-
PDF
- Split View
-
Views
-
Cite
Cite
Praveen Ranadev, Ashwin Revanna, Davis Joseph Bagyaraj, Ambika H Shinde, Sulfur oxidizing bacteria in agro ecosystem and its role in plant productivity—a review, Journal of Applied Microbiology, Volume 134, Issue 8, August 2023, lxad161, https://doi.org/10.1093/jambio/lxad161
- Share Icon Share
Abstract
Sulfur (S) deficiency is becoming more common in agro-ecosystems worldwide due to factors such as agronomic practices, high biomass production, reduced sulfur emissions, and the use of non-sulfur fertilizers. This review explores the natural occurrence and commercial exploitation of sulfur pools in nature, the mineralization and immobilization of sulfur, the physiological role of sulfur in plants, and its deficiency symptoms. Additionally, the organic and inorganic forms of sulfur in soil, their transformations, and the process of microbiological oxidation of sulfur are discussed. The review also addresses the diversity of sulfur-oxidizing bacteria (SOB) and the various biochemical mechanisms involved in their role in plant productivity and soil reclamation. The measurement of S oxidation rate in soil and the variables that influence the process are also examined. Typically, the rate of oxidation of added elemental S is around 40%–51%, which is available for plant uptake. These characteristics of SOB demonstrate their potential as bioinoculants for increasing plant growth, indicating their use as biofertilizers for sustainable crop production in agro-ecosystems.
Introduction
Sulfur (S) is an essential plant nutrient that is considered just as important as nitrogen, phosphorus, and potassium for the growth of plants. Its importance in agriculture has been recognized for more than a century (Sharma et al. 2022). Sulfur is particularly critical for oilseed and pulse crops, with a recommended application rate of 75 and 25 kg ha−1, respectively. Cereal crops such as rice and corn also benefit from S, with a recommended application rate of 40 kg ha−1. Unfortunately, the continuous use of non-S-containing nitrogen (N), phosphorus (P) and potassium (K) fertilizers, reduced use of S-containing pesticides, and greater export of S from soil to crop growth, combined with reduced S input through rainwater and a decrease in sulfur dioxide (SO2) emissions from fossil fuels to the atmosphere, have led to an increase in S deficiency in soils (Camberato and Casteel 2017). In India, nearly 46% of soils are deficient in sulfur, resulting in 20%–40% lower crop yields (https://www.sulfurinstitute.org/abo, accessed 27 January 2023). In some soils of specific areas, such as the Brazilian cerrado, S deficiency can occur naturally (Ribeiro et al. 2001).
To ensure an adequate supply of S for proper crop growth and yield, sustainable agricultural management practices are necessary. This requires an understanding and quantification of S transformations in the soil, including the predominant microbial processes such as S immobilization, mineralization, oxidation, and reduction. One cost-effective way to replenish S lost from the soil is to use elemental sulfur (S0) as fertilizer, which also allows for the application of concentrated commercial forms of NPK fertilizers (Valle et al. 2019). However, S0 replenishment must be carefully adjusted to meet the plant's demand for sulfate (SO42−) in a short period while avoiding soil and water pollution. The oxidation of S0 depends on microbial diversity, soil conditions, and environmental factors (Fuentes-Lara et al. 2019). This review article discusses the natural occurrence of sulfur in soil, the general transformations of S, the physiological role of sulfur in plants, the diversity and microbiological oxidation of various forms of reduced S, methodologies for quantifying sulfur oxidation in soil, factors that influence S oxidation in soil, and the implications of using sulfur-oxidizing bacteria (SOB) as plant growth promoters in the agro-ecosystem.
Natural occurrence and commercial exploitation of sulfur
Sulfur is the 17th most prevalent element in the crust out of the 105 elements that make up the crust, with a measured concentration of roughly 0.06%–0.10%. The chemical element sulfur has the molecular formula S8 and is non-metallic. It is found in three different natural forms: alpha (yellow), beta (brown), and gamma (pale yellow), and it is soluble in organic solvents to varied degrees but insoluble in water (Germida et al. 2021). Salt domes, bedded evaporites, and volcanic deposits are all places where native sulfur can be found. It may also be connected to sulfate minerals like anhydrite, barite, and gypsum, as well as sulfides like chalcopyrite, pyrrhotite, sphalerite, galena, and pyrite (FeS2). Sulfur is also present in natural gas, oil, coal, bitumen sands, pyrobitumen shale, and as hydrogen sulfide (H2S) in anoxic soils, as well as a by-product of industrial activities (Germida et al. 2021). FeS2, a naturally occurring iron disulfide mineral, is the prime source of sulfur in soils. S from FeS2 undergoes oxidation to many forms during weathering and soil formation, and these forms are intimately related to the redox potential of the soil. Sulfur is easily reduced and oxidized, which causes a wide range of reactions in the soil (Schoenau and Malhi 2008).
Mineralization and immobilization of sulfur in soil
The balance between the immobilization of soluble S and the mineralization of organic S affects the amount of S in the soil. Immobilization and mineralization are influenced by a number of factors, such as organic matter in the soil, moisture, pH, the presence of plants, cultivation season, management style, microbial diversity, and soil enzyme activity (Hassan 2022). Plants often have easy access to sulfur in soil from soil organic matter with a C:S ratio of >400, while organic residues with a C:S ratio of 200–400 have no discernible impact on the concentration of SO42− in the soil solution. Animal and vegetable wastes, soil microbial biomass and metabolites, and humus are a few examples of the several sources of mineralizable organic S (Dick et al. 2008).
Various forms of sulfur in agro-ecosystem
Many organic and inorganic forms of sulfur may be found in the soil. Organic forms of sulfur predominate in well-drained, upland agricultural soils, while inorganic sulfate (SO42−) is the main inorganic sulfur form. The total amount of sulfur in soils ranges from 19 to 4000 mg kg−1 and can be found in both organic and mineral forms. The overall sulfur content, however, is typically of minimal significance for the soil's immediate fertility (Dick et al. 2008).
Organic forms of soil sulfur in the agro-ecosystem
Even though the inorganic sulfate (SO42−) fraction is easily accessible to roots, it accounts for <5% of total sulfur in the soil on an average. The significant proportion of sulfur in soil (>95%) is bound to organic molecules, making it available to plants indirectly via oxidation and mineralization (Srinivasarao et al. 2004). Organic sulfur in soil is divided into three main categories: (a) organic sulfur not directly bound to carbon (C), which is reduced to H2S by hydriodic acid; (b) organic sulfur directly bound to C (C–S), which is reduced to H2S by Raney nickel; and (c) residual C-bonded sulfur. The first type of organic sulfur that is not directly bound to C is sulfate esters (C–O–S), which include phenol sulfate, sulfated lipids, and sulfated polysaccharides. S-containing amino acids and also thiols, disulfides, sulfones and sulfonic acids, make up the majority of the second group of organic sulfur (C–S). Sulfonates, sulfoxides, and heterocyclic S are most likely the third group of organic sulfur (Schmidt et al. 2012).
Recent fractionation studies have separated organic sulfur into five fractions based on its oxidation state, using sulfur K-edge X-ray absorption near-edge spectroscopy (XANES) directly on soil samples or humic fractions. Two reduced S fractions (including mono, di, and polysulfides as the first fraction and thiols and thiophenes as the second fraction), two other S fractions in intermediate oxidation states (sulfoxides and sulfonates), and a fifth fraction as sulfate esters (Stellhorn et al. 2022). The sulfur fraction distribution in the soil varies from region to region and depends on various factors such as type of the soil, climate, organic matter, rainfall, temperature, etc. (Hassan 2022). In India, the average values of different fractions of S in the soil profile range from 241 to 391 mg kg−1 with total S ranging from 191 to 362 mg kg−1, organic S ranging from 12.9 to 59.0 mg kg−1, and adsorbed S ranging from 3.5 to 9.2 mg kg−1 for available S. Vertisols had the highest levels of organic and total S, followed by Inceptisols and Alfisols, with Alfisols having the highest levels of adsorbed S. In tropical pasture soils, organic S estimated to account for 97%–99% of the total S content, making it the most significant S pool (Srinivasarao et al. 2004).
Inorganic forms of sulfur in agro-ecosystem soil
Sulfur occurs in various oxidation states in nature, ranging from -2 to +6, with sulfide (S2−) being the most reduced and sulfate (SO42−) the most oxidized form (Schmidt et al. 2012). Elemental sulfur (S0) is produced by the oxidation of H2S and is the most stable form of S (Narayan et al. 2022). Inorganic S exists in soil mainly as sulfate, which is available to plants in the soil solution. Under anaerobic conditions, S is found in reduced forms. In calcareous and saline soils, a significant proportion of S is present as gypsum (CaSO4 2H2O). Arid regions may accumulate high levels of salts such as CaSO4, MgSO4, and Na2SO4.
