-
PDF
- Split View
-
Views
-
Cite
Cite
Ujwal Dahal, Karan Paul, Shelly Gupta, The multifaceted genus Acinetobacter: from infection to bioremediation, Journal of Applied Microbiology, Volume 134, Issue 8, August 2023, lxad145, https://doi.org/10.1093/jambio/lxad145
- Share Icon Share
Abstract
Acinetobacter is a vast bacterial genus comprising of numerous species with variable characteristics. The enigma associated with clinical strains that have been implicated in many nosocomial outbreaks has prompted the need for continuous research on pathogens like Acinetobacter baumannii and members of the ACB complex. However, numerous species of Acinetobacter genus possess diverse metabolic capabilities and have the potential for a plethora of industrial and environment-based applications. Therefore, a comprehensive review on the entire genus, including many under-represented topics, would contribute extensive information to the scientific community indulged in Acinetobacter research. The current review is a unique compilation that attempts to provide the latest update on the genus covering its clinical as well as ecological aspects. Moreover, it is the first study of its kind that focuses on the entire genus and elaborates on the phylogenetic relationships, pathogenesis, and virulence mechanisms, followed by emerging biotechnological applications with future directions.
Abbreviations
- ACB
Acinetobacter calcoaceticus baumannii complex
- AHL
Acyl homoserine lactone
- CRAB
Carbapenem-resistant Acinetobacter baumannii
- DNA
Deoxyribonucleic acid
- GA
Gibberellic acid
- ICU
Intensive care unit
- LPSN
List of prokaryotic names with standing in nomenclature
- MATE
Multidrug and toxic compound extrusion
- MBLs
Metallo-β-lactamase
- MDR
Multi-drug resistance
- MFS
Major facilitator superfamily
- MICs
Minimum inhibitory concentrations
- MLST
Multilocus sequence typing
- NDM
New Delhi metallo-β-lactamase
- OMPs
Outer membrane proteins
- QS
Quorum sensing
- RND
Resistance-nodulation-division
- RNA
Ribonucleic acid
- SMR
Small multidrug resistance
- UTI
Urinary tract infection
Introduction
The genus Acinetobacter is a group of diverse organisms. It comprises of some of the most dreaded infection-causing species implicated in nosocomial as well as community-acquired infections and is becoming increasingly notorious for causing opportunistic infections that may result in serious illness and even death (Almasaudi , 2018). Acinetobacter species have been linked to a variety of disease conditions in hospitals, especially in severely ill patients with debilitated host defense. Such species particularly infect patients using respiratory treatment equipment or catheters. Septicemia, wound sepsis, urinary tract infection (UTI), pneumonia, endocarditis, etc. are some of the critical diseases caused by A. baumannii, which is the most frequently encountered species in hospitals due to its ability to withstand harsh environments. Nosocomial infections are reportedly caused by species like A. calcoaceticus, A. nosocomialis, A. lwoffii, A. junii, A. ursingii, A. bereziniae, and A. serfertii (Rebic et al. 2018). Presence of antibiotic-resistance genes is a common feature of these species, making them naturally resistant to a wide variety of antibiotics. Previously, non-baumannii species were considered less as pathogens due to their limited virulence. In 1970s, they were identified as major nosocomial pathogens with great sensitivity to drugs such as ampicillin, chloramphenicol, and gentamycin (Wong et al. 2017, Kittinger et al. 2017, Baraka et al. 2020).
The genus Acinetobacter harbors many environmentally important species as well, which have a wide range of metabolic capabilities, such as routes for the breakdown of contaminants like hydrocarbons, amino acid derivatives, and crude oil employing them as principal source of nutrition (Xin et al. 2014, Rebic et al. 2018). The majority of the research findings have been acquired utilizing A. baylyi ADP1, A. baumannii, and A. calcoaceticus. Their metabolic pathways and regulatory mechanisms have attracted substantial attention. For example, A. calcoaceticus can degrade up to 90% of aliphatic hydrocarbons in diesel (Ho et al. 2020). Similarly, A. radioresistens APH1, a novel phenol degrader with one of the greatest phenol degrading efficiencies has been identified and used in soil bioremediation (Liu et al. 2020). They have also been implicated in removal of pharmaceutical wastes from the environment (Wang et al. 2018). The use of Acinetobacter species is not limited to biodegradation and bioremediation, they are being used as prospective bioreporters (Li et al. 2021), manufacturers of lipase (Fatima et al. 2021, Patel et al. 2021), biosurfactants (Oanh et al. 2020), and producers of biopolymeric substances (Ranganadha et al. 2020), biodiesel (Zhang et al. 2021, Tan et al. 2022), medicines, and cosmetic and other significant practical uses (Li et al. 2017, Arvay et al. 2021). Therefore, the environmental species also deserve special attention for the benefit of life on land and life below water.
The current review reveals the complexity of Acinetobacter infections, providing crucial new information on their aetiology and their biotechnological potential. We have opened the door for revolutionary improvements in therapy as well as plant-based applications and bioremediation by elucidating the intricate relationship between Acinetobacter, its virulence potential, and the environment. Our work highlights the value of a multidisciplinary approach, emphasizing the need for cooperation among scientists to lessen the burden of Acinetobacter globally and to use it for bioremediation and other biotechnological applications.
Phylogeny
Acinetobacter is a complex group of saprobic bacteria that are Gram-negative coccobacilli with a GC content ranging from 39% to 47%. Members of the genus are non-fermenters, aerobic, catalase-positive, oxidase-negative, and motile in nature. Acinetobacter species can be found in a variety of environments, including water, inside human or animal hosts, different types of plants, and soil (Adewoyin and Okoh 2018, Dandachi et al. 2019). Morphological traits differ according to the growth phase, e.g. appearance of rod-shaped structure is observed during the log phase, but at later stages, coccobacillus structure is displayed. The genus Acinetobacter comprises of 108 closely related species, but only 77 species have discretely published names according to the list of prokaryotic names with standing in nomenclature (LPSN; Cayo et al. 2016, Baraka et al. 2020).
The genus Acinetobacter has a long history of classification. In the past, the Gram-negative non-fermenters, presently known as Acinetobacter, were classified under more than a dozen different generic names. The most well-known scientific names of Acinetobacter species in the nineteenth century were Mima polymorpha, Morexella lwofii, M. glucidolytica, and Micrococcus calcoacetius. Initially, Acinetobacter was suggested to be a broad group of Gram-negative, non-motile saprobes, both oxidase-positive and negative with discernable absence of pigment. Beijernick and colleagues described M. calcoacetius in 1911 (Bergogne-Berezin et al. 1996), and it is the oldest reference for Acinetobacter species. Birsou and Prevot proposed the new genus Achromobacter in 1954, following several revisions. With an improved explanation of the Acinetobacter genus, Baumann and colleagues reclassified many genera and species into the genus Acinetobacteria in 1968 (Ayenew et al. 2021). Additional nutritional studies clearly demonstrated the difference between oxidase-negative and positive strains, and therefore, the subcommittee on the taxonomy of Moraxella and Allied bacteria prescribed that the genus Acinetobacter would solely contain oxidase-negative strains in 1971 (Towner 2009). The use of traditional microbiological as well as numerous molecular and biochemical approaches backed up this prescription. Some of the techniques used to identify and categorize Acinetobacter strains include amplified ribosomal DNA restriction analysis, DNA–DNA hybridization, and 16S DNA sequence analysis. For more than 20 years, these strategies have provided the groundwork for including various species within a genus (Adewoyin and Okoh 2018, Enright et al. 1994).
The genus is divided into three ecologically distinct clades by combined metagenomics, comparative genomics, and phylogenomics research, which revealed two significant environmental transitions at deep phylogenetic levels. One of them has quickly turned toward host-association by acquiring genes responsible for interactions between bacteria and eukaryotes (Almeida et al. 2021, Nemec et al. 2021). Of the three clades, Clade I exhibits the most intra-clade ecological divergence. Members of the clade, such as the Acinetobacter calcoaceticus baumannii (ACB) complex, are more prevalent in soil and habitats where people are present. Both A. calcoaceticus and A. pittii are frequently found in soil, with the former being the most prevalent but rarely found in human hosts. Acinetobacter baumannii, on the other hand, is quite prevalent across the human population. The disparity in the distribution of A. baumannii between soil and host-associated settings raises the possibility that this species is quickly adapting to human-associated environments (including humans, human-associated hosts, and house-holds). Acinetobacter haemolyticus, A. parvus, A. junnii, A. modestus, and other members of clade II are frequently discovered in aquatic habitats and infrequently linked with hosts. Clade III members, such as A. lwoffii, A. generi, A. rudis, A. indicus, and others, are more frequently discovered in organic-rich aquatic habitats, such as wastewater samples and marine sediments. Clade I witnessed substantially greater rates of habitat diversification compared to other groups (Garcia et al. 2017). Recently, Almeida and group used 275 high-quality filtered protein sequences to reconstruct the phylogenic tree with more than 57 species of Acinetobacter. The tree corroborates with the monophyly of the genus Acinetobacter and illustrates four main clades on the basis of the strongest support values (Fig. 1). They also showed ACB complex as a sub-clade of clade II of the genus Acinetobacter (Almeida et al. 2021).
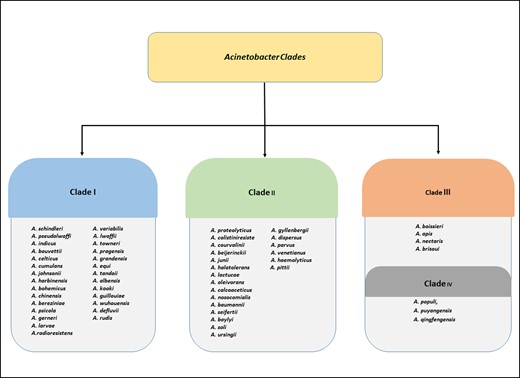
Four main clades according to the phylogenetic tree representing more than 57 species of Acinetobacter as given by Almeida and group in 2021 on the basis of 275 high-quality filtered protein sequences (Almeida et al. 2021).
