducible, ribosomally synthesized peptides, in some cases only active after post-translational modification. | Small antimicrobial peptides that often target specific bacterial species, making them effective narrow-spectrum antibiotics. | Forming pores in the bacterial membrane inhibiting aspartyl-tRNA synthetase, essential in protein synthesis, inhibiting the DNA gyrase GyrB, resulting in double DNA breaks. | (Baquero et al. 2019, Marković et al. 2022) |
Sactipeptides | Escherichia spp. | Presence of thioether linkages between a cysteine-sulfur and the α-carbon of another residue. | Inhibition and virucidal effects on HSV-1 and HSV-2 at ≤ 100 µg/ml and 200 µg/ml, respectively. | Uncertain. | (Fu et al. 2021) |
Thiopeptides | Bacillus spp. | Typically feature a 6-membered nitrogenous ring that serves as a scaffold for at least one macrocycle and a tail. | In nanomolar concentrations, it suppresses the activity of Gram-positive pathogens and prevents viral entry. | Interfering with viral glycoprotein E2 (putative) and blocking protein translation of Gram-positive pathogens. | (Schwalen et al. 2018) |
Proteusins | Cyanobacteria spp. | Composed of largely genome-predicted bacterial peptides carrying several uncommon post-translational modifcations. | Inhibitory action against Lymphocytic choriomeningitis infection disease in mouse cells at low concentrations without poisonous quality towards the host cell. | Membrane binding and disruptive action. | (Bösch et al. 2020) |
Cyclotides | Violaceae, Solanaceae, Rubiaceae, Cucurbitaceae, and Fabaceae spp. | Having 30 amino acids in size and are categorized by their head-to-tail cyclic backbone and cystine knot motif. | Remarkably stable, with resistance to enzymatic or thermal degradation. | Disruption of membranes by a pore formation mechanism. | (De Veer et al. 2019) |
RiPPs peptides . | Producer strain example . | Characterization . | Specific effect or properties . | Mechanism of action . | References . |
---|---|---|---|---|---|
Lanthipeptides | Streptococcus, and Bacillus spp. | Presence of lanthionine or methyllanthionine residues, formed through the cyclization of serine or threonine residues with cysteine residues. | High stability and potency against a variety of pathogens. Such as nisin, which is widely used in food preservation. | Binding to lipid II, which is a crucial precursor in peptidoglycan synthesis, thereby disrupting the formation of cell walls or bacterial ribosomes. | (Repka et al. 2017, Heeney et al. 2019) (Camargo et al. 2018) |
Lasso peptides | Actinobacteria, Streptomyces leeuwenhoekii and Streptomyces sannurensis | Unique lasso-like structure, where the C-terminal tail is threaded through a macrolactam ring formed by the N-terminus. | High stability and resistance to proteolytic degradation. Lasso peptides often exhibit antimicrobial or antiviral activities. | The bactericidal mechanism of lassomycin stimulates the ATPase activity of ClpC1 but uncouples it from ClpP1P2-dependent proteolysis, the exact mechanism of antiviral activity remains unsolved. | (Stariha and McCafferty 2021) |
Cyanobactins | Cyanobacteria spp. | Diverse post-translational modifications, including heterocyclization and prenylation. | Only a few cyanobactins have been reported to possess antibacterial activity. Interesting examples are the kawaguchipeptins A and B isolated from Microcystis aeruginosa NIES-88. | The N-methylation of the phenylalanine in pitipeptolide A (4) was determined as an essential factor for the compound's antibacterial activity. | (Sivonen et al. 2010, Carpine and Sieber 2021) |
Microcins | Enterobacteria spp. | Are non-SOS-inducible, ribosomally synthesized peptides, in some cases only active after post-translational modification. | Small antimicrobial peptides that often target specific bacterial species, making them effective narrow-spectrum antibiotics. | Forming pores in the bacterial membrane inhibiting aspartyl-tRNA synthetase, essential in protein synthesis, inhibiting the DNA gyrase GyrB, resulting in double DNA breaks. | (Baquero et al. 2019, Marković et al. 2022) |
Sactipeptides | Escherichia spp. | Presence of thioether linkages between a cysteine-sulfur and the α-carbon of another residue. | Inhibition and virucidal effects on HSV-1 and HSV-2 at ≤ 100 µg/ml and 200 µg/ml, respectively. | Uncertain. | (Fu et al. 2021) |
Thiopeptides | Bacillus spp. | Typically feature a 6-membered nitrogenous ring that serves as a scaffold for at least one macrocycle and a tail. | In nanomolar concentrations, it suppresses the activity of Gram-positive pathogens and prevents viral entry. | Interfering with viral glycoprotein E2 (putative) and blocking protein translation of Gram-positive pathogens. | (Schwalen et al. 2018) |
Proteusins | Cyanobacteria spp. | Composed of largely genome-predicted bacterial peptides carrying several uncommon post-translational modifcations. | Inhibitory action against Lymphocytic choriomeningitis infection disease in mouse cells at low concentrations without poisonous quality towards the host cell. | Membrane binding and disruptive action. | (Bösch et al. 2020) |
Cyclotides | Violaceae, Solanaceae, Rubiaceae, Cucurbitaceae, and Fabaceae spp. | Having 30 amino acids in size and are categorized by their head-to-tail cyclic backbone and cystine knot motif. | Remarkably stable, with resistance to enzymatic or thermal degradation. | Disruption of membranes by a pore formation mechanism. | (De Veer et al. 2019) |
Class II bacteriocins: (unmodified bacteriocins)
Class II, or non-lantibiotic bacteriocins, are peptides with 30–70 amino acids and generated through LAB (González-Pérez et al. 2018, Ibrahim 2019). These subclasses consist of type IIa, type IIb, and type IIc and contain very large groups of thermostable, non-lanthionine, and unmodified peptides (<10 kDa) with a helical amphiphilic structure that permit them to enter the target cells. Class IIa is defined by the existence of an N-terminal consensus hydrophilic sequence Tyr-Gly-Asn-Gly-Val-Xaa-Cys and is active against Listeria monocytogenes. Class IIb includes lactacin F and lactococcin G (LcnG), which act synergically with antimicrobial effects. Class IIc form pores in the target cells (Geldart and Kaznessis 2017; S. Hosseini et al. 2021; H. Negash and Tsehai 2020, Pilevar et al. 2020b, Hosseini et al. 2024).
Similar to lantibiotics, bacteriocins belonging to this class are synthesized as inactive pre-peptides that commonly possess a distinctive proteolytic double-glycine processing site (Perez et al. 2014). This category is especially interesting because it would be more easily used in biotechnological and medical uses. Pediocin PA-1 has been used successfully as a biopreservative ingredient in food processing as ALTA® 2341, a commercial fermenting agent (Taylor 2014).
Class III bacteriocins
Class III bacteriocins are thermolabile antimicrobial proteins over 30 kD developed mainly by Enterococcus (E.) and Lactobacillus (Lb.) spp. Colicins, produced by Escherichia coli strains containing colicinogenic plasmids, are an example of class III bacteriocins (Ahmad et al. 2017, Negash and Tsehai 2020). Helveticin M, helveticin J, enterolysin A, and zoocin A, developed by Lb. crispatus, Lb. helveticus, and E. faecalis, are class III bacteriocins (Cui et al. 2012). Enterolysin A is a domain of N-terminal endopeptidase and, similar to zoocin A, a C-terminal substrate recognition domain (Alvarez-Sieiro et al. 2016). These bacteriocins are categorized as thermolabile lytic and non-lytic thermolabile bacteriocins (Ahmad et al. 2017).
Novel classification
According to Simons et al. (2020), classifying bacteriocins has been challenging due to their significant structural and functional diversity. Due to their abundance and diversity, several classification systems have been proposed over time (Lagha et al. 2017). Attempting to categorize all known bacteriocins into distinct groups is nearly impossible because of the vast number of bacteriocins and the overlapping structural, functional, and genetic characteristics they exhibit (Simons et al. 2020). Wang et al. (2016), reported that databases such as APD3 have been developed to catalog antimicrobial peptides. For example, various studies (Zacharof and Lovitt 2012, Perez et al. 2014, Ahmad et al. 2017) have classified bacteriocins into different classes based on factors such as size, molecular composition, structure, and modification processes. According to Simons et al. (2020), the primary differentiation between bacteriocins is based on the type of producing organism: Gram-positive and Gram-negative bacteriocins.
In this paper, the following classification provides a detailed understanding of the diverse structures, mechanisms, and applications of bacteriocins, highlighting their potential in various fields, including food preservation, medicine, and biotechnology, according to Zhang et al. (2022). Table 2 summarized the novel classification and characterization of bacteriocins.