The application of chemical fertilizers is the primary source of S input into soil, with sulfur fertilizers being the most common and soluble form. Elemental sulfur (S0) is the most concentrated and insoluble S source, containing 100% S and being either linked to bentonite (90% S) or suspended in clay (40%–60% S) (Schmidt et al. 2012). Some fertilizers, such as S0-coated urea containing 10%–25% S, can be developed in the future to supply sulfur. Soil losses of inorganic S occur via a variety of mechanisms, including sorption, leaching, erosion, crop exportation, and, to a lesser extent, volatilization. Volatilization losses in inundated fields may be greater due to microbial reduction of oxidized S forms to volatile H2S (Schoenau and Malhi 2008).
Physiological role of sulfur in plants
Sulfur plays an essential role in the biosynthesis of the amino acids methionine, cystine, and cysteine, which are crucial for both plant and animal life. On a dry weight basis, the sulfur content in plant biomass can vary between 0.05% and 0.5%, with decreasing concentrations observed in plants belonging to the Cruciferae, Leguminosae, and Gramineae families. The role of Sulfur can be considered in two ways: either directly involved in the synthesis of biomolecules or indirectly by affecting the synthesis of compounds or other nutrients. Crop plants mainly rely on sulfate as their primary sulfur source, which is activated by forming adenosine-5′-phosphosulfate (APS), followed by active sulfate, PAPS (3′-phosphoadenosine-5′-phosphosulfate) (Houhou et al. 2018).
Cysteine serves as the precursor of both methionine and cystine, two important sulfur-containing amino acids used in the construction of proteins. These amino acids help to maintain the unique structure of each protein by forming disulfide bonds (–S–S–). Enzymes are a type of protein, and the formation of these S–S bridges is crucial for their proper conformation, which in turn is necessary for their activity. In plants, sulfolipids (specifically, diacylphoquinivosylglycerol) are a common component of leaf tissue, particularly within chloroplasts and their lamellar membranes. This suggests a close connection with the activity of photosystem I. The roles of sulfolipids in plants are many and varied, but can be broadly described as facilitating the electron transport chain, aiding in the synthesis of important pigments such as flavonoids and carotenoids, and overall contribution to the process of photosynthesis (Houhou et al. 2018). Additionally, sulfur is also important for the synthesis of vitamins such as biotin and thiamine. Biotin, also known as vitamin H or B7, contains a sulfur-containing ring and is involved in several metabolic reactions. Thiamine, or vitamin B1, also contains sulfur and is important for energy metabolism in both plants and animals. Sulfur also plays a role in the defense mechanisms of plants by contributing to the synthesis of phytoalexins, which are compounds produced by plants in response to biotic or abiotic stresses (Narayan et al. 2022).
Ferredoxin is classified as an iron-sulfur enzyme, a type of enzyme that is characterized by containing equal numbers of iron and sulfur atoms but no other groups. These enzymes are important in electron transfer reactions in a wide range of organisms, including plants, animals, and bacteria. In particular, sulfur plays a key role in the nitrogenase enzyme complex (which includes ferredoxin iron-sulfur protein), which catalyzes the fixation of nitrogen by transferring electrons from NADPH (nicotinamide adenine dinucleotide phosphate) (Scherer et al. 1988). Additionally, sulfur has been shown to play a role in the TCA cycle and glycolysis. It also contributes to the synthesis of various lipids, including sulfolipids, ceramide sulfonic acid, sterol sulfur ester, phosphatidylsulfocholine, and chlorosulfolipids (Nakai and Maruyama-Nakashita 2020)). Sulfur is also an important component in the synthesis of polyamines, which have many biochemical and physiological functions. Polyamines are involved in regulating the physical and chemical properties of cell membranes, modulating enzyme activities, and regulating the synthesis of molecules such as nucleic acids. They also play a role in physiological processes such as growth and differentiation, the regulation of hormone-mediated responses, and the retardation of senescence (Nakai and Maruyama-Nakashita 2020).
Certain secondary metabolites containing sulfur, such as glucosinolates, isothiocyanates, thiocyanate, and nitriles, have been shown to act as insect repellents and disease control agents. Glucosinolates are non-volatile and can only be recognized by insects upon contact, while isothiocyanates are generally volatile and can trigger olfactory responses (Nakai and Maruyama-Nakashita 2020). Enzymatically formed isothiocyanates from sinigrin, which result from tissue damage, have been found to have anti-bacterial, anti-fungal, anti-insecticidal, and anti-nematicidal properties (Oh et al. 2018, Tarar and Peng 2022). Allylisothiocyanate, the acrid and sharp-tasting component of mustard, is consumed in small amounts as a table condiment. Isothiocyanate is also a major flavor component in cruciferous vegetables such as cabbage. Sulfonium derivatives, S-methyl sulfonium propanoic acid and S-dimethyl sulfonium pentanoic acid are sulfur-containing plant metabolites that confer saline tolerance in some plants (Tarar and Peng 2022). The effect of sulfur on other mineral nutrient uptake is not fully understood, some experiments have shown that it has a positive effect on nitrogen, phosphorus, zinc, and iron uptake (Salvagiotti et al. 2009, Tabak et al. 2020, Chaudhary et al. 2022).
Sulfur deficiency and deficiency symptoms in plants
Sulfur deficiency is a crucial growth-limiting factor for crop plants, as sulfur is either immobile or only moderately mobile in plant tissue. Therefore, a continuous supply of sulfur is necessary for proper growth and development (Scherer et al. 1988). In tropical regions, sulfur deficiency in soil is commonly found in Andosols, Vertisols, Alfisols, Ultisols, and Oxisols. In Asia, sulfur deficiency has been reported in wetland rice from Bangladesh, Burma, India, Indonesia, Japan, the Philippines, and Sri Lanka. The plant-available form of sulfur is negatively charged, which means it can be easily leached out of plant root zones with precipitation or irrigation. Therefore, sulfur-containing fertilizers are typically recommended to alleviate sulfur deficiency in crops (Tabak et al. 2020). The use of low-sulfur fertilizers, a decrease in organic manure usage, intensive cropping, and reduced atmospheric deposition have all contributed to an increase in sulfur deficiencies (Camberato and Casteel 2017). Under field conditions, sulfur deficiency can be assessed by measuring the ratio between chloride, phosphorus, and sulfur [(Cl)+(P):(S)] content in the mature leaves of plants (Etienne et al. 2018).
Sulfur is an essential component of the enzyme nitrate reductase, which plays a critical role in the conversion of nitrate to organic nitrogen. Consequently, sulfur deficiency can disrupt nitrogen metabolism, leading to symptoms that resemble nitrogen deficiency in many crops. Additionally, sulfur is necessary for chlorophyll synthesis, and thus, the symptoms of sulfur deficiency are typically observed initially in younger leaves (Houhou et al. 2018). However, the deficiency symptoms are usually not severe and do not localize to the older leaves. A lack of sulfur in the plant typically results in a light green coloration throughout the entire plant. Legumes, particularly alfalfa, have a high sulfur requirement, and therefore, deficiencies tend to appear first in these crops. In corn, sulfur deficiency can sometimes resemble other nutrient deficiencies, such as manganese or magnesium, by causing interveinal chlorosis, with the upper leaves appearing striped and the veins remaining darker green than the area between the veins (Gao et al. 2016).
Diversity of SOB in soil
The microbiology of biological oxidation of sulfur compounds and biological removal of sulfides in agroecosystems is primarily based on the activities of diverse microflora, especially SOB groups (Rana et al. 2020). These groups can be broadly classified into three major categories: phototrophs, chemolithotrophs, and heterotrophs, each containing a variety of SOB (see Table 1). Phototrophic SOB obtain energy for their metabolism from light, while chemolithotrophic SOB meet their energy requirements by oxidizing reduced sulfur compounds. Based on the electron acceptor during the oxidation of sulfides, SOB can be classified as aerobic (with oxygen as the terminal electron acceptor) or anoxic/anaerobic (with nitrates or nitrites as the terminal electron acceptor). Among the three groups, chemolithotrophic SOB, also known as colorless SOB is the most dominant and effective sulfur oxidizers, which includes several species of Thiobacillus, Sulfolobus, Thermothrix, Beggiatoa, and Thiothrix (Tourna et al. 2014). Due to their high rate of sulfide oxidation, modest nutritional requirements, and extremely high affinity for sulfides and oxygen, they can successfully compete for the oxidation of sulfides in both natural environments and bioreactors with a limited supply of oxygen (Anandham et al. 2011).