The term ACB complex was coined because the species that make up the group have nearly identical morphological traits and high genetic relatedness, making precise taxonomic assignment reliant on molecular approaches. Initially, the “complex” consisted of A. calcoaceticus, A. baumannii, and two unclassified strains formerly known as Acinetobacter genomic species 3 and Acinetobacter genomic species 13TU, which were formally reclassified as A. pitti and A. nosocomialis, respectively. Acinetobacter oleivorans, A. lactucae, and A. seifertii have all been identified as members of this complex in recent investigations (Mateo et al. 2019, Almeida et al. 2021). Phylogeny of ACB complex genomes has been verified as monophyletic, and these are frequently responsible for nosocomial infections. Environmental species are mostly from the calcoaceticus lineage and are not linked to nosocomial illnesses. Furthermore, the ACB complex contains both environmental and human pathogenic isolates, which can be misleading for medical treatment because most of the pathogenic isolates are equipped with antibiotic resistance genes (Mateo et al. 2019, Djahanschiri et al. 2022).
Clinical significance
Among several species of Acinetobacter, the Acinetobacter baumannii complex (A. nosocomialis, A. pitti, and A. baumannii) is clinically the most important, followed by A. haemolyticus, A. junni, A. johnsonii, and A. lwoffi. Acinetobacter ursingii, and A. schindleri are also found in clinical infections (Atrouni et al. 2016). Acinetobacter baumannii alone is responsible for over 90% infections in human hosts, while other species together account for the rest. The epidemics caused by Acinetobacter species are due to antibiotic resistance and their ability to persist in a harsh hospital environment, including desiccation and disinfectants (Atrouni et al. 2016, Almasaudi 2018 , Brasiliense et al. 2019). Members of the genus usually cause pneumonia and septicemia, as well as endocarditis, meningitis, and infection in wounds, the urinary tract, and the lungs (Mwanamoonga 2022). Outer membrane proteins (OMPs), cell surface hydrophobicity, toxic slime polysaccharides, and verotoxins have been implicated in the suspected virulence mechanisms employed by members of the genus (Dandachi et al. 2019).
MDR Acinetobacter
MDR Acinetobacter species are those that are resistant to at least one antimicrobial agent among fluoroquinolone, aminoglycoside, penicillin, or cephalosporin. The resistance of Acinetobacter species to a wide range of antibacterial drugs, including first- and second-generation cephalosporins and carbapenems, is well recognized (Ayoub Moubareck and Halat 2020, Ayenew et al. 2021). Most Acinetobacter isolates display multiple mechanisms of drug resistance, such as enzymatic drug degradation, target alteration or protection, and decreased permeability or active efflux of antibiotics. Resistance is acquired either by horizontal transfer of genetic elements containing resistance determinants or through mutations in endogenous genes that result in the inactivation, alteration, or overexpression of cellular functions (Maeusli et al. 2020, Ibrahim et al. 2021).
In clinical settings, the selective pressure of powerful antibiotics has slowly led to a worldwide preponderance of Acinetobacter strains resistant to several antibiotics used to treat infection, including impenim, sulbactam, ampicillin, second generation cephalosporins, quinolones, colistin, aminoglycosides, gentamicin, and minocycline. Atrouni et al. (2016), Breijyeh et al. (2020). Carbapenem-resistant A. baumannii has been designated as a clinically relevant pathogen for antimicrobial research and development by the World Health Organization (WHO). Acinetobacter baumannii is the most prevalent MDR species. Moreover, members of the ACB complex like A. pittii and A. calcoaceticus have lately emerged as MDR nosocomial pathogens (Brasiliense et al. 2019). Recent findings reveal increased carbapenem resistance as well as changes in resistance mechanisms employed by A. pittii. For example, carbapenem-resistant A. pittii (CRAP) has been reported to have disseminated worldwide. The presence of carbapenem-hydrolyzing lactamases, such as NDM1, has been a major issue behind CRAP. Similarly, resistance to erythromycin- and telithromycin-like antibiotics in A. seifertii has been attributed to mutations in the 23S rRNA gene (Furlan et al. 2019). Resistance to β-lactams in A. calcoaceticus is speculated to be an outcome of chromosomally encoded cephalosporinase. Decreased outer membrane permeability is also a major factor contributing to A. calcoaceticus natural resistance toward broad-spectrum antibiotics (Obara and Nakae 1991).
Pathogenicity
The ability of Acinetobacter to resist innate immune mechanisms allows them to grow in numbers, resulting in sepsis. Capsule polysaccharide and OmpA are amongst the crucial antigenic factors that permit immune evasion. Furthermore, lipopolysaccharides, the iron acquisition system, phospholipase D, outer membrane vesicles, and penicillin binding proteins facilitate in vivo survival of the pathogens by neutralizing host immune response (Ayoub Moubareck and Halat 2020). Exposure to broad-spectrum antibiotics, the presence and duration of invasive surgery, burns, and other factors like ICUs, use of devices such as endotracheal tubes, catheters, and mechanical ventilation are strongly linked to Acinetobacter infections. Acinetobacter can be extremely virulent and cause an invasive catastrophe, as seen by the incidence of fulminant community acquired infections (Meumann et al. 2019). Some of the major pathogenic species along with their key attributes have been summarized in Table 1.
Organism . | Average GC% and protein count . | Pathogenicity/Infections . | Antibiotic resistance genes/enzymes . | Other attributes . | References . |
---|---|---|---|---|---|
A. baumannii | 39, 3678 | Ventilator-associated pneumonia, sepsis, UTIs, and skin and soft-tissue infection | MBLs genes of blaOXA-23, blaOXA-40, and blaOXA-58 efflux pumping systems, porins | Degradation of propanil (herbicide) MDR Monophyletic origin Member of ACB complex | Tayabali et al. (2012), Chakravarty 2020, Mea et al. (2021) |
A. bereziniae | 37.9, 4184 | Bacteremia, especially in immunocompromised patients | blaOXA-58 | Formerly known as Acinetobacter genomospecies 10 MDR species Opportunistic in nature | Favaro et al. (2019), Lee et al. (2020), Reyes et al. (2020) |
A. calcoaceticus | 38.7, 3592 | Causes pneumonia and other hospital-related infections | blaOXA-822(Class-D) | Cadmium and antibiotic-resistant Reported to be pathogenic in dogs and cat | Obara and Nakae (1991), Glew et al. (1977), Retailliau et al. 1979 |
A. haemolyticus | 39.5, 3095 | Not mentioned, reported pathogenic | blaOXA-265, blaNDM-1, aphA6, and a resistance-nodulation-cell division-type efflux pump | MDR Can produce phosphate binding exo-biopolymer Aerobic denitrification | Kaur and Ghosh (2015), Bello-López et al. (2019), Bai et al. (2020) |
A. johnsonii | 41.4, 3291 | Opportunistic pathogen, nematocidal activity against round worm | blaOXA-23 | Degrades naphthalene (NAP) and anthracene (ANT) MDR | Tian et al. (2016), Jiang et al. (2018), Jia et al. (2021) |
A. junii | 38.7, 3063 | Associated with UTI and outbreaks of sepsis in immuno-compromised patients | Carbapenem resistance, blaOXA-24, and blaOXA-30 | Rarely pathogenic in humans | Abo-Zed (2020), Kollimuttathuillam et al. (2021), Lasarte-Monterrubio et al. (2022) |
A. nosocomialis | 38.7, 3606 | Pathogenic | blaOXA-24/50 | Member of ACB complex Carbapenem and colistin resistant | Teixeira et al. (2013), Subhadra et al. (2020), Lasarte-Monterrubio et al. (2022) |
A. pittii | 38.8, 3685 | Causes nosocomial infections, fish pathogenesis reported | blaNDM-1, blaOXA-820, blaADC-43, and aphA6 reported | Member of ACB complex. Carbapenem-resistant phosphate-solubilizing | Iimura et al. (2020), He and Wan (2021) |
A. seifertii | 38.6, 3651 | Bacteremia | Produces oxa-58, MBLs-2 | Belongs to ACB complex Resistance to levofloxacin and carbapenems, but not colistin | Kishii et al. (2016), Furlan et al. (2019), Na et al. (2021) |
A. towneri | 41.3, 2614 | Human infections | Plasmid-mediated tet (X3) gene, also produces MBLs-1 | MDR including tigecycline | Ma et al. (2020), Maehana et al. (2021), Wang et al. (2020) |
A. ursingii | 40.1, 3173 | Blood stream infections | MBLs-producing | Affects immunocompromised and terminally ill patients. Antibiotic resistance | Faccone et al. (2019), Daniel et al. (2021) |
A. kookii | 43, 2828 | Polyarthritis in giraffe | Unknown | Similar to A. beijerincki Degrades 17α-ethinylestradiol | Schwarz et al. (2020), Palma et al. (2021) |
A. lwoffii | 42.95, 3100 | Emerging pathogen in fish, causes bacteremia, pneumonia, meningitis, and gastritis in humans | Unknown | Dark-green pigmentation reported | Cao et al. (2018), Kulkarni et al. (2021) |