Class . | Type . | Examples . | References . |
---|---|---|---|
Class I: Lantibiotics | Type A (linear lantibiotics) | • Nisin: Widely used as a food preservative due to its ability to inhibit a broad spectrum of Gram-positive bacteria. • Subtilin: Produced by Bacillus subtilis, has similar properties to nisin but is less commonly used. | Jack et al. (1995), Kuwano et al. (2005), Cebrián et al. (2019), Mercado and Olmos (2022) |
Type B (globular lantibiotics) | • Mersacidin: Inhibits peptidoglycan biosynthesis by binding to lipid II • Actagardine: Similar mode of action to mersacidin, used primarily in research settings. | ||
Type C (hybrid lantibiotics) | Specific hybrid lantibiotics active against pathogenic Clostridium spp. | ||
Class IIa | Pediocin-like bacteriocins | Pediocin PA-1: Produced by Pediococcus acidilactici, widely studied for its anti-Listeria properties. —Sakacin: Produced by Lactobacillus sakei, also effective. | Sánchez-Hidalgo et al. (2011) |
Class IIb | Two-Peptide Bacteriocins | LcnG: Produced by Lactococcus lactis, requires two peptides (LcnG-α and LcnG-β) to function. | Sánchez-Hidalgo et al. (2011) |
Class IIc | Circular bacteriocins | —AS-48: Produced by Enterococcus faecalis, has a broad spectrum of activity against Gram-positive bacteria. | Sánchez-Hidalgo et al. (2011) |
Class IId | Single linear bacteriocins | —Lacticin Q: Produced by Lactococcus lactis, has a distinct mode of action compared to other linear bacteriocins. | Acedo et al. (2016) |
Class IIe | Leaderless bacteriocins | —Aureocin A53: Produced by Staphylococcus aureus, does not have a typical leader peptide. | Acedo et al. (2016) |
Class III: Large, Heat-Labile Proteins | Type A (large, heat-labile bacteriocins) | —Helveticin J: Produced by Lb. helveticus, has lytic activity against a variety of Gram-positive bacteria. —Enterolysin A: Produced by E. faecalis, known for its broad-spectrum activity. | Sun et al. (2018) |
Class IV: Complex Bacteriocins | Lipoprotein bacteriocins | Lactococcin DR1: A lipoprotein bacteriocin with enhanced membrane-targeting capabilities. | Sánchez et al. (2000), Bédard and Biron (2018), Paškevičius et al. (2022) |
Glycosylated bacteriocins | Glycosylated variants of known bacteriocins are often engineered for specific applications. | ||
Chimeric bacteriocins | —Engineered chimeric bacteriocins designed for research and therapeutic purposes. | ||
Class V: Unusual Bacteriocins | Tailocins: F-type and R-type | Both of which exclusively target bacterial cells with narrow specificity, resulting in minimal collateral damage to non-target microbiota compared to conventional antibiotics. | Saha (2016) |
Thiopeptides | —Thiocillins: Produced by Bacillus cereus, known for their potent antibacterial activity. | ||
Sactibiotics | —Subtilosin A: Produced by Bacillus subtilis, has unique sulfur linkages contributing to its antimicrobial activity. | ||
Class VI: Post-Translationally Modified Bacteriocins | LanM-type lantibiotics | —Duramycin: Produced by Streptomyces cinnamoneus, has modifications that enhance its antimicrobial properties. | Solis-Balandra and Sanchez-Salas (2024) |
Cyclic bacteriocins | —Circularin A: Produced by Clostridium beijerinckii, is known for its robust cyclic structure. | ||
Emerging Categories | Bacteriocins from non-LAB | Bacillocins: Produced by Bacillus species, with diverse antimicrobial activities. | Chopra et al. (2015), Rashmi (2017) |
Synthetic and Engineered Bacteriocins | —Synthetic versions of nisin or other bacteriocins engineered for improved characteristics. | Musiejuk and Kafarski (2023) | |
Functional and Application-Based Categories | Food preservative bacteriocins | —Nisin: Widely used in the food industry to extend shelf life and ensure safety. —Pediocin: Used in various food products to prevent the growth of Listeria. | Chikindas et al. (2018) |
Therapeutic bacteriocins | Nisin-based formulations: Used for treating skin infections and as part of wound dressings. | ||
Probiotic bacteriocins | Bacteriocins from Lactobacillus strains: Contribute to gut health by inhibiting harmful bacteria. |
Class . | Type . | Examples . | References . |
---|---|---|---|
Class I: Lantibiotics | Type A (linear lantibiotics) | • Nisin: Widely used as a food preservative due to its ability to inhibit a broad spectrum of Gram-positive bacteria. • Subtilin: Produced by Bacillus subtilis, has similar properties to nisin but is less commonly used. | Jack et al. (1995), Kuwano et al. (2005), Cebrián et al. (2019), Mercado and Olmos (2022) |
Type B (globular lantibiotics) | • Mersacidin: Inhibits peptidoglycan biosynthesis by binding to lipid II • Actagardine: Similar mode of action to mersacidin, used primarily in research settings. | ||
Type C (hybrid lantibiotics) | Specific hybrid lantibiotics active against pathogenic Clostridium spp. | ||
Class IIa | Pediocin-like bacteriocins | Pediocin PA-1: Produced by Pediococcus acidilactici, widely studied for its anti-Listeria properties. —Sakacin: Produced by Lactobacillus sakei, also effective. | Sánchez-Hidalgo et al. (2011) |
Class IIb | Two-Peptide Bacteriocins | LcnG: Produced by Lactococcus lactis, requires two peptides (LcnG-α and LcnG-β) to function. | Sánchez-Hidalgo et al. (2011) |
Class IIc | Circular bacteriocins | —AS-48: Produced by Enterococcus faecalis, has a broad spectrum of activity against Gram-positive bacteria. | Sánchez-Hidalgo et al. (2011) |
Class IId | Single linear bacteriocins | —Lacticin Q: Produced by Lactococcus lactis, has a distinct mode of action compared to other linear bacteriocins. | Acedo et al. (2016) |
Class IIe | Leaderless bacteriocins | —Aureocin A53: Produced by Staphylococcus aureus, does not have a typical leader peptide. | Acedo et al. (2016) |
Class III: Large, Heat-Labile Proteins | Type A (large, heat-labile bacteriocins) | —Helveticin J: Produced by Lb. helveticus, has lytic activity against a variety of Gram-positive bacteria. —Enterolysin A: Produced by E. faecalis, known for its broad-spectrum activity. | Sun et al. (2018) |
Class IV: Complex Bacteriocins | Lipoprotein bacteriocins | Lactococcin DR1: A lipoprotein bacteriocin with enhanced membrane-targeting capabilities. | Sánchez et al. (2000), Bédard and Biron (2018), Paškevičius et al. (2022) |
Glycosylated bacteriocins | Glycosylated variants of known bacteriocins are often engineered for specific applications. | ||
Chimeric bacteriocins | —Engineered chimeric bacteriocins designed for research and therapeutic purposes. | ||
Class V: Unusual Bacteriocins | Tailocins: F-type and R-type | Both of which exclusively target bacterial cells with narrow specificity, resulting in minimal collateral damage to non-target microbiota compared to conventional antibiotics. | Saha (2016) |
Thiopeptides | —Thiocillins: Produced by Bacillus cereus, known for their potent antibacterial activity. | ||
Sactibiotics | —Subtilosin A: Produced by Bacillus subtilis, has unique sulfur linkages contributing to its antimicrobial activity. | ||
Class VI: Post-Translationally Modified Bacteriocins | LanM-type lantibiotics | —Duramycin: Produced by Streptomyces cinnamoneus, has modifications that enhance its antimicrobial properties. | Solis-Balandra and Sanchez-Salas (2024) |
Cyclic bacteriocins | —Circularin A: Produced by Clostridium beijerinckii, is known for its robust cyclic structure. | ||
Emerging Categories | Bacteriocins from non-LAB | Bacillocins: Produced by Bacillus species, with diverse antimicrobial activities. | Chopra et al. (2015), Rashmi (2017) |
Synthetic and Engineered Bacteriocins | —Synthetic versions of nisin or other bacteriocins engineered for improved characteristics. | Musiejuk and Kafarski (2023) | |
Functional and Application-Based Categories | Food preservative bacteriocins | —Nisin: Widely used in the food industry to extend shelf life and ensure safety. —Pediocin: Used in various food products to prevent the growth of Listeria. | Chikindas et al. (2018) |
Therapeutic bacteriocins | Nisin-based formulations: Used for treating skin infections and as part of wound dressings. | ||
Probiotic bacteriocins | Bacteriocins from Lactobacillus strains: Contribute to gut health by inhibiting harmful bacteria. |
Class . | Type . | Examples . | References . |
---|---|---|---|
Class I: Lantibiotics | Type A (linear lantibiotics) | • Nisin: Widely used as a food preservative due to its ability to inhibit a broad spectrum of Gram-positive bacteria. • Subtilin: Produced by Bacillus subtilis, has similar properties to nisin but is less commonly used. | Jack et al. (1995), Kuwano et al. (2005), Cebrián et al. (2019), Mercado and Olmos (2022) |
Type B (globular lantibiotics) | • Mersacidin: Inhibits peptidoglycan biosynthesis by binding to lipid II • Actagardine: Similar mode of action to mersacidin, used primarily in research settings. | ||
Type C (hybrid lantibiotics) | Specific hybrid lantibiotics active against pathogenic Clostridium spp. | ||