1 | Photolithotrophs (GSB) Energy—Light C source- CO2 | Chlorobium limicola Chlorobium tepidum Chromatium Chloroherpeton, Prosthecochloris, Pelodictyon, Ancalochloris Ectothiorhodospira spp. Cgloroflexus aurantiacus Oscillatoria sp. Lynbya spp. Microcoleus spp. Phormidium spp. Aphanothece spp. Rhodopseudomonas spp. | Starkey 1935, Kuenen and Beudeker 1982, Tang et al. 2009, Sakurai et al. 2010, Lucheta and Lambais 2012, Simon and Kroneck 2013, Pokorna and Zabranska 2015 |
2 | Facultative photolithotroph (PSB) Energy—Light C source- CO2 or organic compounds | Allochromatium, Thioalkalicoccus Thioradococcus Thiococcus Thiocysts Thiospririllum Ectothiorhodospira Halorhodospira Thiorhodospira | |
3 | Chemolithotrophs C source- CO2 Energy—reduced sulfur and nitrogen/iron compounds | Thiobacillus thioparus Thiobacillus novellas Thiobacillus termophilic Thiobacillus denitrificans Acidithiobacillus ferrooxidans Acidithiobacillus thiooxidans Acidithiobacillus albertensis Thiomicrospira denitrificans Thiosphaera pantotropha Acidianus brierleyi Thermothrix azorensis | |
4 | Facultative chemolithotrophs Energy—reduced sulfur compounds or organic compounds C source- CO2/organic compounds | Sulfolobus acidocaldarius Sulfolobus metallics Thermothrix thiopara Pseudomonas denitrificans Paracoccus denitrificans | |
5 | Chemoheterotrophs (mixotrophs) Energy—reduced sulfur compounds C source- organic compounds | Beggiatoa alba Thiobacillus intermedius Paracoccus versutus Thiobacillus organoparus Pseudomonas spp. | |
6 | Chemoorganotrophs Energy—organic compounds C source- organic compounds | Thiobacterium spp. Thiomonas permetabolis Pseudomonas acidovorans |
1 | Photolithotrophs (GSB) Energy—Light C source- CO2 | Chlorobium limicola Chlorobium tepidum Chromatium Chloroherpeton, Prosthecochloris, Pelodictyon, Ancalochloris Ectothiorhodospira spp. Cgloroflexus aurantiacus Oscillatoria sp. Lynbya spp. Microcoleus spp. Phormidium spp. Aphanothece spp. Rhodopseudomonas spp. | Starkey 1935, Kuenen and Beudeker 1982, Tang et al. 2009, Sakurai et al. 2010, Lucheta and Lambais 2012, Simon and Kroneck 2013, Pokorna and Zabranska 2015 |
2 | Facultative photolithotroph (PSB) Energy—Light C source- CO2 or organic compounds | Allochromatium, Thioalkalicoccus Thioradococcus Thiococcus Thiocysts Thiospririllum Ectothiorhodospira Halorhodospira Thiorhodospira | |
3 | Chemolithotrophs C source- CO2 Energy—reduced sulfur and nitrogen/iron compounds | Thiobacillus thioparus Thiobacillus novellas Thiobacillus termophilic Thiobacillus denitrificans Acidithiobacillus ferrooxidans Acidithiobacillus thiooxidans Acidithiobacillus albertensis Thiomicrospira denitrificans Thiosphaera pantotropha Acidianus brierleyi Thermothrix azorensis | |
4 | Facultative chemolithotrophs Energy—reduced sulfur compounds or organic compounds C source- CO2/organic compounds | Sulfolobus acidocaldarius Sulfolobus metallics Thermothrix thiopara Pseudomonas denitrificans Paracoccus denitrificans | |
5 | Chemoheterotrophs (mixotrophs) Energy—reduced sulfur compounds C source- organic compounds | Beggiatoa alba Thiobacillus intermedius Paracoccus versutus Thiobacillus organoparus Pseudomonas spp. | |
6 | Chemoorganotrophs Energy—organic compounds C source- organic compounds | Thiobacterium spp. Thiomonas permetabolis Pseudomonas acidovorans |
1 | Photolithotrophs (GSB) Energy—Light C source- CO2 | Chlorobium limicola Chlorobium tepidum Chromatium Chloroherpeton, Prosthecochloris, Pelodictyon, Ancalochloris Ectothiorhodospira spp. Cgloroflexus aurantiacus Oscillatoria sp. Lynbya spp. Microcoleus spp. Phormidium spp. Aphanothece spp. Rhodopseudomonas spp. | Starkey 1935, Kuenen and Beudeker 1982, Tang et al. 2009, Sakurai et al. 2010, Lucheta and Lambais 2012, Simon and Kroneck 2013, Pokorna and Zabranska 2015 |
2 | Facultative photolithotroph (PSB) Energy—Light C source- CO2 or organic compounds | Allochromatium, Thioalkalicoccus Thioradococcus Thiococcus Thiocysts Thiospririllum Ectothiorhodospira Halorhodospira Thiorhodospira | |
3 | Chemolithotrophs C source- CO2 Energy—reduced sulfur and nitrogen/iron compounds | Thiobacillus thioparus Thiobacillus novellas Thiobacillus termophilic Thiobacillus denitrificans Acidithiobacillus ferrooxidans Acidithiobacillus thiooxidans Acidithiobacillus albertensis Thiomicrospira denitrificans Thiosphaera pantotropha Acidianus brierleyi Thermothrix azorensis | |
4 | Facultative chemolithotrophs Energy—reduced sulfur compounds or organic compounds C source- CO2/organic compounds | Sulfolobus acidocaldarius Sulfolobus metallics Thermothrix thiopara Pseudomonas denitrificans Paracoccus denitrificans | |
5 | Chemoheterotrophs (mixotrophs) Energy—reduced sulfur compounds C source- organic compounds | Beggiatoa alba Thiobacillus intermedius Paracoccus versutus Thiobacillus organoparus Pseudomonas spp. | |
6 | Chemoorganotrophs Energy—organic compounds C source- organic compounds | Thiobacterium spp. Thiomonas permetabolis Pseudomonas acidovorans |
1 | Photolithotrophs (GSB) Energy—Light C source- CO2 | Chlorobium limicola Chlorobium tepidum Chromatium Chloroherpeton, Prosthecochloris, Pelodictyon, Ancalochloris Ectothiorhodospira spp. Cgloroflexus aurantiacus Oscillatoria sp. Lynbya spp. Microcoleus spp. Phormidium spp. Aphanothece spp. Rhodopseudomonas spp. | Starkey 1935, Kuenen and Beudeker 1982, Tang et al. 2009, Sakurai et al. 2010, Lucheta and Lambais 2012, Simon and Kroneck 2013, Pokorna and Zabranska 2015 |
2 | Facultative photolithotroph (PSB) Energy—Light C source- CO2 or organic compounds | Allochromatium, Thioalkalicoccus Thioradococcus Thiococcus Thiocysts Thiospririllum Ectothiorhodospira Halorhodospira Thiorhodospira | |
3 | Chemolithotrophs C source- CO2 Energy—reduced sulfur and nitrogen/iron compounds | Thiobacillus thioparus Thiobacillus novellas Thiobacillus termophilic Thiobacillus denitrificans Acidithiobacillus ferrooxidans Acidithiobacillus thiooxidans Acidithiobacillus albertensis Thiomicrospira denitrificans Thiosphaera pantotropha Acidianus brierleyi Thermothrix azorensis | |
4 | Facultative chemolithotrophs Energy—reduced sulfur compounds or organic compounds C source- CO2/organic compounds | Sulfolobus acidocaldarius Sulfolobus metallics Thermothrix thiopara Pseudomonas denitrificans Paracoccus denitrificans | |
5 | Chemoheterotrophs (mixotrophs) Energy—reduced sulfur compounds C source- organic compounds | Beggiatoa alba Thiobacillus intermedius Paracoccus versutus Thiobacillus organoparus Pseudomonas spp. | |
6 | Chemoorganotrophs Energy—organic compounds C source- organic compounds | Thiobacterium spp. Thiomonas permetabolis Pseudomonas acidovorans |
Phototrophic SOB
Photoautotrophic bacteria have the ability to convert light energy directly into chemical energy in the presence of light. They use adenosine triphosphate (ATP) to convert carbon dioxide to organic compounds with reduced chemical compounds like H2S acting as an electron source. The Van Niel curve represents the rate of sulfide oxidation as a function of the intensity of the applied light energy. This curve depicts the amount of light energy required to oxidize sulfides to elemental sulfur at a given concentration (Equation 1). If the concentration of sulfides increases at a given light intensity, they accumulate in the photoreactor, whereas if it decreases, they are oxidized to sulfates (Equation 2). The photoautotrophic bacterial photosynthesis is called as chemosynthesis as H2S or molecular hydrogen is used as a hydrogen source instead of water, leading to no release of oxygen to atmosphere.
Green sulfur bacteria (GSB) oxidize sulfides to sulfates via S0. In comparison to other phototrophic organisms, GSB can function in much lower light intensity and anaerobic conditions. They do not, however, grow in complete darkness (Tang et al. 2009). Chlorobium, Chloropsuedomonas, Clorobacterium, Chloroherpeton, Prosthecochloris, Pelodictyon, and Ancalochloris are among the genera included in GSB. Among them, C. limicola is the most important because it has an optimal light absorption spectrum in the range of 350–850 nm, with the optimum being 760 nm (Sakurai et al. 2010).