A. colistiniresistens | 41.3, 3547 | Blood stream infections | Produces Imp-34- and oxa-58 | Previously known as Acinetobacter genomic species 13BJ/14TU Intrinsic resistance to colistin Carbapenem-resistant | Suzuki et al. (2019), Brasiliense et al. (2021) |
A. indicus | 45.8, 2733 | Not reported | blaNDM-1 and tet(X) | Biotechnologically significant (lipase and biosurfactant production) Opportunistic pathogen | He et al. (2020), Tang et al. (2021) |
A. schindleri | 42.5, 2956 | Bacteremia in humans reported | Unknown | Could not use furfural as sole carbon source Opportunistic pathogen | Kee et al. (2018), Mlynarcik et al. (2019), Arteaga et al. (2021) |
A. radioresistens | 41.8, 2881 | Bacteremia, pneumonia, and hepatic hydrothorax | blaOXA-23-like gene tet(B), aph(3′)-Vla, strA, and strB, | Rarely infects human application in soil bioremediation resistant to ampicillin, ceftriaxone, ceftazidime, cefotaxime, streptomycin, and kanamycin | Arvay et al. (2021), Opazo-Capurro et al. (2019), Liu et al. (2020) |
Organism . | Average GC% and protein count . | Pathogenicity/Infections . | Antibiotic resistance genes/enzymes . | Other attributes . | References . |
---|---|---|---|---|---|
A. baumannii | 39, 3678 | Ventilator-associated pneumonia, sepsis, UTIs, and skin and soft-tissue infection | MBLs genes of blaOXA-23, blaOXA-40, and blaOXA-58 efflux pumping systems, porins | Degradation of propanil (herbicide) MDR Monophyletic origin Member of ACB complex | Tayabali et al. (2012), Chakravarty 2020, Mea et al. (2021) |
A. bereziniae | 37.9, 4184 | Bacteremia, especially in immunocompromised patients | blaOXA-58 | Formerly known as Acinetobacter genomospecies 10 MDR species Opportunistic in nature | Favaro et al. (2019), Lee et al. (2020), Reyes et al. (2020) |
A. calcoaceticus | 38.7, 3592 | Causes pneumonia and other hospital-related infections | blaOXA-822(Class-D) | Cadmium and antibiotic-resistant Reported to be pathogenic in dogs and cat | Obara and Nakae (1991), Glew et al. (1977), Retailliau et al. 1979 |
A. haemolyticus | 39.5, 3095 | Not mentioned, reported pathogenic | blaOXA-265, blaNDM-1, aphA6, and a resistance-nodulation-cell division-type efflux pump | MDR Can produce phosphate binding exo-biopolymer Aerobic denitrification | Kaur and Ghosh (2015), Bello-López et al. (2019), Bai et al. (2020) |
A. johnsonii | 41.4, 3291 | Opportunistic pathogen, nematocidal activity against round worm | blaOXA-23 | Degrades naphthalene (NAP) and anthracene (ANT) MDR | Tian et al. (2016), Jiang et al. (2018), Jia et al. (2021) |
A. junii | 38.7, 3063 | Associated with UTI and outbreaks of sepsis in immuno-compromised patients | Carbapenem resistance, blaOXA-24, and blaOXA-30 | Rarely pathogenic in humans | Abo-Zed (2020), Kollimuttathuillam et al. (2021), Lasarte-Monterrubio et al. (2022) |
A. nosocomialis | 38.7, 3606 | Pathogenic | blaOXA-24/50 | Member of ACB complex Carbapenem and colistin resistant | Teixeira et al. (2013), Subhadra et al. (2020), Lasarte-Monterrubio et al. (2022) |
A. pittii | 38.8, 3685 | Causes nosocomial infections, fish pathogenesis reported | blaNDM-1, blaOXA-820, blaADC-43, and aphA6 reported | Member of ACB complex. Carbapenem-resistant phosphate-solubilizing | Iimura et al. (2020), He and Wan (2021) |
A. seifertii | 38.6, 3651 | Bacteremia | Produces oxa-58, MBLs-2 | Belongs to ACB complex Resistance to levofloxacin and carbapenems, but not colistin | Kishii et al. (2016), Furlan et al. (2019), Na et al. (2021) |
A. towneri | 41.3, 2614 | Human infections | Plasmid-mediated tet (X3) gene, also produces MBLs-1 | MDR including tigecycline | Ma et al. (2020), Maehana et al. (2021), Wang et al. (2020) |
A. ursingii | 40.1, 3173 | Blood stream infections | MBLs-producing | Affects immunocompromised and terminally ill patients. Antibiotic resistance | Faccone et al. (2019), Daniel et al. (2021) |
A. kookii | 43, 2828 | Polyarthritis in giraffe | Unknown | Similar to A. beijerincki Degrades 17α-ethinylestradiol | Schwarz et al. (2020), Palma et al. (2021) |
A. lwoffii | 42.95, 3100 | Emerging pathogen in fish, causes bacteremia, pneumonia, meningitis, and gastritis in humans | Unknown | Dark-green pigmentation reported | Cao et al. (2018), Kulkarni et al. (2021) |
A. colistiniresistens | 41.3, 3547 | Blood stream infections | Produces Imp-34- and oxa-58 | Previously known as Acinetobacter genomic species 13BJ/14TU Intrinsic resistance to colistin Carbapenem-resistant | Suzuki et al. (2019), Brasiliense et al. (2021) |
A. indicus | 45.8, 2733 | Not reported | blaNDM-1 and tet(X) | Biotechnologically significant (lipase and biosurfactant production) Opportunistic pathogen | He et al. (2020), Tang et al. (2021) |
A. schindleri | 42.5, 2956 | Bacteremia in humans reported | Unknown | Could not use furfural as sole carbon source Opportunistic pathogen | Kee et al. (2018), Mlynarcik et al. (2019), Arteaga et al. (2021) |
A. radioresistens | 41.8, 2881 | Bacteremia, pneumonia, and hepatic hydrothorax | blaOXA-23-like gene tet(B), aph(3′)-Vla, strA, and strB, | Rarely infects human application in soil bioremediation resistant to ampicillin, ceftriaxone, ceftazidime, cefotaxime, streptomycin, and kanamycin | Arvay et al. (2021), Opazo-Capurro et al. (2019), Liu et al. (2020) |
Organism . | Average GC% and protein count . | Pathogenicity/Infections . | Antibiotic resistance genes/enzymes . | Other attributes . | References . |
---|---|---|---|---|---|
A. baumannii | 39, 3678 | Ventilator-associated pneumonia, sepsis, UTIs, and skin and soft-tissue infection | MBLs genes of blaOXA-23, blaOXA-40, and blaOXA-58 efflux pumping systems, porins | Degradation of propanil (herbicide) MDR Monophyletic origin Member of ACB complex | Tayabali et al. (2012), Chakravarty 2020, Mea et al. (2021) |
A. bereziniae | 37.9, 4184 | Bacteremia, especially in immunocompromised patients | blaOXA-58 | Formerly known as Acinetobacter genomospecies 10 MDR species Opportunistic in nature | Favaro et al. (2019), Lee et al. (2020), Reyes et al. (2020) |
A. calcoaceticus | 38.7, 3592 | Causes pneumonia and other hospital-related infections | blaOXA-822(Class-D) | Cadmium and antibiotic-resistant Reported to be pathogenic in dogs and cat | Obara and Nakae (1991), Glew et al. (1977), Retailliau et al. 1979 |
A. haemolyticus | 39.5, 3095 | Not mentioned, reported pathogenic | blaOXA-265, blaNDM-1, aphA6, and a resistance-nodulation-cell division-type efflux pump | MDR Can produce phosphate binding exo-biopolymer Aerobic denitrification | Kaur and Ghosh (2015), Bello-López et al. (2019), Bai et al. (2020) |
A. johnsonii | 41.4, 3291 | Opportunistic pathogen, nematocidal activity against round worm | blaOXA-23 | Degrades naphthalene (NAP) and anthracene (ANT) MDR | Tian et al. (2016), Jiang et al. (2018), Jia et al. (2021) |
A. junii | 38.7, 3063 | Associated with UTI and outbreaks of sepsis in immuno-compromised patients | Carbapenem resistance, blaOXA-24, and blaOXA-30 | Rarely pathogenic in humans | Abo-Zed (2020), Kollimuttathuillam et al. (2021), Lasarte-Monterrubio et al. (2022) |
A. nosocomialis | 38.7, 3606 | Pathogenic | blaOXA-24/50 | Member of ACB complex Carbapenem and colistin resistant | Teixeira et al. (2013), Subhadra et al. (2020), Lasarte-Monterrubio et al. (2022) |
A. pittii | 38.8, 3685 | Causes nosocomial infections, fish pathogenesis reported | blaNDM-1, blaOXA-820, blaADC-43, and aphA6 reported | Member of ACB complex. Carbapenem-resistant phosphate-solubilizing | Iimura et al. (2020), He and Wan (2021) |
A. seifertii | 38.6, 3651 | Bacteremia | Produces oxa-58, MBLs-2 | Belongs to ACB complex Resistance to levofloxacin and carbapenems, but not colistin | Kishii et al. (2016), Furlan et al. (2019), Na et al. (2021) |
A. towneri | 41.3, 2614 | Human infections | Plasmid-mediated tet (X3) gene, also produces MBLs-1 | MDR including tigecycline | Ma et al. (2020), Maehana et al. (2021), Wang et al. (2020) |
A. ursingii | 40.1, 3173 | Blood stream infections | MBLs-producing | Affects immunocompromised and terminally ill patients. Antibiotic resistance | Faccone et al. (2019), Daniel et al. (2021) |
A. kookii | 43, 2828 | Polyarthritis in giraffe | Unknown | Similar to A. beijerincki Degrades 17α-ethinylestradiol | Schwarz et al. (2020), Palma et al. (2021) |
A. lwoffii | 42.95, 3100 | Emerging pathogen in fish, causes bacteremia, pneumonia, meningitis, and gastritis in humans | Unknown | Dark-green pigmentation reported | Cao et al. (2018), Kulkarni et al. (2021) |
A. colistiniresistens | 41.3, 3547 | Blood stream infections | Produces Imp-34- and oxa-58 | Previously known as Acinetobacter genomic species 13BJ/14TU Intrinsic resistance to colistin Carbapenem-resistant | Suzuki et al. (2019), Brasiliense et al. (2021) |