Class IIa | Pediocin-like bacteriocins | Pediocin PA-1: Produced by Pediococcus acidilactici, widely studied for its anti-Listeria properties. —Sakacin: Produced by Lactobacillus sakei, also effective. | Sánchez-Hidalgo et al. (2011) |
Class IIb | Two-Peptide Bacteriocins | LcnG: Produced by Lactococcus lactis, requires two peptides (LcnG-α and LcnG-β) to function. | Sánchez-Hidalgo et al. (2011) |
Class IIc | Circular bacteriocins | —AS-48: Produced by Enterococcus faecalis, has a broad spectrum of activity against Gram-positive bacteria. | Sánchez-Hidalgo et al. (2011) |
Class IId | Single linear bacteriocins | —Lacticin Q: Produced by Lactococcus lactis, has a distinct mode of action compared to other linear bacteriocins. | Acedo et al. (2016) |
Class IIe | Leaderless bacteriocins | —Aureocin A53: Produced by Staphylococcus aureus, does not have a typical leader peptide. | Acedo et al. (2016) |
Class III: Large, Heat-Labile Proteins | Type A (large, heat-labile bacteriocins) | —Helveticin J: Produced by Lb. helveticus, has lytic activity against a variety of Gram-positive bacteria. —Enterolysin A: Produced by E. faecalis, known for its broad-spectrum activity. | Sun et al. (2018) |
Class IV: Complex Bacteriocins | Lipoprotein bacteriocins | Lactococcin DR1: A lipoprotein bacteriocin with enhanced membrane-targeting capabilities. | Sánchez et al. (2000), Bédard and Biron (2018), Paškevičius et al. (2022) |
Glycosylated bacteriocins | Glycosylated variants of known bacteriocins are often engineered for specific applications. | ||
Chimeric bacteriocins | —Engineered chimeric bacteriocins designed for research and therapeutic purposes. | ||
Class V: Unusual Bacteriocins | Tailocins: F-type and R-type | Both of which exclusively target bacterial cells with narrow specificity, resulting in minimal collateral damage to non-target microbiota compared to conventional antibiotics. | Saha (2016) |
Thiopeptides | —Thiocillins: Produced by Bacillus cereus, known for their potent antibacterial activity. | ||
Sactibiotics | —Subtilosin A: Produced by Bacillus subtilis, has unique sulfur linkages contributing to its antimicrobial activity. | ||
Class VI: Post-Translationally Modified Bacteriocins | LanM-type lantibiotics | —Duramycin: Produced by Streptomyces cinnamoneus, has modifications that enhance its antimicrobial properties. | Solis-Balandra and Sanchez-Salas (2024) |
Cyclic bacteriocins | —Circularin A: Produced by Clostridium beijerinckii, is known for its robust cyclic structure. | ||
Emerging Categories | Bacteriocins from non-LAB | Bacillocins: Produced by Bacillus species, with diverse antimicrobial activities. | Chopra et al. (2015), Rashmi (2017) |
Synthetic and Engineered Bacteriocins | —Synthetic versions of nisin or other bacteriocins engineered for improved characteristics. | Musiejuk and Kafarski (2023) | |
Functional and Application-Based Categories | Food preservative bacteriocins | —Nisin: Widely used in the food industry to extend shelf life and ensure safety. —Pediocin: Used in various food products to prevent the growth of Listeria. | Chikindas et al. (2018) |
Therapeutic bacteriocins | Nisin-based formulations: Used for treating skin infections and as part of wound dressings. | ||
Probiotic bacteriocins | Bacteriocins from Lactobacillus strains: Contribute to gut health by inhibiting harmful bacteria. |
Class . | Type . | Examples . | References . |
---|---|---|---|
Class I: Lantibiotics | Type A (linear lantibiotics) | • Nisin: Widely used as a food preservative due to its ability to inhibit a broad spectrum of Gram-positive bacteria. • Subtilin: Produced by Bacillus subtilis, has similar properties to nisin but is less commonly used. | Jack et al. (1995), Kuwano et al. (2005), Cebrián et al. (2019), Mercado and Olmos (2022) |
Type B (globular lantibiotics) | • Mersacidin: Inhibits peptidoglycan biosynthesis by binding to lipid II • Actagardine: Similar mode of action to mersacidin, used primarily in research settings. | ||
Type C (hybrid lantibiotics) | Specific hybrid lantibiotics active against pathogenic Clostridium spp. | ||
Class IIa | Pediocin-like bacteriocins | Pediocin PA-1: Produced by Pediococcus acidilactici, widely studied for its anti-Listeria properties. —Sakacin: Produced by Lactobacillus sakei, also effective. | Sánchez-Hidalgo et al. (2011) |
Class IIb | Two-Peptide Bacteriocins | LcnG: Produced by Lactococcus lactis, requires two peptides (LcnG-α and LcnG-β) to function. | Sánchez-Hidalgo et al. (2011) |
Class IIc | Circular bacteriocins | —AS-48: Produced by Enterococcus faecalis, has a broad spectrum of activity against Gram-positive bacteria. | Sánchez-Hidalgo et al. (2011) |
Class IId | Single linear bacteriocins | —Lacticin Q: Produced by Lactococcus lactis, has a distinct mode of action compared to other linear bacteriocins. | Acedo et al. (2016) |
Class IIe | Leaderless bacteriocins | —Aureocin A53: Produced by Staphylococcus aureus, does not have a typical leader peptide. | Acedo et al. (2016) |
Class III: Large, Heat-Labile Proteins | Type A (large, heat-labile bacteriocins) | —Helveticin J: Produced by Lb. helveticus, has lytic activity against a variety of Gram-positive bacteria. —Enterolysin A: Produced by E. faecalis, known for its broad-spectrum activity. | Sun et al. (2018) |
Class IV: Complex Bacteriocins | Lipoprotein bacteriocins | Lactococcin DR1: A lipoprotein bacteriocin with enhanced membrane-targeting capabilities. | Sánchez et al. (2000), Bédard and Biron (2018), Paškevičius et al. (2022) |
Glycosylated bacteriocins | Glycosylated variants of known bacteriocins are often engineered for specific applications. | ||
Chimeric bacteriocins | —Engineered chimeric bacteriocins designed for research and therapeutic purposes. | ||
Class V: Unusual Bacteriocins | Tailocins: F-type and R-type | Both of which exclusively target bacterial cells with narrow specificity, resulting in minimal collateral damage to non-target microbiota compared to conventional antibiotics. | Saha (2016) |
Thiopeptides | —Thiocillins: Produced by Bacillus cereus, known for their potent antibacterial activity. | ||
Sactibiotics | —Subtilosin A: Produced by Bacillus subtilis, has unique sulfur linkages contributing to its antimicrobial activity. | ||
Class VI: Post-Translationally Modified Bacteriocins | LanM-type lantibiotics | —Duramycin: Produced by Streptomyces cinnamoneus, has modifications that enhance its antimicrobial properties. | Solis-Balandra and Sanchez-Salas (2024) |
Cyclic bacteriocins | —Circularin A: Produced by Clostridium beijerinckii, is known for its robust cyclic structure. | ||
Emerging Categories | Bacteriocins from non-LAB | Bacillocins: Produced by Bacillus species, with diverse antimicrobial activities. | Chopra et al. (2015), Rashmi (2017) |
Synthetic and Engineered Bacteriocins | —Synthetic versions of nisin or other bacteriocins engineered for improved characteristics. | Musiejuk and Kafarski (2023) | |
Functional and Application-Based Categories | Food preservative bacteriocins | —Nisin: Widely used in the food industry to extend shelf life and ensure safety. —Pediocin: Used in various food products to prevent the growth of Listeria. | Chikindas et al. (2018) |
Therapeutic bacteriocins | Nisin-based formulations: Used for treating skin infections and as part of wound dressings. | ||
Probiotic bacteriocins | Bacteriocins from Lactobacillus strains: Contribute to gut health by inhibiting harmful bacteria. |
Class I: lantibiotics
Lantibiotics, as mentioned in conventional classification (Table 1), are a type of bacteriocin characterized by their post-translational modifications, including the formation of thioether bonds. These modifications involve the introduction of unusual amino acids such as lanthionine and methyllanthionine (Pilevar and Hosseini 2017).
Class II: small, heat-stable peptides
Class II bacteriocins are small peptides that are generally resistant to heat and proteolysis, making them robust antimicrobials under various conditions. Class IIa (pediocin-like bacteriocins) are known for their potent activity against Listeria species. They typically act by forming pores in the target cell membrane. This group of bacteriocins includes: pediocin, enterocin A, pediocin PA-1/Ach, carnobacteriocin B2, leucocin A, curvacin A, sakacin P, and enterocin HF (Arbulu et al. 2015). Class IIa bacteriocins have a preserved sequence in their N-terminus, which acts as an essential part of their anti-listeriosis activity (Camargo et al. 2018). A major feature of class IIa bacteriocins is that they contain 37 to 48 amino acid residues and cationic peptides containing a C-terminus having one or two α helix and one or two cysteine bridges (disulfide bonds) with a pleated-sheet N-terminal part. Nearly 50 variants of class IIa bacteriocins have been isolated from various sources, including fermented beef, fermented vegetables, milk products, smoked salmon, the gastrointestinal tract of humans and animals, as well as from topical applications on a range of plants (Perez et al. 2014).