Purple sulfur bacteria (PSB), which include the genera Allochromatium, Chromatium, Thioalkalicoccus, Thiorodococcus, Thiococcus, Thiocystys, and Thiospirillum, store produced sulfur in the form of spherical particles within the cells. Sulfates are released from the cell following oxidation. PSB can also use organic compounds, which is why they are classified as facultative photolithotrophic bacteria. Some species of SOB, such as Ectothiorhodospira, Halorhodospira, and Thiorhodospira, are capable of producing sulfur outside the cell (Tang et al. 2009, Nosalova et al. 2023). The bacteriochlorophyll of PSB has a composition close to chlorophyll-a, and it also contains red and yellow carotenoids (Khasimov et al. 2021).
GSB and PSB are present in an array of environments, including freshwater and marine ecosystems, where they play an important role in sulfur cycling. Phototrophic SOB can be used in bioremediation processes to remove H2S from biogas or other industrial processes, as well as sulfides and thiosulfates from wastewater and other polluted environments. In addition to light and CO2, they require inorganic nutrients (ammonium salts, chlorides, phosphates, sulfates, or some trace elements) for proper growth. However, due to their slow growth rate and high operating costs, the use of phototrophic SOB is still limited, and alternative methods such as using chemolithotrophic SOB or other bioremediation techniques are frequently preferred (Madigan and Jung 2009).
Chemolithotrophic SOB
The Chemolithotrophic group of SOB is called non-photosynthetic, colorless autotrophs, entirely consisting of bacteria. The Chemolithotrophic SOB obtain energy and electrons by oxidizing reduced inorganic sulfur compounds (RISCs), such as H2S, thiosulfates, sulfites, and elemental sulfur, instead of light. In most cases, these metabolites are combined with molecular oxygen in the cells, resulting in the release of energy through an exothermic reaction. In some cases, they can also obtain energy from organic sulfur compounds such as methanethiol, dimethyl sulfide, and dimethyl disulfide (Kazemi et al. 2021). Carbon dioxide is generally used as a C source for the subsequent food synthesis, resulting in the construction of new cell material.
Chemolithotrophic SOB are Gram-negative, rod-shaped, non-spore-forming aerobes or anaerobes, and they can grow at temperatures between 4 and 90°C and a pH range of 1–9. Most of the studied chemolithotrophic bacteria thrive best in mesophilic or thermophilic conditions, such as Thiobacillus, Sulfolobus, and Thermothrix (Tang et al. 2009). Chemolithotrophic SOB can be classified into two morphologically and taxonomically distinct groups: small, short rods of the genus Thiobacillus (such as T. thioparus, T. denitrificans, and T. thiooxidans) and long, filamentous bacteria of the genera Beggiatoa and Thiothrix. The long, filamentous bacteria oxidize H2S–S0 (Anandham et al. 2011). In an ample supply of H2S, the oxidized sulfur is stored as black droplets within the cells, and S0 is further oxidized to soluble sulfates, which pass through the cell walls. Based on the source of C and energy, chemolithotrophic SOB are classified into three groups (Tang et al. 2009): obligate chemolithotrophic (Thiobacillus, Thiomicrospira), facultative chemolithotrophic (such as Paracoccus denitrificans, many species of Thiobacillus, Thermotrix, and Sulfolobus), and chemolithoheterotrophic or mixotrophic SOB like Beggiatoa. Mixotrophic SOB can obtain energy from the oxidation of inorganic reduced sulfur compounds but requires organic compounds as C sources due to the lack of enzymes in the Calvin cycle. Other examples of mixotrophic growth are T. novellus, Pseudomonas acidovorans, P. putida, and Thiothrix sp. (Anandham et al. 2011). The pattern of food production in chemoautotrophic SOB is illustrated in Fig. 1.
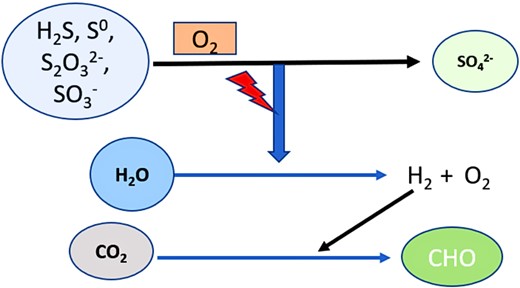
The pattern of food synthesis in chemoautotrophic SOB symbolized above. The chemolithoautotrophic SOB harnesses the energy required for the hydrolysis of water into hydrogen and oxygen molecules by oxidizing the reduced sulfur compounds. Further, the hydrogen ions were utilized in the synthesis of carbohydrates.
The genus Thiobacillus is the most common and well-studied type of chemoautotrophic SOB. It comprises several Gram-negative, rod-shaped, non-endospore, exospore, and cyst-forming bacteria such as T. thioparus, T. denitrificans, T. neopolitanus, T. thioxidans, T. intermidius and T. novellus. T. denitrificans, and T. novellus are neutrophilic and have an optimum pH range of 6–8 (Kelly and Wood 2000). T. thioparus can grow in a broad range of pH levels from 5 to 9, with an optimum of 7.5 at 28°C. Additionally, T. thioparus can also oxidize thiosulfates, apart from sulfides. Thioalkalispira microaerophila is an alkalophilic microorganism with an optimum pH of 10. The temperature requirements of different SOB vary (Anandham et al. 2011). Most of them are mesophilic or thermophilic, but some, such as T. azorensis, have an optimum growth temperature of 76–86°C (Tourna et al. 2014). Most representatives of the genus Thiobacillus spp. are strictly aerobic, such as T. thiooxidans, T. novellus, and T. thioparus. In contrast, T. denitrificans and T. ferroxidans are facultatively anaerobic, and some bacterial genera are tolerant to microaerobic conditions, including T. nivea, T. microaerophila, and Aneurinibacillus aneurinilyticus (Chen et al. 2020). The efficiency of oxidizing reduced sulfur compounds varies among the Thiobacillus species. For instance, T. neapolitanus can only convert 50% of input sulfides to elemental sulfur, while Thiobacillus sp. strain W5 can oxidize sulfides up to 90% (Anandham et al. 2011).
The genera Acidithiobacillus and Halothiobacillus were created from the genus Thiobacillus in the year 2000. Their greater acid tolerance and tight evolutionary ties with other Thiobacillus species led to this reclassification. A Gram-negative sulfur-oxidizing chemolithotrophic bacteria, Acidithiobacillus belongs to the proteobacterial class Acidithiobacillia. The exceptional ability of this species’ members to oxidize different RISCs in order to gain electrons for C dioxide fixation, as well as the ability of some of them to oxidize ferrous iron, is truly astonishing (Wang et al. 2019, Whaley-Martin et al. 2019).
Based on comparisons of the 16S rRNA gene sequence and physiological traits, Acidithiobacillus has been classified into seven species (Kelly and Wood 2000). In addition, Acidithiobacillus can be separated into two groups based on the variations in their energy substrates. Acidithiobacillus thiooxidans, A. caldus, and A. albertensis are among the species that solely oxidize sulfur in the first group. The second group comprises sulfur and ferrous oxidizing species, including A. ferrooxidans, A. ferrivorans, A. ferriphilus, and A. ferridurans (Equations 3 and 4). Acidithiobacillus strains are commonly present in soil, sediments, hot springs, iron-sulfur mineral deposits, and acid mine drainage, all of which are acidic sulfur-containing habitats on land or in the sea. On the other hand, Halothiobacillus are rod-shaped bacteria that obtain energy from the oxidation of reduced forms of sulfur, but they are incapable of oxidizing ferrous ions. They are tolerant to high salt concentrations, and some species, such as H. halophilus, even require high salt concentrations for their growth. The optimum growth temperature for Halothiobacillus is 30–40°C. Other members of this genus include H. neapolitanus (previously known as T. neapolitanus) and H. hydrothermalis (Wang et al. 2019, Whaley-Martin et al. 2019).
Autotrophic denitrifying SOB
Certain species of SOB are capable of using oxidized forms of nitrogen, such as nitrates or nitrites, as electron acceptors and are therefore considered autotrophic and denitrifying. This ability makes them useful in the removal of reduced forms of sulfur from polluted gases and waters. The process of biological oxidation of sulfides in the presence of nitrates or nitrites is based on the activity of T. denitrificans, T. denitrificans, T. versutus, Thiosphaera pantotropha, and P. denitrificans. These bacteria are able to use various inorganic reduced forms of sulfur, such as S2−, S0, S2O32−, S4O62−, and SO32−, as an energy source while simultaneously utilizing nitrate or nitrite as the electron source and reducing it to nitrogen gas. Elemental sulfur, or sulfates, along with nitrogen gas, or nitrites, as an intermediate, are the final products of sulfide oxidation (Sahinkaya and Dursun 2012).