A. indicus | 45.8, 2733 | Not reported | blaNDM-1 and tet(X) | Biotechnologically significant (lipase and biosurfactant production) Opportunistic pathogen | He et al. (2020), Tang et al. (2021) |
A. schindleri | 42.5, 2956 | Bacteremia in humans reported | Unknown | Could not use furfural as sole carbon source Opportunistic pathogen | Kee et al. (2018), Mlynarcik et al. (2019), Arteaga et al. (2021) |
A. radioresistens | 41.8, 2881 | Bacteremia, pneumonia, and hepatic hydrothorax | blaOXA-23-like gene tet(B), aph(3′)-Vla, strA, and strB, | Rarely infects human application in soil bioremediation resistant to ampicillin, ceftriaxone, ceftazidime, cefotaxime, streptomycin, and kanamycin | Arvay et al. (2021), Opazo-Capurro et al. (2019), Liu et al. (2020) |
Organism . | Average GC% and protein count . | Pathogenicity/Infections . | Antibiotic resistance genes/enzymes . | Other attributes . | References . |
---|---|---|---|---|---|
A. baumannii | 39, 3678 | Ventilator-associated pneumonia, sepsis, UTIs, and skin and soft-tissue infection | MBLs genes of blaOXA-23, blaOXA-40, and blaOXA-58 efflux pumping systems, porins | Degradation of propanil (herbicide) MDR Monophyletic origin Member of ACB complex | Tayabali et al. (2012), Chakravarty 2020, Mea et al. (2021) |
A. bereziniae | 37.9, 4184 | Bacteremia, especially in immunocompromised patients | blaOXA-58 | Formerly known as Acinetobacter genomospecies 10 MDR species Opportunistic in nature | Favaro et al. (2019), Lee et al. (2020), Reyes et al. (2020) |
A. calcoaceticus | 38.7, 3592 | Causes pneumonia and other hospital-related infections | blaOXA-822(Class-D) | Cadmium and antibiotic-resistant Reported to be pathogenic in dogs and cat | Obara and Nakae (1991), Glew et al. (1977), Retailliau et al. 1979 |
A. haemolyticus | 39.5, 3095 | Not mentioned, reported pathogenic | blaOXA-265, blaNDM-1, aphA6, and a resistance-nodulation-cell division-type efflux pump | MDR Can produce phosphate binding exo-biopolymer Aerobic denitrification | Kaur and Ghosh (2015), Bello-López et al. (2019), Bai et al. (2020) |
A. johnsonii | 41.4, 3291 | Opportunistic pathogen, nematocidal activity against round worm | blaOXA-23 | Degrades naphthalene (NAP) and anthracene (ANT) MDR | Tian et al. (2016), Jiang et al. (2018), Jia et al. (2021) |
A. junii | 38.7, 3063 | Associated with UTI and outbreaks of sepsis in immuno-compromised patients | Carbapenem resistance, blaOXA-24, and blaOXA-30 | Rarely pathogenic in humans | Abo-Zed (2020), Kollimuttathuillam et al. (2021), Lasarte-Monterrubio et al. (2022) |
A. nosocomialis | 38.7, 3606 | Pathogenic | blaOXA-24/50 | Member of ACB complex Carbapenem and colistin resistant | Teixeira et al. (2013), Subhadra et al. (2020), Lasarte-Monterrubio et al. (2022) |
A. pittii | 38.8, 3685 | Causes nosocomial infections, fish pathogenesis reported | blaNDM-1, blaOXA-820, blaADC-43, and aphA6 reported | Member of ACB complex. Carbapenem-resistant phosphate-solubilizing | Iimura et al. (2020), He and Wan (2021) |
A. seifertii | 38.6, 3651 | Bacteremia | Produces oxa-58, MBLs-2 | Belongs to ACB complex Resistance to levofloxacin and carbapenems, but not colistin | Kishii et al. (2016), Furlan et al. (2019), Na et al. (2021) |
A. towneri | 41.3, 2614 | Human infections | Plasmid-mediated tet (X3) gene, also produces MBLs-1 | MDR including tigecycline | Ma et al. (2020), Maehana et al. (2021), Wang et al. (2020) |
A. ursingii | 40.1, 3173 | Blood stream infections | MBLs-producing | Affects immunocompromised and terminally ill patients. Antibiotic resistance | Faccone et al. (2019), Daniel et al. (2021) |
A. kookii | 43, 2828 | Polyarthritis in giraffe | Unknown | Similar to A. beijerincki Degrades 17α-ethinylestradiol | Schwarz et al. (2020), Palma et al. (2021) |
A. lwoffii | 42.95, 3100 | Emerging pathogen in fish, causes bacteremia, pneumonia, meningitis, and gastritis in humans | Unknown | Dark-green pigmentation reported | Cao et al. (2018), Kulkarni et al. (2021) |
A. colistiniresistens | 41.3, 3547 | Blood stream infections | Produces Imp-34- and oxa-58 | Previously known as Acinetobacter genomic species 13BJ/14TU Intrinsic resistance to colistin Carbapenem-resistant | Suzuki et al. (2019), Brasiliense et al. (2021) |
A. indicus | 45.8, 2733 | Not reported | blaNDM-1 and tet(X) | Biotechnologically significant (lipase and biosurfactant production) Opportunistic pathogen | He et al. (2020), Tang et al. (2021) |
A. schindleri | 42.5, 2956 | Bacteremia in humans reported | Unknown | Could not use furfural as sole carbon source Opportunistic pathogen | Kee et al. (2018), Mlynarcik et al. (2019), Arteaga et al. (2021) |
A. radioresistens | 41.8, 2881 | Bacteremia, pneumonia, and hepatic hydrothorax | blaOXA-23-like gene tet(B), aph(3′)-Vla, strA, and strB, | Rarely infects human application in soil bioremediation resistant to ampicillin, ceftriaxone, ceftazidime, cefotaxime, streptomycin, and kanamycin | Arvay et al. (2021), Opazo-Capurro et al. (2019), Liu et al. (2020) |
The most significant pathogenic species of the genus, A. baumannii, is one of the “ESKAPE” pathogens (Enterococcus faecium, Staphylococcus aureus, Klebsiella pneumonia, A. baumannii, Pseudomonas aeruginosa, and Enterobacter spp.) that can cause epidemics, especially in intensive care patients, and can be detected using 16s ribosomal RNA or seven housekeeping genes, rpoD, gyrB, gdhB, recA, gltA, gpi, and cpn60, through MLST. Ventilator-associated pneumonia, sepsis, UTIs, and skin and soft-tissue infections are the common diseases caused by A. baumannii (Hong and Kim 2021, Whiteway et al. 2022). Acinetobacter baumannii is a common cutaneous and upper respiratory tract colonizer that has been identified in human sputum, blood, urine, and faeces. Acinetobacter baumannii may survive for long durations on hospital surfaces and has been isolated from a variety of sources, including tap water faucets, angiography catheters, ventilators, air, gloves, and bed-side urinals (Ibrahim et al. 2021, Knauf et al. 2022). One-fifth of the infections in intensive care units globally are attributed to A. baumannii (Hazen et al. 2023). Crucial factors that assist in the pathogenicity of Acinetobacter baumanii and other pathogens are described in the following section.
Role of enzymes
The development of β-lactamases, particularly oxacillinases, linked to the promoter gene sequence ISAba1, is the basis of carbapenem resistance in Acinetobacter spp. (Yazdansetad et al. 2019, Bansal et al. 2020). The most common oxacillinase, transferred through mobile genetic elements, is blaOXA-23. The genes blaOXA-23 and blaOXA-51 had been previously linked to A. baumannii. However, recent reports have revealed the presence of these genes in other species as well. Acinetobacter pittii and A. nosocomialis are both reported to contain the blaOXA-23 gene (Meshkat et al. 2019). In addition, A. calcoaceticus, A. johnsonii, and A. haemolyticus, also contain oxacillinase-producing genes (Figueiredo et al. 2012).
Acinetobacter baumannii has an intrinsic class D type oxacillinase and a non-inducible chromosomal AmpC type cephalosporinase that is expressed at a low level. Oxacillinases from A. baumannii are OXA51 enzymes, which have more than 40 sequences and can hydrolyze many types of penicillin (Kadom et al. 2020, Farajzadeh et al. 2021). In addition to this, all strains of A. baumannii have chromosomal cephalosporinases (AmpC enzymes), which can hydrolyze almost all derivatives of penicillin and cephalosporin (Nordman et al. 2019). Metallo-β-lactamases (MBLs) are rarely found in A. baumannii, but their carbapenem-degrading activity is much stronger. All the β-lactams and carbapenems are hydrolyzed by these enzymes, except for aztreonam (Ramirez et al. 2020). Presence of MBLs was described in A. pittii (Deglmann et al. 2019).
Other crucial enzymes encoded by genus Acinetobacter include phospholipase C and D. Acinetobacter baumanni encodes for phospholipase D, while A. calcoaceticus encodes phospholipase C (Lehmann 2009). The two enzymes can be distinguished by their ability to digest a phospholipid molecule. Phospholipase D only digests the head group, whereas phospholipase C digests the phosphorylated head of a phospholipid molecule (Ayoub Moubareck and Halat 2020). Phospholipases are important hydrolytic enzymes with lipolytic action against phospholipids in human cell membranes serving as crucial virulence mechanism in A. baumannii. Phospholipase D increases the survival rate of bacteria in human serum, whereas phospholipase C damages epithelial cells. CpaA has been acquired recently by A. baumannii as a virulence factor that prevents blood coagulation by inactivating factor XII. As a result, CpaA inhibits the production of thrombin at intravascular regions, allowing A. baumannii to spread more widely (Harding et al. 2018, Morris et al. 2019).