Pediocin-like bacteriocins are found in bacteria like Bacillus coagulans, Bifidobacterium infantis, Bifidobacterium bifidum, and L. innocua. Bacteriocins of this class do not undergo post-translational modifications, except for the formation of disulfide bonds. Similar to other antimicrobial peptides, pediocin-like bacteriocins lack a defined 3D structure when dissolved in water. However, they adopt a specific and stable conformation when exposed to a hydrophobic or interfacial environment (Balandin et al. 2019).
Class IIb (two-peptide bacteriocins)
These bacteriocins require two different peptides to function synergistically, often providing a broader spectrum of activity or enhanced potency. Class IIb bacteriocins are characterized by their heterodimeric nature, which is crucial for their function. These bacteriocins require the presence of two distinct molecules, typically in nearly identical concentrations, to exhibit their maximum antimicrobial activity (Colombo et al. 2018). Remarkably, these bacteriocins consist of two distinct peptides with double glycine-type N-terminal leaders (where glycine may be substituted with alanine or serine). The presence of these two peptides in approximately equal amounts is essential for optimal antimicrobial activity (Britton et al. 2020).
In aqueous environments, these two-peptide bacteriocins adopt a random coil conformation, while in membrane-mimicking conditions, they assume an α-helical state. Two-peptide bacteriocins can be further classified into E-type and S-type. The E-type exhibits enhanced functionality when combined with other polypeptides, resulting in improved bacteriostatic activity. Each polypeptide within these bacteriocins possesses its own distinct activity (Nissen-Meyer et al. 2011, Acedo et al. 2018).
To date, at least 15 two-peptide bacteriocins have been isolated. The first, LcnG from L. lactis, was isolated in 1992 (Pérez-Ramos et al. 2021). A novel two-peptide bacteriocin isolated from Lactococcus lactis QU 4, known as Lactococcin Q, contains two peptides: Qa and Qb. Both lactococcins Q and G have a narrow and specific antimicrobial spectrum against strains isolated from Lactococcus lactis (Simons et al. 2020). Enterocin X, a new two-peptide bacteriocin from E. faecium KU-B5, consists of two peptides: Xa and Xb. In contrast, enterocin NKR-5–3AZ (Ent53AZ) is one of the various bacteriocins produced by the bacterial strain E. faecium NKR-5–3. Their mode of action includes potential membrane disruption and reduced intracellular ATP concentration (Fig. 2) (Balciunas 2013). The discovery of new two-peptide bacteriocins with novel synergistic mechanisms has expanded our understanding of this subclass. For example, new variants have been found that interact with different membrane components, leading to more effective pore formation and bacterial cell death.
Class IIc (circular bacteriocins)
These bacteriocins form a cyclic structure through head-to-tail peptide bonds, which provides enhanced stability and resistance to proteolytic degradation (Sánchez-Hidalgo et al. 2011). According to Sánchez-Hidalgo et al. (2011), AS-48 serves as the model for circular bacteriocins (class IV), with its structure and genetic regulation fully understood. AS-48 is a 70-residue, gene-encoded, α-helical circular cationic bacteriocin produced by various Enterococcus species. Its antimicrobial properties have been well documented against food-borne Gram-positive and Gram-negative pathogens (such as E. coli, Salmonella spp., Listeria monocytogenes, Staphylococcus aureus, and Bacillus cereus) as well as food-spoilage bacteria (including Bacillus spp. and Paenibacillus spp.). The stability and solubility of AS-48 across a broad range of pH levels and temperatures make it a promising candidate for use as a food biopreservative (Sánchez-Hidalgo et al. 2011).
Class IId (single linear bacteriocins)
These are linear peptides that do not fit into the other Class II subcategories due to their unique structures and mechanisms of action (Acedo et al. 2016). Class IId is a miscellaneous group of unmodified, linear, non-pediocin-like bacteriocins with different structures, mechanisms of secretion, and antimicrobial modes of action (Ovchinnikov et al. 2016). These bacteriocins miss the sequence motif characteristic of pediocins (Oftedal et al. 2021).
Class IIe (leaderless bacteriocins)
These bacteriocins lack a leader sequence that is typically involved in guiding the bacteriocin through the secretion pathway. They are secreted via non-classical pathways (Acedo et al. 2016). According to Acedo et al. lacticin and aureocin are notable for lacking an N-terminal leader sequence and exhibit activity against a wide spectrum of Gram-positive bacteria. Lacticin is composed of 53 amino acids, while Aureocin consists of 51 amino acids, with their sequences being 47% identical (Acedo et al. 2016).
Class III: large, heat-labile proteins
Class III bacteriocins are large proteins that are sensitive to heat and often require specific conditions for activity. Type A (large, heat-labile bacteriocins) proteins are susceptible to heat and proteolytic enzymes. They often exhibit complex modes of action, such as degrading cell walls or membranes of target bacteria (Sun et al. 2018).
Class IV: complex bacteriocins
Class IV bacteriocins have complex structures that include additional non-protein components, such as lipids or carbohydrates (Ranaei et al. 2020). Lipoprotein bacteriocins contain lipid moieties, which can enhance their interaction with bacterial membranes and contribute to their antimicrobial activity (Sánchez et al. 2000). Glycosylated bacteriocins have sugar moieties attached, which can affect their stability, activity, and interaction with target bacteria (Bédard and Biron 2018). Chimeric bacteriocins are fusion proteins that combine domains from different bacteriocins, potentially broadening their spectrum of activity and enhancing their functional properties (Paškevičius et al. 2022).
Class V: unusual bacteriocins
Class V includes bacteriocins with unique structures or modes of action that significantly differ from traditional categories. Tailocins, which resemble bacteriophage tails, can inject DNA or protein toxins into target cells, effectively killing them. Saha (2016), explained that tailocins are particles evolutionarily related to bacteriophage (phage) tails, the viruses that infect bacteria. There are two types of tailocins: F-type and R-type, both of which exclusively target bacterial cells with narrow specificity, resulting in minimal collateral damage to non-target microbiota compared to conventional antibiotics. Additionally, tailocins can be genetically engineered to target previously resistant bacterial strains. Thus, these naturally occurring tailocins offer a promising avenue for developing new therapeutics to address antibiotic resistance (Saha 2016). Sactibiotics contain sulfur-to-alpha carbon linkages, which are rare in nature and confer stability and specificity. Thiopeptides are sulfur-rich macrocyclic peptides with complex structures and potent activity against Gram-positive bacteria.
Class VI: post-translationally modified bacteriocins
These bacteriocins undergo significant post-translational modifications, resulting in unique and potent antimicrobial properties. LanM-Type Lantibiotics, modified by LanM enzymes, contain unusual amino acid residues introduced post-translationally. Cyclic bacteriocins are modified to form cyclic structures via head-to-tail cyclization, which enhances their stability and activity.
Recent studies have identified new pediocin-like bacteriocins with unique structural features and enhanced antimicrobial activity. These updates include modifications to the leader peptides and mature peptide regions that improve stability and functionality. Researchers have obtained varying results in studies investigating the concentration, in vitro aflatoxin binding, and degradation effects of LAB (Fakhri et al. 2021, Mollayusefian et al. 2021, Fakhri et al. 2022, Fakhri et al. 2024, Mahmudiono et al. 2024). In a study by Sezer et al. (2013), the effectiveness of LAB and their bacteriocins in detoxifying aflatoxins was examined. The study evaluated the aflatoxin B1 detoxification abilities of LAB in liquid culture, as concentrated pellets, as well as their bacteriocins and mixtures of these three forms. While bacteria and their bacteriocins were individually effective at detoxification, their efficacy increased when used together. When Lc. lactis and Lb. plantarum were incubated in separate tubes and then mixed, the group showed a significantly increased toxin-binding ability (59%) compared to their use alone. The highest detoxification rate (81%) was achieved when these two strains were incubated together in a single broth culture. Within this co-culture group, bacteriocins alone were the most effective, removing 90% of aflatoxin B1 from the solution (Sezer et al. 2013).
Synthesis of bacteriocins
Synthesis of pediocin-like bacteriocins
Class IIa bacteriocins are regulated by the quorum sensing (QS) system, which is present in both Gram-positive and Gram-negative bacteria. QS is a common bacterial mechanism that monitors the cellular density of a bacterial population (Cui et al. 2012). The regulatory systems involved in controlling the production of class IIa bacteriocins utilize quorum detection mechanisms. These systems consist of three gene products, forming three-component regulatory systems. These components include a membrane-associated histidine protein kinase, an inducer peptide, and a cytoplasmic response regulator (Kjos et al. 2014). Recent findings have revealed that the production of divercin V41 from Carnobacterium divergens V41 relies on a two-component signaling transduction system. Moreover, the synthesis of carnobacteriocins has been demonstrated to involve these two systems in an intriguing manner (Acedo et al. 2017, Marutani-Hert et al. 2020).