Pseudomonas denitrificans is an autotrophic denitrifying bacterium belonging to the alpha-proteobacteria. It is a Gram-negative, spherical, aerobic bacterium that grows as a chemoheterotroph on mono-C organic compounds such as methanol or methylamine. It oxidizes these compounds to CO2, and as a chemolithotroph, uses reduced forms of sulfur and hydrogen as electron donors during denitrification (Chen et al. 2020). Thiobacillus denitrificans, on the other hand, is a facultative anaerobe that belongs to the beta-proteobacteria class. It can exploit both oxidized forms of nitrogen and Fe2+ in FeS2 (FeS2 and FeS) during the oxidation of sulfides and thiosulfates. In an aerobic environment, T. denitrificans uses thiosulfates and thiocyanates, whereas in an anaerobic environment, it utilizes sulfides and elemental sulfur. The optimal growth conditions for T. denitrificans are pH 6.9 and a temperature of 29.5°C, while the optimum pH for denitrification is 6.85 and a temperature of 32.8°C (Kelly and Wood 2000). In 2007, T. denitrificans was reclassified as Sulfurimonas denitrificans DSM 1251 (Sievert et al. 2008). Table 1 presents the classification of different trophic groups of SOB based on their C and energy sources.
Thiobacillus thioparus is one of the very few autotrophic denitrifiers capable of converting nitrates to nitrites via sulfide oxidation (Tang et al. 2009). Although autotrophic denitrifying bacteria are chemolithotrophic, P. versatus, P. denitrificans, and Beggiatoa sp. can adapt to autotrophic, heterotrophic, and mixotrophic growth. However, results from pure culture studies cannot be directly applied to real-world conditions because mixed cultures of microorganisms are commonly used in practice, where chemolithotrophic denitrifiers may predominate. Autotrophic bacteria use inorganic substances such as CO2 as a source of C, eliminating the need for external organic C sources. Therefore, the technological application of autotrophic denitrification in wastewater treatment has many advantages over conventional methods, such as reduced operating costs, lower production of activated sludge, and less time requirement (Zhou et al. 2021).
Mechanisms of S oxidation by the SOB
Thiosulfate is the most common reduced form of sulfur and can be found in a variety of environments. It is used as an electron source for energy generation in the photosynthetic and respiratory systems of many bacteria. Another reduced form of sulfur used by chemolithotrophic bacteria is tetrathionate, which can be produced as an intermediate sulfur compound in the oxidation of thiosulfate. Three oxidation processes for sulfide, thiosulfate, and elemental sulfur have been proposed over time. The first is the formation of tetrathionate as an intermediate sulfur compound, while the second is the direct oxidation of thiosulfate to sulfate (Rohwerder and Sand 2008). Tetrathionate is produced as an intermediate compound by obligate chemolithotrophic beta and gamma-proteobacteria such as Acidithiobacillus (S4I). Meanwhile, photo- and chemolithotrophic alpha-proteobacteria like Paracoccus use the “Paracoccus sulfur oxidation” or “PSO pathway,” also known as the Kelly–Friederick pathway and regulated by the Sox operon (Kelly and Wood 2000). The “branched thiosulfate oxidation pathway” refers to a third mechanism of thiosulfate oxidation in anaerobic photo or chemolithotrophic bacteria that involves intracellular sulfur depositing (Friedrich et al. 2001).
Sox system
The sulfur (S) oxidation process known as the Sox pathway has received the greatest attention and is the most well-understood pathway. However, there are still some unanswered questions. In the lithoautotrophic P. pantotrophus, a multi-enzyme periplasmic system was found. Several evolutionary groups of phototrophic and chemolithotrophic sulfur-oxidizing prokaryotes are thought to include this complex in a broadly distributed manner. Sulfide, thiosulfate, sulfite, and S0 are all oxidized to sulfate as the end product by a multienzyme complex that is encoded by the soxTRS-VW-XYZABCDEFGH operon. SoxXA genes code for c-type cytochromes, SoxYZ genes for proteins that covalently bind sulfur and proteins that chelate sulfur compounds, SoxB genes for monomeric dimanganese-containing proteins, and Sox (CD)2 genes for sulfur dehydrogenases. Via a cysteinyl residue at the C terminus of the SoxY subunit of the SoxYZ protein, the Sox complex covalently bonds to a molecule of thiosulfate and oxidizes the S atoms by transferring electrons to a c-type cytochrome without creating intermediate molecules (Pyne et al. 2018).
The oxidation of sulfide, thiosulfate, sulfite, and elemental sulfur to sulfate is carried out through the periplasmic multi-enzyme Sox route. This pathway’s core process, thiosulfate oxidation, involves a number of enzymatic stages. A cysteine S-thiosulfonate derivative, designated SoxYZ-S-S-SO3, is created when SoxXA catalyzes the interaction between the sulfane sulfur of thiosulfate and the SoxY-cysteine-sulfhydryl group of the SoxYZ complex. The SoxYZ complex’s terminal sulfone (-SO3) group is then hydrolyzed by SoxB to produce sulfate (SO42). The remaining SoxY-cysteine persulfide (SoxYZ-S-S) is then oxidized by Sox(CD)2 to cysteine-S-sulfate (SoxYZ-S-SO3). Lastly, SoxB hydrolyzes the sulfonate moiety (-SO3) once more, regenerating SoxYZ (Fig. 2).
![Overview diagram of soil sulfur oxidation. Thiocyanate is either oxidized to the level of elemental sulfur via the cyanate pathway [TcDH; TCDH] or degraded to H2S via the carbonyl sulfide pathway [thiocyanate hydrolase to COS, then COS hydrolase to H2S]. By either flavocychrome [FCC] or sulfide quinone reductase [SQR], SO2 can be converted to elemental sulfur. Thiosulfate is covalently attached to SoxYZ by SoxXA, and then SoxB cleaves the sulfane sulfur to produce sulfate, which can then be oxidized by SoxCD into elemental sulfur or released as sulfate. The reverse dissimilatory sulfite reductase system [DSR] converts zero-valent sulfur to sulfite, which is then converted to sulfate either directly [SDH] or indirectly by producing adenylyl phosphosulfate in the presence of adenylyl phosphosulfate Reductase, then to sulfate, which is mediated by Sulfate Adenylyltrasferase. The color gradient represents the oxidation state for each component, fully reduced [-II, Blue] to fully oxidized [+IV, Green].](https://oup.silverchair-cdn.com/oup/backfile/Content_public/Journal/jambio/134/8/10.1093_jambio_lxad161/1/m_lxad161fig2.jpeg?Expires=1747921254&Signature=gIaMUX4uOfM1Q6YfL-MgM-Yl8OeqvfbvR8l-uj7c0h2-UIDiFE-k2wkshkWPfvu2IrJ6j5NKDDVDRRGIyH6qXxCNtqDxSeZh-dh2fsdCKy2D3-KZF3ub2ZGitVd1oqyU674itREFsfUSk22PeMcC-0M8yNGRytVlzBl-xAnxn9D-vUpk~QB9~A~8tX0Qt7XRUoZ3W0ODo8rE7J0ST61aUf6my2vkxAzf0-3j8KJeMxknJw3wx5zK~AN2gLBTXytAQ3rKj1Cl1qhy1SecHwdFECru7-3CeF0pr9Xk5uIPIR0AG8dvyLkwEh-tRZIv8egp77aK1FCM8C4bidRC4fbl3w__&Key-Pair-Id=APKAIE5G5CRDK6RD3PGA)
Overview diagram of soil sulfur oxidation. Thiocyanate is either oxidized to the level of elemental sulfur via the cyanate pathway [TcDH; TCDH] or degraded to H2S via the carbonyl sulfide pathway [thiocyanate hydrolase to COS, then COS hydrolase to H2S]. By either flavocychrome [FCC] or sulfide quinone reductase [SQR], SO2 can be converted to elemental sulfur. Thiosulfate is covalently attached to SoxYZ by SoxXA, and then SoxB cleaves the sulfane sulfur to produce sulfate, which can then be oxidized by SoxCD into elemental sulfur or released as sulfate. The reverse dissimilatory sulfite reductase system [DSR] converts zero-valent sulfur to sulfite, which is then converted to sulfate either directly [SDH] or indirectly by producing adenylyl phosphosulfate in the presence of adenylyl phosphosulfate Reductase, then to sulfate, which is mediated by Sulfate Adenylyltrasferase. The color gradient represents the oxidation state for each component, fully reduced [-II, Blue] to fully oxidized [+IV, Green].
The comprehension of the procedure has improved with the identification of intermediates in the Sox route. It has been suggested that SoxYZ, the real carrier species in the Sox route, conjugates with multiple sulfane atoms to generate SoxYZ-S-thioperoxosulfonate (SoxY(SSSO3)Z') instead of cysteine S-thiosulfonate. Moreover, there are Sox pathways that are deficient in Sox (CD)2, which is lacking in a variety of SOB that depend on the Sox system. The sulfane intermediate’s sulfur atom is probably supplied to other Sox routes or changed into storage forms of sulfur in these abbreviated Sox pathways. Further details on the catalytic mechanism of the Sox system at the atomic level have been revealed by recent studies on the three-dimensional structures of Sox proteins and the discovery of active sites (Grabarczyk and Berks 2017).