Outer membrane proteins
OMPs found in Acinetobacter species contribute significantly to their pathogenicity and evolving antibiotic resistance. OMPs reportedly modulate the uptake antimicrobial agents preventing them from entering the bacterial system (Nie et al. 2020, Uppalapati et al. 2020). Furthermore, OMPs promote greater cell adherence and maintain the integrity of cell membranes. OMPs regulate the generation of outer membrane vesicles, which is crucial for antibiotic resistance and in formation of biofilms (Mozaheb et al. 2020). OmpA, CarO, and OmpW are the major types of porins discovered in species like A. baumannii and A. nosocomialis (Uppalapati et al. 2020).
The first porin to be discovered and characterized in Acinetobacter was OmpA (initially identified as Omp38). OmpA is comparatively impermeable with respect to other porins of similar size (e.g. OprF from E. coli), implicating it in antibiotic resistance. OmpA is highly conserved across species, having 92% amino acid sequence similarity with A. nosocomialis (Kwon et al. 2019) and plays a key role in its pathogenesis mechanism. OmpA expressed on the outer membrane of A. nosocomialis helps the bacteria in forming biofilms on abiotic surfaces and adherence to human epithelial cells facilitating cytotoxicity (Knight et al. 2018).
CarO, or carbapenem-susceptible porin, is an outer membrane channel protein with an 8-strand β-barrel structure that does not have a continuous channel but mediates the inflow of beta lactams (mostly imipenem) into A. baumannii. CarO provides carbapenem resistance to A. baumannii and works similar to other OMPs in enhancing cell attachment. It can also manipulate the immunological response of host cells by inhibiting micro-tubule associated protein thereby reducing the expression of pro-inflammatory genes. Recent evidence suggests that it plays a role in enhanced bacterial endurance inside the host as it acts as a selective filter dodging antimicrobials produced by the host (Zhu et al. 2019, Mea et al. 2021).
OmpW, identified in A. baumannii, is highly similar to OmpW found in P. aeruginosa and E. coli. Although the direct role of OmpW in A. baumannii is unknown, colistin-resistant A. baumannii mutant grown in vitro exhibited a decreased expression of the porin. However, a report from 2016 revealed the porin’s role in iron assimilation and its ability to accommodate colistin molecules (Sarshar et al. 2021, Gil et al. 2022).
Biofilm
The formation of biofilms is associated with increased bacterial survival rates and therefore augments pathogenicity. Pathogenic members of the genus like A. baumannii, A. pittii, and A. calcoaceticus reportedly form biofilms and are more resistant to antimicrobial treatments (Bravo et al. 2018, Knight et al. 2018). Moreover, the biofilm so formed enables them to remain active on biotic and abiotic surfaces while avoiding the host reaction (Gedefie et al. 2021). The biofilm-associated protein (bap) expressed by the bap gene is involved in intercellular adhesion, bacterial cell accumulation, and biofilm formation. The presence and expression of the blaPER-1 gene have also been associated with certain A. baumannii clinical isolates forming biofilms. Moreover, the development of pilus and exo-polysaccharide structures for defense against host is phenotypically linked to biofilm formation in A. baumannii strains that adhere to host cells (Yang et al. 2019, Gedefie et al. 2021, Mea et al. 2021). Similarly, as per a report, A. pittii may recover quickly from desiccation and express adhesion factors to infect new hosts as a result of biofilm formation (Bravo et al. 2018).
Efflux pumping systems
Efflux pumps serve as potent mechanisms for preventing antibiotics from entering the bacterial cell and induce resistance. Antimicrobials are expelled from the bacterial cell through these pumps resulting in lower drug accumulation therefore higher minimum inhibitory concentrations (MICs) for several antibiotic families such as β-lactams, quinolones, and aminoglycosides. Efflux pumps are essential for the extrusion of bile molecules, fatty acids, and peptides, as well as the active secretion of virulence factors such as siderophores in other Gram-negative bacteria. AceI and the AdeABC efflux pumps in Acinetobacter induce resistance to aminoglycosides and biocides, respectively (Morris et al. 2019).
The small multidrug resistance (SMR) family, the resistance-nodulation-division (RND) family, the major facilitator superfamily (MFS), and the multidrug and toxic compound extrusion (MATE) family are the four types of efflux pumps prevalent in A. baumannii. These families and their membrane-associated transporters have been shown to target specific antibiotic classes (Chakravarty 2020). Narrow-spectrum pumps from MFS include minocycline (TetB) resistance pumps and tetracycline (TetA, TetB) pumps, as well as the CmlA system that extrudes chloramphenicol. Two RND pump systems found in A. baumannii are AdeABC and AdeIJK (Xu et al. 2019). Chloramphenicol, aminoglycosides, cefotaxime, fluoroquinolones, tetracyclines, erythromycin, and trimethoprim are all pumped out by the AdeABC efflux pump in A. baumanni. AdeIJK, the second RND pump, prefers amphiphilic molecules as a substrate and works in tandem with AdeABC to promote tigecycline resistance. AbeM, a pump from the MATE family, has been implicated in lowering sensitivity toward erythromycin, quinolones, gentamicin, trimethoprim, chloramphenicol, and kanamycin upon overexpression. AbeS, a member of the SMR family of bacterial integral membrane proteins is linked to Chloramphenicol, quinolone, and macrolide resistance (Darby et al. 2023, Naidu et al. 2023).
In addition to antibiotic resistance mechanisms, pathogenesis, cell multiplication, and biofilm development are all known to be influenced by multidrug efflux pumping systems in A. nosocomialis. AcrR regulates the transcription of the AcrAB efflux pump in A. nosocomialis. The acrAB operon encoding AcrA and AcrB has also been discovered in A. nosocomialis and is significantly similar to arpAB operon involved in aminoglycoside resistance in A. baumannii (Subhadra et al. 2020).
Quorum sensing
Quorum sensing (QS) is a mode of communication between bacteria in order to maintain population density, using signal molecules known as “auto-inducers.” The LuxI/LuxR system is commonly found in various Gram-negative bacteria and is analogous to the two components of A. baumannii's QS circuit; the AbaI inducer and its corresponding receptor AbaR. AbaR serves as an acyl homoserine lactone (AHL) receptor protein, while AbaI is a sensor protein that acts as an auto-inducer synthase to produce AHL signal molecules. When AHL binds to AbaR, a series of reactions are triggered. According to recent studies, QS can be crucial for the development of biofilms, which act as insulation and help organisms live in hostile conditions and develop drug resistance (Saipriya et al. 2020). In addition, AnoI/AnoR, similar to AbaI/AbaR regulatory systems, were discovered in A. nosocomialis. The LuxI- and LuxR-type proteins AnoI and AnoR make up the QS system. AnoI is the producer of the QS signal N-3-hydroxydodecanoyl-L-homoserine lactone in A. nosocomialis. The formation of A. nosocomialis biofilm is significantly influenced by surface motility, which is further mediated by this QS regulating network (Subhadra et al. 2019).
Ecological/industrial significance
Another facet of the genus Acinetobacter entails its role in rescuing the environment by degradation of contaminants such as biphenyl, phenol, crude oil, acetonitrile, removal of phosphate from wastes, and many more. Some species can be used for producing fermentation-based industrial goods such as lipases, proteases, bio-emulsifiers, and a variety of biopolymeric compounds (Oanh et al. 2020, Patel et al. 2021). The ability to produce lipase is associated with hydrocarbon breakdown. They are frequently isolated from waste water treatment plants and sewage, which include high levels of petroleum-related hydrocarbons and other xenobiotics (Chen et al. 2019). In Thailand, e.g. Acinetobacter species strain MUB1 was identified with a remarkable ability to digest crude oil (Ecker et al. 2006). Furthermore, A. venetianus species contain marine hydrocarbon-degrading strains and have been recommended as an attractive model system for researching the mechanisms behind the process of alkane degradation, as well as a good platform for bioremediation of contaminated environments in general (Alattraqchi et al. 2021). A short description of various ecological applications of the Acinetobacter species has been included in the following sections and summarized in Table 2.