Induction peptide A is synthesized at low levels as a biologically inactive precursor with a leader sequence or N-terminal extension. This precursor contains a distinct proteolytic processing site with two glycine residues. Similarly, the leader N-sequence or N-terminal sequence of induction peptide A is synthesized ribosomally at low levels with a double glycine motif (Todorov et al. 2016). The inducer peptides are small, hydrophobic, cationic, heat-stable, and similar to the corresponding class IIa bacteriocins (Tresnak and Hackel 2020). The following cleavage of the precursor at a special processing site simultaneously eliminates the main sequence from the antimicrobial molecules (Geldart and Kaznessis 2017). In the case of pediocin, the leading peptide of 18–24 residues is eliminated after attaining its glycine doublet motif and secreted by the human ATP-binding cassette (ABC) transporter and the accessory proteins (Tresnak and Hackel 2020). The amino acid sequences of several N-terminal leader peptides from class IIa bacteriocins, ranging from 18 to 27 residues in length, have been identified (Tresnak and Hackel 2020).
With the exception of enterosin P, the membrane translocation of pediocin-like bacteriocins is facilitated by accessory proteins and an ABC transporter (Porto et al. 2017). These bacteriocins require sufficient concentrations of these proteins outside the cell. The inducer peptide interacts with a transmembrane histidine kinase, leading to the autophosphorylation of a histidine residue on the cytosolic side of the transmembrane protein (Porto et al. 2017). Subsequently, the phosphorylated histidine protein kinase transfers a phosphate group to its associated response regulator, which acts as a transcription activator. This activator then binds to gene-specific bacteriocin promoters and stimulates transcription (Kareb and Aïder 2020).
The synthesis of class IIa bacteriocins requires a minimum of four genes: a structural bacteriocin gene responsible for encoding a precursor peptide, an immunity gene that encodes an immune protein, genes encoding an ATP-binding cassette transporter, and an accessory protein involved in the extracellular translocation of the bacteriocin (Geldart and Kaznessis 2017). Once secreted, bacteriocins rapidly increase antimicrobial synthesis, significantly impacting the microbiota. Studies have shown that environmental signals such as pH, ionic strength, and growth temperature can influence the regulation of class II bacteriocin production (Chhetri et al. 2019).
The highest production of bacteriocins typically occurs at temperatures above 20°C, but production drops to zero at temperatures above 35°C (Bosma et al. 2017). Studies have shown that the production of sakacin A, a bacteriocin, is temperature-sensitive and regulated by QS. When the temperature exceeds 35°C, cells of Lb. curvatus LTH1174 and Lb. sakei LB706 exhibit reduced synthesis of the inducer factor and bacteriocin (Bosma et al. 2017). Interestingly, if exogenous pheromones are added, the cells restore the production of bacteriocin. Interestingly, the addition of exogenous pheromones restores bacteriocin production, indicating that higher temperature conditions affect inducer factor expression (Jiang et al. 2021).
Synthesis of two-peptide bacteriocins
The synthesis of certain two-peptide bacteriocins is controlled by an intricate system comprising three components: a peptide pheromone, a membrane-associated histidine kinase protein, and response regulators (Ekblad et al. 2017). Class IIb bacteriocins require a minimum of five genes typically found in one or two operons. These genes include two structural genes and genes encoding a specialized ABC carrier for membrane translocation of the bacteriocin, along with an accessory protein possibly involved in bacteriocin secretion (Acedo et al. 2018).
In many cases, additional genes encoding a three-component regulatory system are located on or near the operon containing the structural genes. The genes for the accessory protein and the ABC transporter are usually found either in the same operon as the structural and immunity genes (e.g. LcnG) or in a separate operon (e.g. enterocin 1071, plantaricin E/F, and J/K) (Heeney et al. 2019).
Lactobacillus plantarum C11 produces a peptide pheromone known as plantaricin A, which gives rise to two two-peptide bacteriocins: plantaricin J/K and plantaricin E/F (Nilsen et al. 2020). The gene encoding plantaricin A is located within a regulatory operon containing genes for a histidine kinase protein and two response regulators. Upon release, the peptide pheromones interact with the membrane-bound histidine kinase, activating it and leading to the phosphorylation of the intracellular response regulator. This activation prompts the response regulators to activate the operon responsible for bacteriocin production and secretion (Meng et al. 2021). Initially, plantaricin A interacts non-chirally with membrane lipids, forming an α-helical structure within a specific region of the peptide (Syaputri and Iwahashi 2020). Subsequently, the pheromone adopts a suitable structure and position within the membrane, allowing it to engage in a chiral interaction with the histidine protein kinase located near the membrane-water interface (Syaputri and Iwahashi 2020).
Chemical synthesis of bacteriocins
Despite the high potential and efficacy of bacteriocins, their synthesis is limited primarily due to high manufacturing costs, low production efficiency, demanding technical requirements, and complex purification processes (Bédard and Biron 2018). Chemical synthesis methods have been proposed to address these challenges, aiming for high-yield production. Among these methods, the step-by-step approach and the condensing segment approach are particularly relevant. Recently, large-scale production of bacteriocins through a chemical synthesis approach enhanced the medicine yield for human diseases as well as agri-food systems (Reuben and Torres 2024).
Chemical synthesis of bacteriocins is usually carried out by solid-phase, peptide synthesis in the stepwise assembly (SPPS) (Böhm et al. 2012, Bédard and Biron 2018). The first stage of protein synthesis is necessarily the binding of individual amino acids. Since 1963, SPPS has been the major method used for the routine processing of peptides (Mäde et al. 2014). Chemical methods are now an enhanced option than biotechnology methods for synthesizing medium-sized peptides with recombinant DNA or biocatalysis. The benefits of large-scale SPPS have emerged as a feasible way to produce short and medium peptides from around 5 to 50 residues (Bédard and Biron 2018). Also, it uses a repeated process that causes the polymer-bound peptide chain to be elongated by successive amino acid additions until the peptide has been synthesized for the desired sequence and length.
Two protective groups, tert-butoxycarbonyl (tBu) and 9-fluorenylmethoxycarbonyl (Fmoc) are common chemical synthesis bacteriocins preparation SPPS strategies. The first amino acid is attached to the polymer substrate (resin) using a bonding group, and the strategy used must be considered when selecting the suitable type of resin bond (Góngora-Benítez et al. 2013). To achieve a rapid and efficient separation of the growing peptide product from complex reaction mixtures during synthesis, as well as to protect the C-terminal carboxyl group, the utilization of an insoluble solid resin is recommended. This approach ensures a clean separation while maintaining the integrity of the C-terminal carboxyl group (Guan et al. 2014).
Chemical synthesis of bacteriocins involves a wide range of solid supports, linkers, linking reagents, and binding solvents, with the Fmoc-SPPS method being the most commonly used technique. Pediocin PA-1 is synthesized using solid-phase peptide synthesis (SPPS) with the Fmoc/tBu strategy, utilizing HMPB-ChemMatrix resin. Chemically synthesized PA-1 has demonstrated high antimicrobial potency against Clostridium perfringens. The total yield of PA-1 after chemical synthesis was 11% (Bédard et al. 2018). To prevent difficult bacteriocins purification after microbial fermentation, chemical synthesis is a successful beginning point for achieving pure bacteriocins.
Optimization of bacteriocin production
Optimizing the synthesis methods of bacteriocins is essential to meet the increasing demand for these peptides in various applications, such as food preservation, medicine, and agriculture. Traditional fermentation methods often yield insufficient quantities of bacteriocins and involve complex and costly purification processes. Therefore, alternative methods like the SPPS are gaining attention. Fmoc-SPPS allows for the precise and efficient production of high-purity bacteriocins by assembling peptides stepwise on a solid resin. However, this method requires optimization of parameters such as solid supports, linkers, and binding solvents to enhance efficiency and scalability. Additionally, optimizing synthesis methods is crucial to address issues related to the stability and bioactivity of bacteriocins in various conditions. Factors like temperature and pH during production significantly affect yield and functionality. By refining chemical synthesis processes, it is possible to avoid common challenges of microbial fermentation, such as contamination and product inconsistency. Thus, improving these methods is vital for producing bacteriocins in quantities and qualities suitable for industrial and medical applications, ensuring their practical utility and effectiveness. The growth phase of microorganisms, the richness of the media regarding carbon and nitrogen sources, and the existence of Tween 80 are important factors that influence bacteriocin production (Houra et al. 2020).
Mechanism of action
Acting mechanism of pediocin-like bacteriocins
A lot of bacteriocins, mainly class II, have cationic properties. Therefore, it is believed that interaction and disturbance of the bacterial cytoplasmic membrane are crucial in the process of destruction (Soltani et al. 2021). At first, the binding of positively charged class IIa bacteriocins to the membrane was assumed to not require any specific receptor and only an electrostatic attraction (Balandin et al. 2019).
Before the identification of the mannose-specific phosphoenolpyruvate-dependent phosphotransferase system (man-PTS) as the specific receptor for class IIa bacteriocins, it was believed that the bacteriocins could interact directly with the cell membrane (Trivedi and Nair 2020). Peptides found in pediocin-like bacteriocins, like all other bacteriocins, possess a pI (isoelectric point) ranging from 8 to 11 and carry a positive charge at physiological pH levels (de Freire Bastos et al. 2020).