S4I pathway
There are many beta and gamma-proteobacteria that possess the thiosulfate oxidation pathway, which produces tetrathionate as an intermediate (S4I) and is particularly prevalent in obligately chemolithotrophic genera like Acidithiobacillus, Thermithiobacillus, Halothiobacillus, and Tetrathiobacter (Dam et al. 2007). The thiosulfate: quinone oxidoreductase (Tqo) encoded by DoxDA, and tetrathionate hydrolase is a component of the S4I pathway. Tetrathionate is produced when TetH hydrolyzes thiosulfate, while TQO oxidizes thiosulfate to create tetrathionate. Tetrathiobacter kashmirensis was subject to a description of another suggested mechanism. In this instance, the membrane is the site of total tetrathionate oxidation, whereas a periplasmic oxidation pathway converts thiosulfate to tetrathionate. By transferring electrons from sulfite to oxygen through ubiquinone-cytochrome b, sulfite dehydrogenase oxidizes sulfite in the cytoplasm (Dam et al. 2007).
Many enzymes, including sulfur dioxygenase, are involved in the sulfur oxidation pathways. This enzyme has previously been proposed as the initial enzyme in charge of the SDO-dependent sulfur oxidation system’s extracellular elemental sulfur oxidation (Rohwerder and Sand 2007). In the intracellular space of Acidithiobacillus and other SOB and archaea, the heterodisulfide reductase (Hdr)-like system may also serve as an elemental sulfur oxidation enzyme (Koch and Dahl 2018). The membrane-bound protein SQR has also been shown to oxidize sulfide to zero-valent sulfur while producing electrons that are supplied into the membrane quinone pool. The APS system, which makes use of the enzymes APS reductase (AprBA) and ATP sulfurylase, is another system that contributes to sulfite oxidation (SAT). Moreover, these enzymes contribute to the dissimilatory sulfate reduction process in sulfate-reducing bacteria (Griesbeck et al. 2000).
Methods for determination of S oxidation in soil
This is because total sulfur includes both available and unavailable forms of sulfur, and only the available forms are important for plant nutrition. The available sulfur is the fraction of total sulfur that can be readily taken up by plants in the form of sulfate ions (SO42−). In general, the available sulfur content of soil is much lower than the total sulfur content, and the availability of sulfur for plant uptake is often limited by the slow rate of mineralization of organic sulfur compounds. Therefore, it is important to determine the available sulfur content of the soil to determine the sulfur fertilizer requirement for crops.
Most studies on S0 oxidation involve incubating soil samples with S0 under laboratory conditions for several weeks and then analyzing the ionic S species and/or changes in the population of SOB. The rate of S0 oxidation in soil can be determined directly by quantifying the remaining S0 after the incubation period (Watkinson et al. 1987), or indirectly by measuring the sulfate produced as the final product of S0 oxidation (Harris et al. 2003). Other indirect methods of measurement include pH determination, which tends to decrease with the formation of H2SO4; respirometry for aerobic SOB; and 14CO2 uptake (Boyd et al. 2009, Mora et al. 2016). The remaining S° can be quantified through high-pressure liquid chromatography (HPLC) analysis of chloroform soil extracts using a reverse-phase column and an isocratic gradient of methanol and chloroform (Nair et al. 2014).
Alternative methods have been proposed for quantifying S0 in soil samples, such as a gas chromatography method (Yan 2002). Additionally, different sulfur fractions can be extracted with chloroform and analyzed by techniques like inductively coupled plasma atomic emission spectroscopy or sulfur K-edge X-ray absorption spectroscopy. Soluble SO42− can be determined by the turbidimetric method, which involves extraction with monocalcium phosphate or ammonium acetate followed by precipitation with barium chloride solution (10%) and measurement of absorbance at 420 nm (Godoi et al. 2019). Another approach to quantify sulfate in soil is ion exchange chromatography (Chai et al. 2016).
For assessing S0 oxidation in soils, the determination of remaining S0 is a more accurate method than the determination of SO42− due to mineralization of organic S and/or immobilization of SO42−during long incubation times, which can reduce the efficacy of the technique. Germida and Janzen (1993) proposed shortening the incubation period before sulfate determination to six days to reduce this effect. The use of toxic solvents such as toluene, chloroform, and acetone for S0 extraction from soil samples is a major drawback of determining the remaining S0 (Chai et al. 2016). Furthermore, soil moisture and sample drying temperature have a significant impact on chloroform extraction of S0, and soil particle aggregates cannot be properly dispersed, interfering with solubilization (Watkinson et al. 1987).
Factors influencing the oxidation S0 in agro-ecosystem
The oxidation of reduced S compounds in soil is influenced by a range of biotic and abiotic factors. Abiotic factors that play a significant role in S0 oxidation include temperature, moisture, aeration, pH, the presence of organic matter, different particle sizes, soil dispersion, and fertilizer formulation. The diversity of SOB is a major biotic factor that affects S0 oxidation in soil.
Soil temperature
The rate of S mineralization in soil is significantly affected by soil temperature. Extremely low temperatures below 10°C and high temperatures above 40°C result in a very low S mineralization rate, while the optimum temperature for S mineralization is around 30°C. Several studies have reported an increase in the rate of S oxidation with an increase in soil incubation temperature. For instance, after 57 days of incubation, the percentage of S0 oxidation was 8% at 5°C, 22% at 15°C, and 47% at 30°C. The Ratkowsky equation describes the connection between microbial proliferation and temperature (Franzmann et al. 2005) (Equation 5).
Here, T is the temperature, Time is typically the generation time or the time required to reach a particular condition (e.g. the time required for a culture to increase its optical density to 0.6 absorbance units), TMIN is the theoretically extrapolated minimum temperature for growth, Tmax is the theoretically extrapolated maximal temperature for growth, and b and c are fitting parameters.
Soil pH
The bio-oxidation of sulfur in the soil is significantly influenced by the pH of the soil. The rate of sulfur oxidation rises in alkaline or slightly acidic soils with a pH of 6.5–6.0, but it falls when soil pH falls. According to studies, elemental S in soil with a pH of 6.7 was oxidized to around 40% in the second week and to 90% in the fourteenth week (Rousk et al. 2010). The impact of pH on the makeup and activity of the microbial community is thought to be connected to its effect on the rate of S0 oxidation. When pH rises in arable soils, bacterial diversity and abundance increase. Agricultural soils frequently include SOB, such as neutral Thiobacillus spp. and heterotrophs, which favor neutral and alkaline conditions. In terms of soil characteristics, pH and EC have a negative correlation with plant-available S in the top 30 cm of the soil profile and a positive correlation with organic C in the bottom 30–60 cm (Rousk et al. 2010).
Soil moisture
In addition to temperature and pH, moisture and aeration are also important abiotic factors affecting S0 oxidation in soils. The relationship between S oxidation and soil moisture is parabolic. Minimal oxidation occurs at low moisture content, but as moisture availability increases up to a peak (around 60% of field capacity), the rate of oxidation rises. However, it then decreases as moisture content exceeds optimal levels. The near-optimum moisture level for maximum S0 oxidation is close to the soil field capacity, as it allows for good soil aeration. The concentration of SO4-2 ions in the soil solution ranged from 32% to 53% and 72% to 106% at moisture levels of 40% and 90% of the field capacity, respectively. Soil moisture varies depending on soil type, texture, and degree of aeration. Soil aeration is also vital because SOB require oxygen for their growth and metabolism. Insufficient aeration can limit the growth of SOB and reduce the rate of S0 oxidation (Ghaderi et al. 2019, Pourbabaee et al. 2020).
Land cultivation and agronomic practices
S oxidation in the soil is influenced in part by land cultivation and agronomic techniques. Following the initial cultivation, the S concentration in the soil might dramatically fall. Nevertheless, depending on climatic circumstances, management approaches, and local soil features, balance can be established over time. This connection implies that S is less mineralizable in soil than C and N (Solberg et al. 2003). Conventional tillage can result in a rapid loss of soil organic matter, lowering S and the soil’s long-term fertility. Soils that get frequent S0 treatments have a greater Thiobacillus spp. count, suggesting a larger S0 oxidation potential than soils that have never received S0, implying a priming effect of Thiobacillus spp. oxidation. The rate of S oxidation is also affected by the kind of soil. The rate of sulfur oxidation is often higher in sandy loam, followed by clay soil. Sulfur oxidation activity is nevertheless considerably reduced in silty clay soil (Solberg et al. 2003, Yang et al. 2007).
Crop and cropping system
As proven over fallow land, the crop and cropping system have an important role in modulating sulfur transformations in the soil, owing mostly to increased microbial biomass in the rhizosphere. Various plants have distinct sulfur conversions, but there has been little effort to quantify them inside the rhizosphere independently from the bulk soil until recently. Nevertheless, the rhizosphere microbial community composition differs greatly between plant species due to differences in the volume and composition of root exudates (Grayston and Germida 1990, Zhalnina et al. 2018). Adopting cropping strategies that result in reduced nutrient losses and increased soil organic matter would thus be useful in boosting soil S supply in a mineralization-friendly year while minimizing the amount of fertilizer S applied (Schoenau and Malhi 2008, Iannucci et al. 2021).