Biotechnological application . | Organisms . | Degradation/production potential . | References . |
---|---|---|---|
Phenol degradation | A. calcoaceticus | 91.6% of 0.8 g/l phenol in 48 h | Irankhah et al. (2019) |
A. radioresistens | 99% of 450 mg/kg of phenol-contaminated soil | Liu et al. (2020) | |
A. lwoffii | 41.67 mg/l per hour | Irankhah et al. (2019) | |
A. tandoii | 100% degradation at the concentration of 280 mg/l | Van et al. (2019) | |
Nitrogen assimilation and removal | A. boisseri | Not mentioned | Alvarez-Perez et al. () |
A. calcoaceticus | Capable of nitrogen removal under low temperature conditions | Wu et al. (2022) | |
A. nectaris | Not mentioned | Alvarez-Perez et al. () | |
Chromium reduction | A. bouvetii | Able to reduce 40% chromium absorbed by plant roots | Qadir et al. (2021) |
Bioremediation of heavy metals | A. imdicus | Can reduce chromium(IV) and mercury(II) | Hu et al. (2021) |
Hydrocarbon degradation | A. lwoffii | Can degrade C13- C35 n-alkanes in crude oil | Liu et al. (2020) |
A. pitti | Can degrade 88% of crude oil | Chettri et al. (2019) | |
A. baumannii | Can degrade 76% diesel and 90% paraffins | Kumar and De (2023) | |
Dye discoloration and degradation | A. pittii | Can degrade 84% methylene blue in 24 h | Ogunlaja et al. (2020) |
A. calcoaceticus | Azo dye amaranth degradation with 90% efficiency | Ameenudeen et al. (2021) | |
A. haemolyticus | Can degrade methylene green, basic violet, and acid blue dyes | Hossain et al. (2022) | |
A. baumannii | Decolourized 90% of 500 mg/l of azo dye | Shreedharan et al. (2021) | |
Toulene | A. junnii | Can degrade 80% of 50 ppm toluene within 72 h | Singh et al. (2018) |
Diesel degradation | A. vivani | Can use diesel as the sole source of carbon | Zhang et al. (2022) |
A. haemolyticus | Diesel degradation facilitated by kurstakin molecules | Diallo et al. (2021) | |
A. baumannii | Can degrade 99% diesel at pH 7 | Imron et al. (2018) | |
A. lwoffii | Bioremediation in marine environment | Imron et al. (2020) | |
A. calcoaceticus | Presence of diesel degrading genes alkM and xcpR | Ho et al. (2020) | |
Polyurethane degradation | A. baumannii | Grows on polyurethane | Espinosa et al. (2020) |
Crude oil degradation | A. venetianus | Can degrade upto 60.6% waxy crude oil | Liu et al. (2021), Wang et al. (2019) |
A. pitti | Can degrade 36% percent crude oil in 21 days at 10 g/l | Wang et al. (2019) | |
Insecticide degradation | A. schindleri | Can degrade insecticides α-endosulfan and α-cypermethrin with more than 60% efficiency | Gur and Algur (2022) |
Furfural degradation | A. baylyi | Can degrade 1 g furfural in 1 h | Arteaga et al. (2021) |
Fipronil degradation | A. calcoaceticus | 86.6% degradation after 45 days | Uniyal et al. (2016) |
A. oleivorans | 89.7% degradation after 45 days | Uniyal et al. (2016) | |
NAP, ANT, and other polyaromatic hydrocarbon degradation | A. johnsonii | Can degrade 200 mg/l NAP and 1950 mg/l ANT | Jiang et al. (2018) |
A. baumannii | Efficient at 300 mg/l concentration of pyrene | Gupta et al. (2020) | |
Catechol production | A. bouveti | Produces novel biscatechol siderophores namely propanochelin, butanochelin, and pentanochelin | Reitz and Butler (2020) |
N-acetyl-β-D-glucosamine production | A. parvus | Can convert chitin to N-acetyl-β-D-glucosamine | Kim et al. (2017) |
Cellulase production | A. junnii | Capable of producing cellulase at 112.38 U/ml | Banerjee et al. (2020) |
Mevalonate production |
| Produces mevalonate from lignin derived compounds by β-keto adipose pathway | Arvay et al. (2021) |
Lipase production | A. indicus | Efficient lipase producer from industrial waste | Patel et al. (2021) |
A. radioresistens | Can produce 4.16 U/ml (at pH 9) of enzyme after 72 h | Gupta et al. (2018) | |
A. haemolyticus | Produces lipase which is highly stable at 4°C displaying 90% activity even after 2 months | Sarac et al. (2016) | |
A. calcoaceticus | A. calcoaceticus Rag-1 produces the most widely studied Emulsan (1000 kDa) | Mujumdar et al. (2019) | |
Bioemulsion and biosurfactant production | A. pittii | Can produce 0.57 g/l lipopeptide biosurfactant when incubated with 1% (v/v) crude oil | Mujumdar et al. (2019) |
A. beijerinckii | Produces the only bioemulsion that contains lipoprotein while others contain polysaccharides | Mujumdar et al. (2019) | |
A. baumannii | Produces lipoglycan, using edible oil as carbon source | Mujumdar et al. (2019) | |
A. radioresistens | Produces alsan, utilizing carbon source as ethanol | Mujumdar et al. (2019) | |
A. bouvetii | Produces the highest molecular weight lipo-hetero-polysaccharide bioemulsifier | Mujumdar et al. (2019) | |
A. lwoffii | Produces proteoglycan in presence of castor oil as carbon source | Mujumdar et al. (2019) | |
Phenanthrene degradation | A. venetianus | Phenanthrene degradation ability facilitated by ball-milled biochar (2.4 times increase) | Guo et al. (2022) |
Proteases production | A. pittii | Yields as high as 11–12 U/ml with de-oiled neem seed cake | Reddy et al. (2022) |
Biohydrogen production | A. junii | Can produce up to 566 ml/l of H2 from wastewaters at pH 7.5 | Murugan et al. (2021) |
Biodiesel degradation | A. oleivorans | Uses biodiesel as a sole source of carbon at 30°C | Deems et al. (2021) |
Polyhydroxybutyrate (PHB) production | A. nosocomialis | Can yield up to 5.88 g/l of PHB under optimal conditions | Ranganadha et al. (2020) |
Biotechnological application . | Organisms . | Degradation/production potential . | References . |
---|---|---|---|
Phenol degradation | A. calcoaceticus | 91.6% of 0.8 g/l phenol in 48 h | Irankhah et al. (2019) |
A. radioresistens | 99% of 450 mg/kg of phenol-contaminated soil | Liu et al. (2020) | |
A. lwoffii | 41.67 mg/l per hour | Irankhah et al. (2019) | |
A. tandoii | 100% degradation at the concentration of 280 mg/l | Van et al. (2019) | |
Nitrogen assimilation and removal | A. boisseri | Not mentioned | Alvarez-Perez et al. () |
A. calcoaceticus | Capable of nitrogen removal under low temperature conditions | Wu et al. (2022) | |
A. nectaris | Not mentioned | Alvarez-Perez et al. () | |
Chromium reduction | A. bouvetii | Able to reduce 40% chromium absorbed by plant roots | Qadir et al. (2021) |
Bioremediation of heavy metals | A. imdicus | Can reduce chromium(IV) and mercury(II) | Hu et al. (2021) |
Hydrocarbon degradation | A. lwoffii | Can degrade C13- C35 n-alkanes in crude oil | Liu et al. (2020) |
A. pitti | Can degrade 88% of crude oil | Chettri et al. (2019) | |
A. baumannii | Can degrade 76% diesel and 90% paraffins | Kumar and De (2023) | |
Dye discoloration and degradation | A. pittii | Can degrade 84% methylene blue in 24 h | Ogunlaja et al. (2020) |
A. calcoaceticus | Azo dye amaranth degradation with 90% efficiency | Ameenudeen et al. (2021) | |
A. haemolyticus | Can degrade methylene green, basic violet, and acid blue dyes | Hossain et al. (2022) | |
A. baumannii | Decolourized 90% of 500 mg/l of azo dye | Shreedharan et al. (2021) | |
Toulene | A. junnii | Can degrade 80% of 50 ppm toluene within 72 h | Singh et al. (2018) |
Diesel degradation | A. vivani | Can use diesel as the sole source of carbon | Zhang et al. (2022) |
A. haemolyticus | Diesel degradation facilitated by kurstakin molecules | Diallo et al. (2021) | |
A. baumannii | Can degrade 99% diesel at pH 7 | Imron et al. (2018) | |
A. lwoffii | Bioremediation in marine environment | Imron et al. (2020) | |
A. calcoaceticus | Presence of diesel degrading genes alkM and xcpR | Ho et al. (2020) | |
Polyurethane degradation | A. baumannii | Grows on polyurethane | Espinosa et al. (2020) |
Crude oil degradation | A. venetianus | Can degrade upto 60.6% waxy crude oil | Liu et al. (2021), Wang et al. (2019) |
A. pitti | Can degrade 36% percent crude oil in 21 days at 10 g/l | Wang et al. (2019) | |
Insecticide degradation | A. schindleri | Can degrade insecticides α-endosulfan and α-cypermethrin with more than 60% efficiency | Gur and Algur (2022) |
Furfural degradation | A. baylyi | Can degrade 1 g furfural in 1 h | Arteaga et al. (2021) |
Fipronil degradation | A. calcoaceticus | 86.6% degradation after 45 days | Uniyal et al. (2016) |
A. oleivorans | 89.7% degradation after 45 days | Uniyal et al. (2016) | |
NAP, ANT, and other polyaromatic hydrocarbon degradation | A. johnsonii | Can degrade 200 mg/l NAP and 1950 mg/l ANT | Jiang et al. (2018) |
A. baumannii | Efficient at 300 mg/l concentration of pyrene | Gupta et al. (2020) | |
Catechol production | A. bouveti | Produces novel biscatechol siderophores namely propanochelin, butanochelin, and pentanochelin | Reitz and Butler (2020) |
N-acetyl-β-D-glucosamine production | A. parvus | Can convert chitin to N-acetyl-β-D-glucosamine | Kim et al. (2017) |
Cellulase production | A. junnii | Capable of producing cellulase at 112.38 U/ml | Banerjee et al. (2020) |
Mevalonate production |
| Produces mevalonate from lignin derived compounds by β-keto adipose pathway | Arvay et al. (2021) |
Lipase production | A. indicus | Efficient lipase producer from industrial waste | Patel et al. (2021) |
A. radioresistens | Can produce 4.16 U/ml (at pH 9) of enzyme after 72 h | Gupta et al. (2018) | |
A. haemolyticus | Produces lipase which is highly stable at 4°C displaying 90% activity even after 2 months | Sarac et al. (2016) | |
A. calcoaceticus | A. calcoaceticus Rag-1 produces the most widely studied Emulsan (1000 kDa) | Mujumdar et al. (2019) | |
Bioemulsion and biosurfactant production | A. pittii | Can produce 0.57 g/l lipopeptide biosurfactant when incubated with 1% (v/v) crude oil | Mujumdar et al. (2019) |