Class IIa bacteriocins specifically target the sugar transporter proteins of the Man-PTS (Kjos et al. 2011). In Gram-positive Firmicutes and Gram-negative Gamma proteobacteria, except eukaryotes, the PTS is responsible for the transport and phosphorylation of sugars (Jeckelmann and Erni 2020). The Man-PTS family is classified into three groups, namely I, II, and III, with only group I members acting as receptors (Maurya et al. 2021). The Man-PTS consists of several components, including the general PTS protein enzyme I, HPr (a histidine-containing phosphocarrier protein), and the carbohydrate-specific protein complex known as enzyme II. The mannose-specific PTS permeases consist of subunits IIA, IIB, IIC, and IID. The cytoplasmic domains IIA and IIB are involved in phosphorylation, while IIC and IID form a transmembrane protein complex responsible for transport (Jeckelmann and Erni 2020) (Fig. 3).
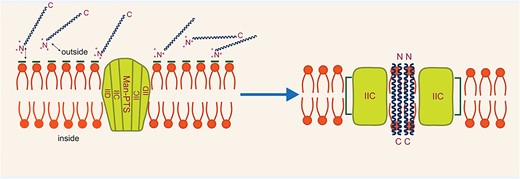
The mechanism of action of class IIa bacteriocins involves binding the extracellular loop of the IIC protein to the N-terminal domain of the bacteriocin's β-sheet region, specifically targeting the extracellular loop of IIC in the Man-PTS (Kjos et al. 2011). Subsequently, the hairpin domain of the bacteriocin interacts with the transmembrane helices of the Man-PTS, inducing conformational changes in the Man-PTS proteins that disrupt transport. This leads to irreversible and uncontrolled alterations in the cell’s essential molecules, ultimately resulting in cell death (Kjos et al. 2011).
Like other class II bacteriocins, pediocin causes a partial or general imbalance in the distribution of transmembrane protons in susceptible cells (Porto et al. 2017). Subsequently, it was suggested that the man-PTS was accountable for the susceptibility of Enterococcus and L. monocytogenes to class IIa bacteriocins (Colombo et al. 2018, Pilevar et al. 2020b). Literature has demonstrated that the flexible hinge found in the conserved N-terminal domain, as well as the highly diverse C-terminal domain, play crucial roles in facilitating the distinct functions of each region of the bacteriocin (Trivedi and Nair 2020). When the N-terminal region binds to the membrane surface, the hydrophobic segment of the lipid bilayer is enveloped by the C-terminal portion.
Acting mechanism of two-peptide bacteriocins
The mechanism of action of class IIb bacteriocins involves interaction with the target membrane, leading to permeabilization. Class IIb bacteriocins appear to make particular pores that dissipate transmembrane potential. Optimal LcnG activity is obtained when both peptides (a and b peptides) are present at a ratio of 1:1. LcnG can permeate the membranes of the target cell for cations, like choline, Cs, Rb, Na, K, and Li, but not for H+ or Mg2+ phosphate (Thongkhao et al. 2018).
When sensitive cells are exposed to bacteriocins, it results in the disruption of the transmembrane potential, leading to a swift decline in cellular ATP levels. Additionally, the release of intracellular potassium ions occurs, which can be detected through pH-dependent 86Rb1 efflux (observed at pH levels higher than 5) (Gan et al. 2021). Plantaricin E/F and plantaricin J/K, unlike LcnG, could make the membranes permeable for individual ions, such as H+, but not with divalent ions like phosphate and Mg2+ (Heeney and Marco 2019).
According to the result of Huang et al. (2021), who conducted comparative genomic analysis and functional characteristics of Lb. acidophilus, the two bacteriocins disintegrate the transmembrane electric potential, leading to the dissipation of the pH gradient and the secretion of small cations (Rb + and choline ions). They concluded that both peptides together exhibited greater effectiveness in enhancing bacterial membrane permeability compared to when each peptide was used separately (Huang et al. 2021). In addition, the high potency of two-peptide bacteriocins shows that membrane permeability depends on a relatively small number of peptides, unlike detergents, which are estimated to impair membrane permeability (Fig. 3).
Bacteriocins in nanotechnology
Nanotechnology appears to be an option for delivering natural preservatives into foods, typically having benefits over the use of free antimicrobials (Lopes and Brandelli 2018, Taghizadeh et al. 2023). The convergence of nanotechnology and biotechnology can be a solution to the problems associated with many biological products and potential viewpoints. The use of bacteriocins as biopreservative/antibacterial agents is contested by a variety of limitations, which involve degradation by proteolytic enzymes (Oroojalian et al. 2020). Also, high doses of bacteriocins are required for the inhibition of resistant multi-drug bacteria that are found in food and water as well as for their adverse reaction with other food constituents (Sidhu and Nehra 2019, Taghizadeh et al. 2019, Sulthana and Archer 2021).
Nano-formulations offer a promising approach to address the limitations associated with bioactive compounds (Andishmand et al. 2024). To protect bacteriocins from enzymatic degradation and prevent unwanted interactions with other food components, nanoencapsulation is an effective technique that improves their stability over prolonged periods (Pinilla et al. 2021). Additionally, by formulating nanomaterials in a targeted manner, they can deliver the active payload to specific tissues or contaminated areas. This targeted delivery approach reduces the quantity of antimicrobials required for successful treatment (Sivaraj et al. 2018).
Antimicrobial film coatings, nanofibers, nanoliposomes, and nanoparticle systems were primarily used for encapsulation of bacteriocins (Chandrakasan et al. 2019). At present, inorganic nanoparticles like silver, copper, zinc, and gold are not considered solely for their antibacterial effect as well as their diverse potential biomedical applications (Naskar and Kim 2021 et al. 2021).
Two major nanoparticles containing silver and gold have exhibited considerable antibacterial effects over a large spectrum of Gram-negative and Gram-positive bacteria (Ravishankar Rai and Jamuna Bai 2011). Silver nanoparticles coated with synthesized enterocine (En-SNPs) indicated wide-spectrum inhibition against some foodborne pathogens (Dhanam et al. 2021). The potential activity of these specific nanoparticles over pathogenic microorganisms is due to their good affinity towards phosphorus and sulfur. Generally, the interaction of silver nanoparticles with sulfur-containing amino acids existing on bacterial cell membranes leads to a change in the permeability of bacterial cells (Méndez-Vilas 2023). In addition, enterocin combined with Ag-NPs showed high efficiency against different food pathogenic microorganisms, including S. aureus, E. coli, and L. monocytogenes (Sharma et al. 2012). Conjugation of silver nanoparticles and bacteriocin has been shown to be more efficient against different food pathogens (Sidhu and Nehra 2020).
The encapsulation of pediocin by hydrating the film in vesicular nanocarriers was effectively performed using liposomes prepared with soybean lecithin and an inhibition established against different Listeria species (Jiao et al. 2020). The size of liposomes varies from micrometers to nanometers. In both hydrophilic and hydrophobic compounds, liposomes, along with suitable encapsulants, are non-toxic and biodegradable agents (Yousefi et al. 2023, Kheiriabad et al. 2024).
In bacteriocins distributed in a condensed form, like encapsulation in nanoparticles, the efficacy and potentially toxic side effects are maximized and minimized, respectively (Zimina et al. 2020). In brief, the synthesis of nanostructures through the nanotechnology approach has made this approach a promising way to increase bacteriocin stability for food products. For instance, nanoencapsulation increases the shelf life of food through bacteriocin protection against the inactivation of proteolytic and lipolytic enzymes (Terra et al. 2022). Incorporating bacteriocins into condensed forms, such as nanoparticles, not only reduces the risk of toxic side effects but also enhances their effectiveness and broadens the application spectrum of bacteriocins in preserving food from spoilage microorganisms. In summary, ongoing research and innovation in the field of bacteriocins offer new perspectives in their classification, synthesis, mode of action, and application in the food industry. By addressing their limitations and capitalizing on nanotechnology, bacteriocins have the potential to revolutionize food preservation and safety.
Bacteriocin in food preservation
According to a study by Zhang et al. (2022), Class II bacteriocins can be directly used as food preservatives. Furthermore, certain bacteriocins, such as AS-48, have potential as alternative antibiotics through the development of bacteriocin-based therapies, offering a promising solution to antibiotic resistance. In general, LAB strains use a QS system to coordinate the production of class IIa and IIb bacteriocins at an appropriate growth stage. The regulatory mechanisms of genes responsible for class IIc and IId bacteriocin biosynthesis still require further investigation. The potential use of bacteriocins as natural food preservatives depends on the ability of genetically modified heterologous host strains to express bacteriocin genetic determinants at an industrial scale. Currently, commercial-scale bacteriocin production is limited by high costs and low yields (Zhang et al. 2022).
In recent years, food safety and quality concerns have increased attention on the use of bacteriocins as biopreservatives (Porto et al. 2017). According to recent studies, bacteriocin originating from LAB has an important preventing activity against spoilage pathogenic and spoilage microorganisms in food (Zommiti et al. 2018). Bacteriocins, were used typically in fermented and non-fermented foods for many decades. Therefore, this made them suitable for use in health applications (Daba and Elkhateeb 2020). Bacteriocins, which are incorporated into food products, often encompass a combination of antimicrobial compounds. Table 3 demonstrates the antibacterial activities of bacteriocins in foods.