Soil microbes
Soil microorganisms are critical in converting reduced sulfur compounds in the soil to an absorbable form (SO42−). Despite the fact that various microorganisms are engaged in S-oxidation, bacteria, notably Thiobacillus and related taxa, have a significant impact in sulfur oxidation (Rana et al. 2020). The size and activity of the microbial biomass influence the rate of elemental S oxidation in agricultural soils. S oxidation in soil is largely driven by heterotrophic sulfur-oxidizing groups, followed by autotrophic microorganisms. The bulk of organic S in soil resides as the sulfate ester portion (up to 60%), which may be readily mineralized by the activity of enzymes generated by a broad variety of heterotrophic bacteria, including Pseudomonas and Thiobacillus sp. (Lawrence and Germida 1988).
Certain microbial species or genera in a crop rhizosphere play a larger role in sulfur cycling than others in the crop itself. Yet, because most soil microorganisms cannot be cultivated in artificial medium, this functional specialization has received little attention. Because of this constraint, cultivation-based approaches produce a skewed picture of the soil’s microbial ecology. A focused functional diversity investigation is required to better understand the role of soil microorganisms in sulfur cycling. This may be accomplished using cultivation-independent approaches such as stable isotope probing and soil genetic profiling (Radajewski et al. 2000; Dumont and Hernández García 2019).
Previous research revealed that the population of SOB, notably Thiobacillus and related taxa, is insignificant in soil. Recent research has revealed, however, that the population of SOB may grow with the addition of elemental sulfur and can carry out sulfur oxidation for up to two weeks. On calcareous soils, inoculating soil with Thiobacillus at a rate of 104 cells per gram of soil, combined with varying quantities of elemental sulfur, has been shown to boost yields, nutrient availability, and nutrient uptake by plants. Promoting SOB development in soil with elemental sulfur and Thiobacillus inoculation might thus be a promising technique for boosting agricultural production and nutrient cycling in soils.
Particle size
In agro-ecosystems, the rate of S0 oxidation is inversely related to particle size (Lee et al. 1988). Direct contact between A. thiooxidans and S0 particles is required for efficient oxidation (McCaskill and Blair 1987). The particle size of applied sulfur particles should be between 80 and 1000 mesh or smaller to ensure increased efficacy of sulfur fertilizer (S0). Unfortunately, because S0 is combustible, it is difficult to use extremely small particles of S0 in the field. The form of the particle influences the overall surface area, with larger spherical shapes having lower mass/area ratios. Many mathematical models have been presented over time to calculate the oxidation rate depending on the size and shape of S0 particles, but the optimum standard model is still being debated. Furthermore, an inadequate dispersion of S0 particles in soil significantly reduces the oxidation rate (Degryse et al. 2016, Zhao et al. 2016).
Soil organic matter or organic C
Organic C and soil organic matter show a considerable positive connection with soil sulfur. Organic sulfur is a diverse mixture of soil organisms and partially degraded plant, animal, and microbial leftovers that contributes up to 95% of total soil sulfur in most agricultural soils (Srinivasarao et al. 2004). Application of organic wastes with a broad C:N ratio (>400:1) immobilizes the available sulfur during breakdown. In contrast, the breakdown of sulfur-rich wastes with a narrow C:S ratio (<200:1) is linked with quick net mineralization and increasing sulfur concentration. Since freshly generated organic sulfur mineralizes faster than old organic sulfur, the latter being released more slowly. Organic debris such as wheat straw, broom grass cutting, farm yard manure, poultry litter, and peat moss has been shown to improve soil sulfur concentration (Lee et al. 2021, Malik et al. 2021).
Applications of SOB in agriculture
Sulfur deficiency can have a detrimental influence on plant development and output. Sulfur reactions have been documented in 23 key crops in 40 tropical countries (Fuentes-Lara et al. 2019). To solve this issue, sulfur fertilizers, generally in the form of reduced sulfur, are widely given to soils (S0). Thiobacillus and related taxa are recognized to play an important role in crop production by enabling S-oxidation, P-solubilization, and the solubilization of other important nutrients such as Zn, Fe, and others. They are utilized in the reclamation of alkaline soil, making unavailable nutrients available for plant growth (Rana et al. 2020). Although SOB occur naturally in soils, the most essential species are not typically present in agricultural soils; hence, inoculation is generally utilized to get a desirable result.
SOB and S nutrition in crop plants
The rate of oxidation of supplied elemental S is typically 40%–51%, making it available for plant absorption. Nevertheless, the application of SOB can boost natural S oxidation, resulting in the generation of sulfates that are easily accessible to plants at various phases of growth. This can lead to improved plant yield (Kulczycki 2021), as well as an increase in secondary metabolite content, such as increased allylthiocyanate and allylisothiocyanate content in mustard (Brassica juncea) oil and roots, respectively. Importantly, garlic plants in Korea exhibited a substantial positive relationship between allylsulfide concentration and soil-accessible sulfur (Avato et al. 2000). Inoculation of Thiobacillus sp. and elemental sulfur enhanced the plant growth and yield of several agricultural plants (Vidyalakshmi et al. 2009). The inoculation of SOB with Rhizobium resulted in synergistic interactions promoting the yield and oil content of groundnuts, in S-deficit soils. There was a significant increase in plant height (62%), yield (221%), and N uptake (629%) in onions when inoculated with SOB (T. thiooxidans) and a nitrogen-fixing bacterium (Azotobacter chroococcum) (Awad et al. 2011).
SOB and P nutrition in crop plants
After nitrogen, phosphorus is the second most essential plant nutrient, having a vital role in plant development, yield, and produce quality (Sharpley 2000). Unfortunately, the bulk of the phosphorus in the soil is in a form that agricultural plants cannot use, and phosphatic fertilizers are frequently fixed in the soil, with around 90% of the administered phosphorus being inaccessible to plants. Phosphorus deficits can result in poor growth and root development, reducing yield and quality (Vidyalakshmi et al. 2009, Malhotra et al. 2018). The two basic options for enhancing phosphorus nutrition through microorganisms are the use of phosphorus-solubilizing bacteria and phosphorus mobilizers such as arbuscular mycorrhizal fungi. Another popular method for improving phosphorus nutrition in an agro-ecosystem is to utilize SOB in combination with a reduced form of sulfur (generally sulfur trioxide) with or without a complex phosphorus source like rock phosphate (RP).
The amount of water-soluble phosphate in the soil was increased after inoculating the soil with the SOB T. thiooxidans, RP, elemental sulfur, and vermicompost. In a different study, the application of sulfur, RP, and Thiobacillus sp. greatly improved soil extractable phosphorus and phosphorus uptake by plants (Jazaeri et al. 2016). Nutrient absorption, yield, and oil content in canola have all been demonstrated to increase with the use of Thiobacillus sp. and a phosphorus-solubilizing bacteria, Bacillus sp. This investigation was further supported by research by Bashir et al. (2020) in maize and Masoodi and Hakimi (2017) in pumpkin. Thiobacillus thiooxidans strain has been demonstrated to improve soil fertility due to its capacity to oxidize sulfur. This biological oxidation alters soil structure by decreasing pH and salt content and increasing positive microbial activity. Soil fertility changes allow plants to absorb more nutrients, resulting in increased growth, yield, and quality (Vidyalakshmi et al. 2009, Liu et al. 2023, Nadeem et al. 2023).
Bio-acidulation of RP
In recent years, there has been a significant interest in the practical application of RP as a fertilizer due to its being the cheapest source of P fertilizer. However, the majority of the world’s RP deposits are low-reactive and thus unsuitable for direct application without pre-treatment. Microbiological approaches have been proposed to improve the agronomic value of RP. It has been demonstrated that inoculating RP with phosphate-solubilizing bacterial species such as Dyella ginsengisoli, Pandoraea pnomenusa, Halothiobacillus sp., Pandoraea sp., Bacillus megatherium, A. ferrooxidans, and others significantly improves RP solubilization (Anandham et al. 2007a).
The solubilization of RP has always been accompanied by a decrease in the pH of the incubation mixture. In calcareous alkaline soils, biochemical sulfur oxidation produces sulfuric acid (H2SO4), which lowers soil pH and dissolves calcium carbonate (CaCO3). This, in turn, increases the available P in soil by increasing the solubility of phosphate rocks, providing phosphorus to the plant growth (Stamford et al. 2007). Acidithiobacillus ferrooxidans has a high potential for neutralizing alkaline pH and accelerating primary mineral weathering in iron ore tailings. Several studies have found that SOB improves sulfur availability, RP solubilization, and plant growth promotion in canola, groundnut, and yam bean (Stamford et al. 2007). Under low-nutrient soils, an Acidithiobacillus sp., which oxidizes sulfur and iron, solubilized ∼34.5% of inorganic phosphorus from RP and increased sugarcane yield. This finding was further confirmed by other researchers (Ashraf et al. 2018, Joshi et al. 2021). As a result, SOB can be used as a key component in P-solubilization and the production of soluble phosphate from insoluble sources (Joshi et al. 2021).