A. beijerinckii | Produces the only bioemulsion that contains lipoprotein while others contain polysaccharides | Mujumdar et al. (2019) | |
A. baumannii | Produces lipoglycan, using edible oil as carbon source | Mujumdar et al. (2019) | |
A. radioresistens | Produces alsan, utilizing carbon source as ethanol | Mujumdar et al. (2019) | |
A. bouvetii | Produces the highest molecular weight lipo-hetero-polysaccharide bioemulsifier | Mujumdar et al. (2019) | |
A. lwoffii | Produces proteoglycan in presence of castor oil as carbon source | Mujumdar et al. (2019) | |
Phenanthrene degradation | A. venetianus | Phenanthrene degradation ability facilitated by ball-milled biochar (2.4 times increase) | Guo et al. (2022) |
Proteases production | A. pittii | Yields as high as 11–12 U/ml with de-oiled neem seed cake | Reddy et al. (2022) |
Biohydrogen production | A. junii | Can produce up to 566 ml/l of H2 from wastewaters at pH 7.5 | Murugan et al. (2021) |
Biodiesel degradation | A. oleivorans | Uses biodiesel as a sole source of carbon at 30°C | Deems et al. (2021) |
Polyhydroxybutyrate (PHB) production | A. nosocomialis | Can yield up to 5.88 g/l of PHB under optimal conditions | Ranganadha et al. (2020) |
Biotechnological application . | Organisms . | Degradation/production potential . | References . |
---|---|---|---|
Phenol degradation | A. calcoaceticus | 91.6% of 0.8 g/l phenol in 48 h | Irankhah et al. (2019) |
A. radioresistens | 99% of 450 mg/kg of phenol-contaminated soil | Liu et al. (2020) | |
A. lwoffii | 41.67 mg/l per hour | Irankhah et al. (2019) | |
A. tandoii | 100% degradation at the concentration of 280 mg/l | Van et al. (2019) | |
Nitrogen assimilation and removal | A. boisseri | Not mentioned | Alvarez-Perez et al. () |
A. calcoaceticus | Capable of nitrogen removal under low temperature conditions | Wu et al. (2022) | |
A. nectaris | Not mentioned | Alvarez-Perez et al. () | |
Chromium reduction | A. bouvetii | Able to reduce 40% chromium absorbed by plant roots | Qadir et al. (2021) |
Bioremediation of heavy metals | A. imdicus | Can reduce chromium(IV) and mercury(II) | Hu et al. (2021) |
Hydrocarbon degradation | A. lwoffii | Can degrade C13- C35 n-alkanes in crude oil | Liu et al. (2020) |
A. pitti | Can degrade 88% of crude oil | Chettri et al. (2019) | |
A. baumannii | Can degrade 76% diesel and 90% paraffins | Kumar and De (2023) | |
Dye discoloration and degradation | A. pittii | Can degrade 84% methylene blue in 24 h | Ogunlaja et al. (2020) |
A. calcoaceticus | Azo dye amaranth degradation with 90% efficiency | Ameenudeen et al. (2021) | |
A. haemolyticus | Can degrade methylene green, basic violet, and acid blue dyes | Hossain et al. (2022) | |
A. baumannii | Decolourized 90% of 500 mg/l of azo dye | Shreedharan et al. (2021) | |
Toulene | A. junnii | Can degrade 80% of 50 ppm toluene within 72 h | Singh et al. (2018) |
Diesel degradation | A. vivani | Can use diesel as the sole source of carbon | Zhang et al. (2022) |
A. haemolyticus | Diesel degradation facilitated by kurstakin molecules | Diallo et al. (2021) | |
A. baumannii | Can degrade 99% diesel at pH 7 | Imron et al. (2018) | |
A. lwoffii | Bioremediation in marine environment | Imron et al. (2020) | |
A. calcoaceticus | Presence of diesel degrading genes alkM and xcpR | Ho et al. (2020) | |
Polyurethane degradation | A. baumannii | Grows on polyurethane | Espinosa et al. (2020) |
Crude oil degradation | A. venetianus | Can degrade upto 60.6% waxy crude oil | Liu et al. (2021), Wang et al. (2019) |
A. pitti | Can degrade 36% percent crude oil in 21 days at 10 g/l | Wang et al. (2019) | |
Insecticide degradation | A. schindleri | Can degrade insecticides α-endosulfan and α-cypermethrin with more than 60% efficiency | Gur and Algur (2022) |
Furfural degradation | A. baylyi | Can degrade 1 g furfural in 1 h | Arteaga et al. (2021) |
Fipronil degradation | A. calcoaceticus | 86.6% degradation after 45 days | Uniyal et al. (2016) |
A. oleivorans | 89.7% degradation after 45 days | Uniyal et al. (2016) | |
NAP, ANT, and other polyaromatic hydrocarbon degradation | A. johnsonii | Can degrade 200 mg/l NAP and 1950 mg/l ANT | Jiang et al. (2018) |
A. baumannii | Efficient at 300 mg/l concentration of pyrene | Gupta et al. (2020) | |
Catechol production | A. bouveti | Produces novel biscatechol siderophores namely propanochelin, butanochelin, and pentanochelin | Reitz and Butler (2020) |
N-acetyl-β-D-glucosamine production | A. parvus | Can convert chitin to N-acetyl-β-D-glucosamine | Kim et al. (2017) |
Cellulase production | A. junnii | Capable of producing cellulase at 112.38 U/ml | Banerjee et al. (2020) |
Mevalonate production |
| Produces mevalonate from lignin derived compounds by β-keto adipose pathway | Arvay et al. (2021) |
Lipase production | A. indicus | Efficient lipase producer from industrial waste | Patel et al. (2021) |
A. radioresistens | Can produce 4.16 U/ml (at pH 9) of enzyme after 72 h | Gupta et al. (2018) | |
A. haemolyticus | Produces lipase which is highly stable at 4°C displaying 90% activity even after 2 months | Sarac et al. (2016) | |
A. calcoaceticus | A. calcoaceticus Rag-1 produces the most widely studied Emulsan (1000 kDa) | Mujumdar et al. (2019) | |
Bioemulsion and biosurfactant production | A. pittii | Can produce 0.57 g/l lipopeptide biosurfactant when incubated with 1% (v/v) crude oil | Mujumdar et al. (2019) |
A. beijerinckii | Produces the only bioemulsion that contains lipoprotein while others contain polysaccharides | Mujumdar et al. (2019) | |
A. baumannii | Produces lipoglycan, using edible oil as carbon source | Mujumdar et al. (2019) | |
A. radioresistens | Produces alsan, utilizing carbon source as ethanol | Mujumdar et al. (2019) | |
A. bouvetii | Produces the highest molecular weight lipo-hetero-polysaccharide bioemulsifier | Mujumdar et al. (2019) | |
A. lwoffii | Produces proteoglycan in presence of castor oil as carbon source | Mujumdar et al. (2019) | |
Phenanthrene degradation | A. venetianus | Phenanthrene degradation ability facilitated by ball-milled biochar (2.4 times increase) | Guo et al. (2022) |
Proteases production | A. pittii | Yields as high as 11–12 U/ml with de-oiled neem seed cake | Reddy et al. (2022) |
Biohydrogen production | A. junii | Can produce up to 566 ml/l of H2 from wastewaters at pH 7.5 | Murugan et al. (2021) |
Biodiesel degradation | A. oleivorans | Uses biodiesel as a sole source of carbon at 30°C | Deems et al. (2021) |
Polyhydroxybutyrate (PHB) production | A. nosocomialis | Can yield up to 5.88 g/l of PHB under optimal conditions | Ranganadha et al. (2020) |
Biotechnological application . | Organisms . | Degradation/production potential . | References . |
---|---|---|---|
Phenol degradation | A. calcoaceticus | 91.6% of 0.8 g/l phenol in 48 h | Irankhah et al. (2019) |
A. radioresistens | 99% of 450 mg/kg of phenol-contaminated soil | Liu et al. (2020) | |
A. lwoffii | 41.67 mg/l per hour | Irankhah et al. (2019) | |
A. tandoii | 100% degradation at the concentration of 280 mg/l | Van et al. (2019) | |
Nitrogen assimilation and removal | A. boisseri | Not mentioned | Alvarez-Perez et al. () |
A. calcoaceticus | Capable of nitrogen removal under low temperature conditions | Wu et al. (2022) | |
A. nectaris | Not mentioned | Alvarez-Perez et al. () | |
Chromium reduction | A. bouvetii | Able to reduce 40% chromium absorbed by plant roots | Qadir et al. (2021) |
Bioremediation of heavy metals | A. imdicus | Can reduce chromium(IV) and mercury(II) | Hu et al. (2021) |
Hydrocarbon degradation | A. lwoffii | Can degrade C13- C35 n-alkanes in crude oil | Liu et al. (2020) |
A. pitti | Can degrade 88% of crude oil | Chettri et al. (2019) | |
A. baumannii | Can degrade 76% diesel and 90% paraffins | Kumar and De (2023) | |
Dye discoloration and degradation | A. pittii | Can degrade 84% methylene blue in 24 h | Ogunlaja et al. (2020) |
A. calcoaceticus | Azo dye amaranth degradation with 90% efficiency | Ameenudeen et al. (2021) | |
A. haemolyticus | Can degrade methylene green, basic violet, and acid blue dyes | Hossain et al. (2022) | |
A. baumannii | Decolourized 90% of 500 mg/l of azo dye | Shreedharan et al. (2021) | |
Toulene | A. junnii | Can degrade 80% of 50 ppm toluene within 72 h | Singh et al. (2018) |
Diesel degradation | A. vivani | Can use diesel as the sole source of carbon | Zhang et al. (2022) |
A. haemolyticus | Diesel degradation facilitated by kurstakin molecules | Diallo et al. (2021) | |
A. baumannii | Can degrade 99% diesel at pH 7 | Imron et al. (2018) | |
A. lwoffii | Bioremediation in marine environment | Imron et al. (2020) | |
A. calcoaceticus | Presence of diesel degrading genes alkM and xcpR | Ho et al. (2020) | |
Polyurethane degradation | A. baumannii | Grows on polyurethane | Espinosa et al. (2020) |
Crude oil degradation | A. venetianus | Can degrade upto 60.6% waxy crude oil | Liu et al. (2021), Wang et al. (2019) |
A. pitti | Can degrade 36% percent crude oil in 21 days at 10 g/l | Wang et al. (2019) | |
Insecticide degradation | A. schindleri | Can degrade insecticides α-endosulfan and α-cypermethrin with more than 60% efficiency | Gur and Algur (2022) |
Furfural degradation | A. baylyi | Can degrade 1 g furfural in 1 h | Arteaga et al. (2021) |
Fipronil degradation | A. calcoaceticus | 86.6% degradation after 45 days | Uniyal et al. (2016) |
A. oleivorans | 89.7% degradation after 45 days | Uniyal et al. (2016) | |