Type of bacteriocin . | Inhibitory activity . | Base . | Source . | Pathogenic microorganism . | Main results . | Reference . |
---|---|---|---|---|---|---|
Enterocin | 6400 and 400 AU/ml | Raw camel milk | Enterococcus faecium | Listeria monocytogenes | Good anti listerial activity | Rahmeh et al. (2018) |
Lantibiotic bacteriocins and durancin ED26E/7 | - | Goat milk | Enterococcus faecium CCM 4231 | Enterococcal species | Low-grade biofilm formation ability | Lauková et al. (2021) |
Enterocin A | 10 and 100 AU/ml | Media culture | Enterococcus faecium CTC492 | Listeria monocytogenes | Bactericidal mode of action | Yang and Moon (2021) |
Enterocin | 3200 AU/ml | Traditional sourdough | Lactobacillus plantarum | Staphylococcus aureus, E. coli, and L. monocytogenes | Reduction for 24h | Zangeneh et al. (2020) |
Enterocin | 2 048 AU/g cheese | Fresh cheese | Enterococcus faecalis | Listeria monocytogenes | Reduction for 6–72 h | Ribeiro (2018) |
Pediocin PA-1 | 5000 BU/ml | Spanish dry-fermented frankfurters | Pediococcus acidilactici MCH14 | Listeria monocytogenes | Reduction 2 log for 60 days at 4°C-Reduction 0.6 log for 30 days at 15°C | Nieto-Lozano et al. (2010) |
Pediocin PA-1 | 5000 BU/ml | Spanish sausage | Pediococcus acidilactici MCH14 | Listeria monocytogenes | Reduction 2 log | Nieto-Lozano et al. (2010) |
Pediocin PA-1 | 5000 BU/m | Frankfurters | Pediococcus acidilactici MCH14 | Clostridium perfringens | Reduction 2 log for 60 days at 10°C—Reduction 0.8 log for 30 days at 15°C | Nieto-Lozano et al. (2010) |
Sactipeptid (SacP23) | - | Marine sponge | Bacillus subtilis BS34A | Staphylococcus aureus | Extensive spectrum antimicrobial activity against Gram-negative and Gram-positive bacteria | Khánh et al. 2023) |
Novel bacteriocin | - | Spoiled fruits and vegetable wastes | Lysinibacillus | Bacillus pumilus | High activity against foodborne bacterial pathogens, Act as a bio-preservative agent against different food-borne pathogens. | Ahmad et al. (2019) |
Pediocin SA-1 | 6 320 BU/ml | Maize silages | Pediococcus acidilactici | Listeria monocytogenes | Could be used as an additive to control the presence of L. monocytogenes in maize silages selectively, while improving their fermentative quality and eventually their aerobic stability. | Amado et al. (2016) |
Novel bacteriocin | Fruits and vegetable waste | Lysinibacillus | Staphylococcus aureus, (22 mm ZOI), S. epedermidis and B. cereus | Used as an alternative food preservative or therapeutic agent to control spoilage of different food products. | Ahmad et al. (2014) |
Type of bacteriocin . | Inhibitory activity . | Base . | Source . | Pathogenic microorganism . | Main results . | Reference . |
---|---|---|---|---|---|---|
Enterocin | 6400 and 400 AU/ml | Raw camel milk | Enterococcus faecium | Listeria monocytogenes | Good anti listerial activity | Rahmeh et al. (2018) |
Lantibiotic bacteriocins and durancin ED26E/7 | - | Goat milk | Enterococcus faecium CCM 4231 | Enterococcal species | Low-grade biofilm formation ability | Lauková et al. (2021) |
Enterocin A | 10 and 100 AU/ml | Media culture | Enterococcus faecium CTC492 | Listeria monocytogenes | Bactericidal mode of action | Yang and Moon (2021) |
Enterocin | 3200 AU/ml | Traditional sourdough | Lactobacillus plantarum | Staphylococcus aureus, E. coli, and L. monocytogenes | Reduction for 24h | Zangeneh et al. (2020) |
Enterocin | 2 048 AU/g cheese | Fresh cheese | Enterococcus faecalis | Listeria monocytogenes | Reduction for 6–72 h | Ribeiro (2018) |
Pediocin PA-1 | 5000 BU/ml | Spanish dry-fermented frankfurters | Pediococcus acidilactici MCH14 | Listeria monocytogenes | Reduction 2 log for 60 days at 4°C-Reduction 0.6 log for 30 days at 15°C | Nieto-Lozano et al. (2010) |
Pediocin PA-1 | 5000 BU/ml | Spanish sausage | Pediococcus acidilactici MCH14 | Listeria monocytogenes | Reduction 2 log | Nieto-Lozano et al. (2010) |
Pediocin PA-1 | 5000 BU/m | Frankfurters | Pediococcus acidilactici MCH14 | Clostridium perfringens | Reduction 2 log for 60 days at 10°C—Reduction 0.8 log for 30 days at 15°C | Nieto-Lozano et al. (2010) |
Sactipeptid (SacP23) | - | Marine sponge | Bacillus subtilis BS34A | Staphylococcus aureus | Extensive spectrum antimicrobial activity against Gram-negative and Gram-positive bacteria | Khánh et al. 2023) |
Novel bacteriocin | - | Spoiled fruits and vegetable wastes | Lysinibacillus | Bacillus pumilus | High activity against foodborne bacterial pathogens, Act as a bio-preservative agent against different food-borne pathogens. | Ahmad et al. (2019) |
Pediocin SA-1 | 6 320 BU/ml | Maize silages | Pediococcus acidilactici | Listeria monocytogenes | Could be used as an additive to control the presence of L. monocytogenes in maize silages selectively, while improving their fermentative quality and eventually their aerobic stability. | Amado et al. (2016) |
Novel bacteriocin | Fruits and vegetable waste | Lysinibacillus | Staphylococcus aureus, (22 mm ZOI), S. epedermidis and B. cereus | Used as an alternative food preservative or therapeutic agent to control spoilage of different food products. | Ahmad et al. (2014) |
Type of bacteriocin . | Inhibitory activity . | Base . | Source . | Pathogenic microorganism . | Main results . | Reference . |
---|---|---|---|---|---|---|
Enterocin | 6400 and 400 AU/ml | Raw camel milk | Enterococcus faecium | Listeria monocytogenes | Good anti listerial activity | Rahmeh et al. (2018) |
Lantibiotic bacteriocins and durancin ED26E/7 | - | Goat milk | Enterococcus faecium CCM 4231 | Enterococcal species | Low-grade biofilm formation ability | Lauková et al. (2021) |
Enterocin A | 10 and 100 AU/ml | Media culture | Enterococcus faecium CTC492 | Listeria monocytogenes | Bactericidal mode of action | Yang and Moon (2021) |
Enterocin | 3200 AU/ml | Traditional sourdough | Lactobacillus plantarum | Staphylococcus aureus, E. coli, and L. monocytogenes | Reduction for 24h | Zangeneh et al. (2020) |
Enterocin | 2 048 AU/g cheese | Fresh cheese | Enterococcus faecalis | Listeria monocytogenes | Reduction for 6–72 h | Ribeiro (2018) |
Pediocin PA-1 | 5000 BU/ml | Spanish dry-fermented frankfurters | Pediococcus acidilactici MCH14 | Listeria monocytogenes | Reduction 2 log for 60 days at 4°C-Reduction 0.6 log for 30 days at 15°C | Nieto-Lozano et al. (2010) |
Pediocin PA-1 | 5000 BU/ml | Spanish sausage | Pediococcus acidilactici MCH14 | Listeria monocytogenes | Reduction 2 log | Nieto-Lozano et al. (2010) |
Pediocin PA-1 | 5000 BU/m | Frankfurters | Pediococcus acidilactici MCH14 | Clostridium perfringens | Reduction 2 log for 60 days at 10°C—Reduction 0.8 log for 30 days at 15°C | Nieto-Lozano et al. (2010) |
Sactipeptid (SacP23) | - | Marine sponge | Bacillus subtilis BS34A | Staphylococcus aureus | Extensive spectrum antimicrobial activity against Gram-negative and Gram-positive bacteria | Khánh et al. 2023) |
Novel bacteriocin | - | Spoiled fruits and vegetable wastes | Lysinibacillus | Bacillus pumilus | High activity against foodborne bacterial pathogens, Act as a bio-preservative agent against different food-borne pathogens. | Ahmad et al. (2019) |
Pediocin SA-1 | 6 320 BU/ml | Maize silages | Pediococcus acidilactici | Listeria monocytogenes | Could be used as an additive to control the presence of L. monocytogenes in maize silages selectively, while improving their fermentative quality and eventually their aerobic stability. | Amado et al. (2016) |
Novel bacteriocin | Fruits and vegetable waste | Lysinibacillus | Staphylococcus aureus, (22 mm ZOI), S. epedermidis and B. cereus | Used as an alternative food preservative or therapeutic agent to control spoilage of different food products. | Ahmad et al. (2014) |
Type of bacteriocin . | Inhibitory activity . | Base . | Source . | Pathogenic microorganism . | Main results . | Reference . |
---|---|---|---|---|---|---|
Enterocin | 6400 and 400 AU/ml | Raw camel milk | Enterococcus faecium | Listeria monocytogenes | Good anti listerial activity | Rahmeh et al. (2018) |
Lantibiotic bacteriocins and durancin ED26E/7 | - | Goat milk | Enterococcus faecium CCM 4231 | Enterococcal species | Low-grade biofilm formation ability | Lauková et al. (2021) |
Enterocin A | 10 and 100 AU/ml | Media culture | Enterococcus faecium CTC492 | Listeria monocytogenes | Bactericidal mode of action | Yang and Moon (2021) |
Enterocin | 3200 AU/ml | Traditional sourdough | Lactobacillus plantarum | Staphylococcus aureus, E. coli, and L. monocytogenes | Reduction for 24h | Zangeneh et al. (2020) |
Enterocin | 2 048 AU/g cheese | Fresh cheese | Enterococcus faecalis | Listeria monocytogenes | Reduction for 6–72 h | Ribeiro (2018) |
Pediocin PA-1 | 5000 BU/ml | Spanish dry-fermented frankfurters | Pediococcus acidilactici MCH14 | Listeria monocytogenes | Reduction 2 log for 60 days at 4°C-Reduction 0.6 log for 30 days at 15°C | Nieto-Lozano et al. (2010) |