SOB in zinc and iron nutrition of crop plants
The yield of groundnut was enhanced, along with increased zinc and iron content in grains, by the application of Rhizobium and Thiobacillus sp. together with the spray application of Zn and Fe micronutrients (Anandham et al. 2007). In arid regions, the application of Thiobacillus and sulfur reduced the soil pH, resulting in increased availability of NPK and other nutrients for crop growth and yield (Tabak et al. 2020). The yield and grain quality of maize were improved by inoculating with T. thiooxidans along with micronutrient spray application of iron and zinc (Anandham et al. 2011). Increased Fe and Zn content was reported in wheat plants after inoculation with Thiobacillus sp. and application of ground (crumb) rubber. In soil, boron and molybdenum interact negatively with sulfur, while nitrogen, phosphorus, potassium, magnesium, and zinc interact positively (Salvagiotti et al. 2009, Tabak et al. 2020).
SOB and biocontrol of pests and diseases
SOB have shown potential in controlling plant pathogens and pests through various mechanisms. The oxidation of sulfur compounds by these bacteria has been observed to balance the sodium content in the soil, which in turn controls certain plant pathogens (Kertesz and Mirleau 2004). For instance, potato scab and rot of sweet potato caused by Streptomyces scabies and S. ipomea, respectively, can be controlled by inoculating sulfur-amended soil with Thiobacillus under acidic conditions. This is due to the increased availability of both sulfur and phosphorus. Furthermore, SOB has been found to inhibit the growth of root pathogens like Rhizoctonia solani and Leptosphaeria maculans in canola. In addition, sulfur content in plants has been reported to induce resistance against various plant pathogens such as Rhizoctonia and Verticillium dahliae (Avato et al. 2000). Therefore, the application of SOB can be a promising strategy for biocontrol of plant pathogens and pests (Kertesz and Mirleau 2004, Gao and Fan 2023).
Certain secondary metabolites containing sulfur, such as glucosinolates, isothiocyanates, thiocyanate, and nitriles, have been shown to act as insect repellents and disease control agents. Isothiocyanates are compounds that are formed enzymatically from sinigrin following tissue damage. These compounds have antibacterial, antifungal, and antinematicidal properties (Tarar and Peng 2022). For example, Brassica species have been observed to inhibit the mildew pathogen Peronospora parasitica and the root-knot nematode (Meloydogine spp.) due to their high isothiocyanate content (Kertesz and Mirleau 2004). In cultivated B. oleracea, there is a correlation between isothiocyanate content and disease resistance. Allylisothiocyanate is not only a repellent, but it can also be toxic to insects. For instance, when larvae of the black swallowtail butterfly fed on 0.1% sinigrin-treated celery leaves, the mortality rate was 100%. This demonstrates that sinigrin in cabbage plants serves a clear defensive function against insect pests that do not typically feed on Brassicaceae (Tarar and Peng 2022).
SOB and soil reclamation
SOB can play an important role in the reclamation of soils with alkaline, sodic, and calcareous properties, as well as those contaminated with heavy metals. Through biochemical sulfur oxidation, SOB can produce H2SO4, which reduces soil pH and dissolves CaCO3 in calcareous soils. Studies have shown that the application of Acidithiobacillus sp. with sulfur and gypsum to saline and sodic soils improves crop stand compared to the application of sulfur and gypsum alone (Stamford et al. 2015). Additionally, A. ferrooxidans is involved in the biosynthesis of schwertmannite, a soil amendment that can immobilize arsenic in contaminated soil (Chai et al. 2016). Other research has demonstrated that the application of elemental sulfur and SOB-treated peat moss can more effectively reduce soil pH compared to treating the soil with peat moss alone (Yi et al. 2021).
The application of Thiobacillus sp. and sulfur has been shown to improve plant growth in calcareous and saline soils by increasing nutrient availability (Yi et al. 2021). Additionally, SOB have been used as biosensors to detect metal ion contamination in soil and water. This is accomplished through the measurement of electrical conductivity (EC) and pH, with SOB activity being inversely proportional to the presence of contaminants. Thiobacillus spp., for example, produce high levels of H2SO4 in the absence of toxic chemicals and under aerobic conditions. However, the presence of toxic chemicals in the medium reduces H2SO4 production significantly (Amin and Mihoub 2021, Yi et al. 2021).
SOB and plant growth-promoting activity
SOB has been found to have plant growth-promoting activity when applied along with elemental sulfur. This has been observed in many crops, including garlic, pumpkin, groundnut, leucena, cowpea, yam bean, and canola. Co-inoculation of SOB with other plant growth-promoting rhizobacteria (PGPR) has also shown a positive effect on crop performance under both pot and field conditions. For instance, the co-inoculation of SOB and Proteus mirabilis enhanced seed germination and the vegetative and reproductive parameters of fennel. Inoculation of SOB like A. thiooxidans and T. thioparus with other soil microorganisms such as Scolecobasidium constrictum, Myrothecium sp., and Aspergillus terreus increased wheat yield by 5%–40% compared to the uninoculated control. The application of SOB has also been shown to enhance stress tolerance against salinity, drought, pests, and diseases by either directly producing growth-promoting substances like IAA, gibberellins, salicylic acid, ACC-deaminase, and siderophores or indirectly by enhanced production of sulfur-containing compounds (Anandham et al. 2007, Joshi et al. 2021).
Conclusion
Sulfur deficiency in soil is becoming more common worldwide, which can lead to reduced crop productivity and yield losses. In India, nearly 46% of soils are deficient in sulfur, resulting in 20%–40% lower crop yields. Similar reports have also been come from various parts of the world. While sulfur is present in the majority of soils in organic forms, it is not readily available for plant uptake. Utilizing S0 is a cost-effective alternative for quickly replenishing sulfur levels, but it must be oxidized to sulfate before plants can take it up. The efficiency of S0 oxidation in soil is influenced by soil and environmental factors, as well as the size of sulfur particles and the diversity of the soil microbiota. Biotechnological applications of sulfur-oxidizing microorganisms offer an opportunity to develop new biofertilizers and increase S oxidation rates in soil. Although sulfur together with SOB has shown beneficial effects in crop growth and production, studies are limited, and the survivability of SOB in different formulations has not been addressed. SOB also possesses multiple plant growth-promoting characteristics, including IAA production, siderophore production, antimicrobial activity, and ACC deaminase activity, which can be harvested as biofertilizer traits. Further studies are needed to understand and study the various biochemical pathways for sulfur oxidation in different soils in various agroclimatic zones.
Future directions in agriculture and other branches of sciences
The application of SOB is not limited to agriculture; it has a wide range of applications in various other fields. In environmental science, they play a crucial role in detoxifying H2S, soil bioremediation, and waste water treatment. Moreover, in the present-day interest in nanotechnology, SOB is used in the synthesis of nanosized sulfide particles with better optical, electronic, and mechanical properties than other metal nanoparticles. Additionally, SOB plays a significant role in enhancing the operational duration of bio-electrochemical fuel cells, such as sediment microbial fuel cells (SMFC), by limiting phosphorus release from the sediment, which aids in the production of electrical current. SOB can be found in extreme environmental conditions, such as high saline areas (halophytes) and low pH soils (acidophilic), which provide a vast genetic diversity to address many existing problems in agriculture and other fields of science using biotechnological tools. In the gas industry, the removal of H2S from natural gas, biogas, synthesis gas, etc. requires high investment and maintenance. However, biological desulfurization (BDS) offers several remarkably environmental advantages as it operates at ambient temperature and atmospheric pressure with no requirement of toxic chemicals. Further, this technology produces biologically re-usable sulfur (S0) as a by-product, which attracted the attention of many industries. Nevertheless, the low biomass of SOB, the acidification of the process solution, and the selectivity of bio-S0 limit its industrial application. Therefore, more efforts should be made to improve the BDS process for its industrial application through different research perspectives. In the energy sector, scientists are using cellulose nanofiber from SOB to improve lithium-sulfur batteries (LSBs). In metallurgy, the bioleaching of various metals, such as Cu, Zn, Fe, etc., using SOB is cost-effective. Currently, CRISPR-Cas is one of the commonly used techniques for genome editing. This system can be used to develop genetically modified SOB with increased thermostability, solvent tolerance, and wider substrate specificity, which help in the better utilization of SOB in agriculture and various other fields.
Acknowledgement
This work would not have been possible without the financial support from the SABIC Research and Technology Pvt. Ltd, Bengaluru, India. We are also immensely grateful to Dr. Haldar S., Central Salt & Marine Chemicals Research Institute, G.B. Marg, Bhavnagar, India for the valuable comments and suggestions for the preparation of this manuscript.
Conflict of interest
All the authors have no conflict of interest.
Funding
SABIC Research and Technology Pvt. Ltd., Bengaluru, INDIA.
Author contributions
Praveen Ranadev (Data curation, Formal analysis, Investigation, Methodology, Software, Writing – original draft), Ashwin Revanna (Methodology, Software, Supervision, Writing – review & editing), Davis Joseph Bagyaraj (Conceptualization, Funding acquisition, Project administration, Supervision, Validation, Writing – review & editing), and Ambika H. Shinde (Resources)
Data Availability
There are no new data associated with this article.