Pediocin PA-1 | 5000 BU/ml | Spanish sausage | Pediococcus acidilactici MCH14 | Listeria monocytogenes | Reduction 2 log | Nieto-Lozano et al. (2010) |
Pediocin PA-1 | 5000 BU/m | Frankfurters | Pediococcus acidilactici MCH14 | Clostridium perfringens | Reduction 2 log for 60 days at 10°C—Reduction 0.8 log for 30 days at 15°C | Nieto-Lozano et al. (2010) |
Sactipeptid (SacP23) | - | Marine sponge | Bacillus subtilis BS34A | Staphylococcus aureus | Extensive spectrum antimicrobial activity against Gram-negative and Gram-positive bacteria | Khánh et al. 2023) |
Novel bacteriocin | - | Spoiled fruits and vegetable wastes | Lysinibacillus | Bacillus pumilus | High activity against foodborne bacterial pathogens, Act as a bio-preservative agent against different food-borne pathogens. | Ahmad et al. (2019) |
Pediocin SA-1 | 6 320 BU/ml | Maize silages | Pediococcus acidilactici | Listeria monocytogenes | Could be used as an additive to control the presence of L. monocytogenes in maize silages selectively, while improving their fermentative quality and eventually their aerobic stability. | Amado et al. (2016) |
Novel bacteriocin | Fruits and vegetable waste | Lysinibacillus | Staphylococcus aureus, (22 mm ZOI), S. epedermidis and B. cereus | Used as an alternative food preservative or therapeutic agent to control spoilage of different food products. | Ahmad et al. (2014) |
The use of pediocins in food may offer a good alternative to protect it from foodborne pathogens such as L. monocytogenes, Pseudomonas, E. coli, and S. aureus (Silva et al. 2018). Pediocin PA-1 is synthesized by P. acidilactici isolated from American sausages. Sakacin, curvacin A, and enterocin A, which were produced by Lb. sakei, Lb. curvatus, and E. faecium, respectively, were isolated from fermented sausages and meat. These bacteriocins indicated significant inhibitory effects against Clostridium spp. and L. monocytogenes (Ranaei et al. 2021). In the meat industry, pediocin PA-1 or its producing culture, which inhibits the growth of spoilage microorganisms through the storage period, is commercially used as a fermented powder containing bacteriocin (Leroy and De Vuyst 2010). The pediocin PA-1/AcH, which is produced by Pediococcus pentosaceus BCC 3772, when used as a starter culture for Nham (a traditional Thai fermented pork sausage), was effective in controlling the growth of L. monocytogenes without affecting the quality of Nham (Perez et al. 2014).
Another approach to controlling L. monocytogenes in meat and poultry products can be the use of pediocin in food packaging film (H. Hosseini et al. 2024). The extract of pediocin PA-1 from milk-based media and anti-listerial cellulose casings prepared by internal coating with the pediocin powder has a bactericidal effect against L. monocytogenes through 12 weeks (Woraprayote et al. 2016). When the pediocin PA-1/Ac has been incorporated in a packaging film, the initial load of L. monocytogenes on the surface of meat has been significantly reduced (López-Córdoba 2018).
Enterocins are generated by Enterococcus species and include a variety of bacteriocins that may be used as starter cultures, antimicrobial cultures, and by direct addition of raw or purified preparations in the fermentation process (Balciunas 2013, Kyriakou et al. 2016). While enterocins have not been approved for use commercially, they could prevent the growth of certain Gram-positive bacteria, like Staphylococcus spp., Clostridium spp., Listeria spp., Bacillus spp., Enterococci spp., and Gram-negative pathogens (Vibrio cholerae, E. coli) (Radaic et al. 2020).
Enterococci exist in certain dairy products because they are used as starter cultures, and they are found more specifically in cheese, where they are naturally occurring (Domingos-Lopes et al. 2017, Pilevar and Hosseini 2017). Unlike most LAB that have the GRAS status, the risk associated with the use of some enterococci in food is high (infections in humans and antibiotic resistance genes). So, it seems that the usage of enterocins can be more appropriate for food consumption (Kyriakou et al. 2016, Bindu and Lakshmidevi 2021). The usage of enterocins in milk products could be a natural barrier to microorganisms growth (Abengózar et al. 2017).
Recent studies have shown the inhibiting effect of enterocin, which is produced by enterococci strains against L. monocytogenes in raw milk (Taghizadeh et al. 2017, Heeney et al. 2019, Taghizadeh et al. 2022). Enterocin CCM 4231 from E. faecium CCM 4231 demonstrated significant hindering effects versus L. monocytogenes in yogurt and S. aureus in skim milk yogurt (Hammami et al. 2019). Enterosin A, which belongs to class IIa bacteriocins and is isolated from E. faecium CTC492, showed antibacterial effects against 13 strains of L. monocytogenes (Al Kassaa et al. 2021; Yang and Moon 2021). It was reported that when enterocin was added to soy milk, L. monocytogenes Ohio strain was eliminated after 24 h (Engstrom 2021). The effectiveness of semi-purified enterocin produced by the E. faecalis strain to decrease the infection of cheese samples by L. monocytogenes in the duration of storage (72 h) has been proven.
The supplementation of E. faecium CTC492 enterocins A and B has successfully inhibited L. innocua in various kinds of meat-based foods (Favaro and Todorov 2017). In meat products, the effectiveness of enterocin against special pathogens, particularly L. monocytogenes has been proven (Ribeiro et al. 2017). The use of enterocins in meat preservation has high potential, particularly since nisin is not efficient as a preservative in meat products (Khelissa et al. 2021). Enterocins show high activity, especially for Listeria species in low concentrations (Table 3) (Schittler et al. 2019, Hosseini et al. 2021).
Conclusion
Bacteriocins exhibit substantial potential as antimicrobial agents in the food industry, addressing both food spoilage and pathogenic microorganisms, thereby enhancing food safety. Pediocin-like and two-peptide bacteriocins, with their unique antimicrobial mechanisms and biosynthetic pathways, have garnered considerable attention for their industrial applications. The chemical synthesis of these bacteriocins, particularly through the Fmoc-SPPS method, has proven effective in meeting the demand for bioactive compounds, facilitating their large-scale application. Despite the promising properties of bacteriocins, several challenges, such as purification post-microbial fermentation and ensuring stability in various environmental conditions, hinder their widespread adoption. However, advancements in nanotechnology offer innovative solutions to these challenges. Techniques such as nanoencapsulation can protect bacteriocins from enzymatic degradation, enhance their stability, and extend their antimicrobial spectrum. This not only increases their effectiveness but also reduces the risk of toxic side effects.
Ongoing research and innovation in the field of bacteriocins are crucial for overcoming existing limitations and unlocking their full potential. By integrating nanotechnology and exploring new variants, bacteriocins can revolutionize food preservation and safety, offering a sustainable and effective alternative to conventional chemical preservatives.
Acknowledgments
The authors gratefully acknowledge Department of Food Science and Technology, Tabriz University of Medical Sciences and University of Tabriz.
Author contributions
Sara Bahrami (Conceptualization, Investigation, Writing – original draft, Writing – review & editing), Hashem Andishmand (Formal analysis, Validation, Writing – original draft, Writing – review & editing), Zahra Pilevar (Supervision, Validation, Writing – review & editing), Fataneh Hashempour-baltork (Conceptualization, Investigation, Validation, Writing – original draft, Writing – review & editing), Mohammadali Torbati (Investigation, Validation, Writing – original draft, Writing – review & editing), Manouchehr Dadgarnejad (Conceptualization, Data curation, Writing – original draft, Writing – review & editing), Hossein Rastegar (Data curation, Formal analysis, Writing – original draft, Writing – review & editing), Seyed Ali Mohammadi (Data curation, Formal analysis, Writing – original draft, Writing – review & editing), and Sodeif Azadmard-Damirchi (Conceptualization, Formal analysis, Writing – original draft, Writing – review & editing)
Conflict of interest
None declared.
Funding
This study has been supported by Halal Research Center of IRI, Iran Food and Drug Administration, Ministry of Health and Medical Education, Tehran, Iran.
Data availability
No new data were generated or analysed in support of this research.