NAP, ANT, and other polyaromatic hydrocarbon degradation | A. johnsonii | Can degrade 200 mg/l NAP and 1950 mg/l ANT | Jiang et al. (2018) |
A. baumannii | Efficient at 300 mg/l concentration of pyrene | Gupta et al. (2020) | |
Catechol production | A. bouveti | Produces novel biscatechol siderophores namely propanochelin, butanochelin, and pentanochelin | Reitz and Butler (2020) |
N-acetyl-β-D-glucosamine production | A. parvus | Can convert chitin to N-acetyl-β-D-glucosamine | Kim et al. (2017) |
Cellulase production | A. junnii | Capable of producing cellulase at 112.38 U/ml | Banerjee et al. (2020) |
Mevalonate production |
| Produces mevalonate from lignin derived compounds by β-keto adipose pathway | Arvay et al. (2021) |
Lipase production | A. indicus | Efficient lipase producer from industrial waste | Patel et al. (2021) |
A. radioresistens | Can produce 4.16 U/ml (at pH 9) of enzyme after 72 h | Gupta et al. (2018) | |
A. haemolyticus | Produces lipase which is highly stable at 4°C displaying 90% activity even after 2 months | Sarac et al. (2016) | |
A. calcoaceticus | A. calcoaceticus Rag-1 produces the most widely studied Emulsan (1000 kDa) | Mujumdar et al. (2019) | |
Bioemulsion and biosurfactant production | A. pittii | Can produce 0.57 g/l lipopeptide biosurfactant when incubated with 1% (v/v) crude oil | Mujumdar et al. (2019) |
A. beijerinckii | Produces the only bioemulsion that contains lipoprotein while others contain polysaccharides | Mujumdar et al. (2019) | |
A. baumannii | Produces lipoglycan, using edible oil as carbon source | Mujumdar et al. (2019) | |
A. radioresistens | Produces alsan, utilizing carbon source as ethanol | Mujumdar et al. (2019) | |
A. bouvetii | Produces the highest molecular weight lipo-hetero-polysaccharide bioemulsifier | Mujumdar et al. (2019) | |
A. lwoffii | Produces proteoglycan in presence of castor oil as carbon source | Mujumdar et al. (2019) | |
Phenanthrene degradation | A. venetianus | Phenanthrene degradation ability facilitated by ball-milled biochar (2.4 times increase) | Guo et al. (2022) |
Proteases production | A. pittii | Yields as high as 11–12 U/ml with de-oiled neem seed cake | Reddy et al. (2022) |
Biohydrogen production | A. junii | Can produce up to 566 ml/l of H2 from wastewaters at pH 7.5 | Murugan et al. (2021) |
Biodiesel degradation | A. oleivorans | Uses biodiesel as a sole source of carbon at 30°C | Deems et al. (2021) |
Polyhydroxybutyrate (PHB) production | A. nosocomialis | Can yield up to 5.88 g/l of PHB under optimal conditions | Ranganadha et al. (2020) |
Phenol degradation
Due to its widespread use as a raw material, phenol makes up a sizable component of the industrial wastewater released from chemical factories. Bacterial phenol degradation involves the metabolic transformation of complicated aromatic metabolites into essential primary (3–4) carbon compounds for bacterial growth. Catechol is produced after phenol is first oxidized by phenol hydroxylase. It is then changed through a variety of ring-opening processes, such as the ortho and meta cleavage pathways, which are, respectively, mediated by catechol 1,2-dioxygenase and catechol 2,3-dioxygenase. Catechol is turned into cis-muconate and then succinyl-CoA in the ortho-cleavage process. Similarly, it is transformed into 2-hydroxymuconate semialdehyde, 2-keto-4-pentenoic acid via two pathways (Fig. 2), and then acetyl-CoA in the meta-cleavage pathway (Xu et al. 2021). One of the most effective phenol-degrading bacteria employed in soil bioremediation is A. radioresistens APH1 (Liu et al. 2020). A recent study revealed the potential role of A. lwoffii NL1 in the breakdown of phenol in waste water (Xu et al. 2021). Phenol can be used by A. tandoii as the only carbon source, and it can totally degrade phenol using both the ortho and meta pathways (Van et al. 2019). When compared to loose cells, Acinetobacter sp. strain AQ5NOL 1 encapsulated in gellan gum has a greater ability to break down phenol (Ahmad and Shamaan 2012).
Bio-emulsifier and biosurfactant production
When present in aqueous solutions and hydrocarbon mixtures, bio-emulsifiers and biosurfactants are the most significant active substances produced by microorganisms that play a crucial role in lowering surface and interfacial tensions. High-molecular-weight substances known as bio-emulsifiers are made up of intricate blends of polysaccharides, lipids, and proteins. They create stable emulsions by forging a strong bond on hydrocarbon surfaces. Contrarily, biosurfactants are low-molecular-weight substances that have the ability to lower surface and interfacial tension at the interfaces of phases, such as gas–liquid–solid interfaces. They produce stable emulsions as well and contain complex assemblages of proteins, glycolipids, and lipo-peptides (Markande et al. 2021). Many Acinetobacter species, including A. venetianus RAG-1, A. calcoaceticus RAG-1, A. calcoaceticus BD4 RAG-1, and A. radioresistens KA53 etc. are capable of producing polymeric bio-emulsions. The best emulsions produced by several Acinetobacter strains are emulsan, biodispersan, and alasan. The substrates that are insufficiently soluble in water are broken down using emulsan, generated by A. calcoaceticus RAG-1. It has also been claimed that the biosurfactant derived from A. junii B6 lowers the surface tension of cultured oil broth. Biosurfactant produced by Acinetobacter sp. ACMS25 was found to inhibit the growth of Xanthomonas oryzae XAV24. An important study revealed attenuated proliferation of lung cancer cells upon exposure to the biosurfactant produced by A. indicus M6, indicating its anticancer properties (Mujumdar et al. 2019, Markande et al. 2021).
Lipases
Lipases which hydrolyze lipids into fatty acids and glycerol at the water-oil interface and catalyze the processes of esterification and resolution find abundant use in the food, biofuel, dairy, cosmetic, and pharmaceutical industry (Costantini and Califano 2021). Lipolytic Acinetobacter strains have been isolated from a wide range of substrates, including human skin, dairy products etc. as well as from various soil and water environments. Lipolytic clinical strains frequently result in serious nosocomial infections in immunocompromised adults and newborns. Since bacteria use lipolysis appropriately to meet their specific needs during invasion of host cell targets, the lipase activity of pathogenic species may be a contributory factor in their pathogenicity. Together with their corresponding Lif chaperone, lipases are encoded in an operon. With the exception of A. calcoaceticus BD413, which has the reverse arrangement, the Lif chaperone is typically encoded downstream from the structural gene. For the mature lipase to be secreted, lipase genes (lip) must co-express with their cognate foldases. There have been many reports of Acinetobacter spp. producing lipases, including A. beijerinckii, A. baumanii, Acinetobacter nov. sp. KM109, A. radioresistens, A. haemolyticus CMC-1, A. calcoaceticus BD413, and Acinetobacter sp. RAG-1 (Furuni and Berger 2018, Liu et al. 2020). Very recently, A. indicus strain UBT1 was proved to efficient producer of lipase and biosurfactant using industrial waste as a sole source of carbon (Patel et al. 2021).
Wax esters
Wax esters, e.g. are prospective high-value lipids for the production of a wide variety of applicants, such as cosmetics, lubricants, medicines, printing, food supplies, etc. When nitrogen is scarce and carbon is limited, bacteria collect wax esters for storage (Ishige et al. 2003). Acinetobacter baylyi, a naturally existing producer of wax esters, is a suitable model organism for understanding the potential and modifiability of wax esters in natural hosts (Santala et al. 2014). Engineered A. baylyi ADP1 can produce three times as much wax via overexpression of fatty acyl-CoA reductase Acr1 and deletion of the gene aceA encoding for isocitrate lyase in the wax ester synthesis pathway (Luo et al. 2022). Similar to this, A. baylyi ADP1 naturally catabolizes aromatic substrates derived from lignin using the β-ketoadipate pathway to produce mevalonate from lignin-derived compounds (Arvay et al. 2021).
Crude oil degradation
Large amounts of poly-aromatic hydrocarbons, alkanes, and cycloalkanes, including benzene, xylenes, and toluene, are some of the hazardous substances found in crude oil (Appolinario et al. 2019). There are numerous Acinetobacter species that are able to break down crude oil and its hazardous byproducts. In 2019, Pradeep and colleagues showed that toluene could be broken down into non-toxic intermediate molecules by A. junii in petroleum-contaminated soil. Furthermore, combined cultures of Acinetobacter species and hydrocarbon-degrading fungus have been shown to be effective in breaking down crude oil because the fungus has a stronger ability to break down n-alkanes while the bacteria are efficient at breaking down other components like aromatic and branched alkanes. The combined culture of A. baumannii and Talaromyces sp. showed exceptional resistance to alkaline environment and a high ability to degrade crude oil (Zhang et al. 2021). Use of biosurfactant-producing Acinetobacter sp. Y2 in combination with the fungus Scedosporium doubled the breakdown of petroleum hydrocarbons in comparison to the fungus alone (Atakpa et al. 2022). Acinetobacter species with high methyl tolerance and lipase production ability, A. junii C69 and A. pittii C95 can also catalyze the conversion of soybean oil into biodiesel (Tan et al. 2022).
Diesel oil degradation
Diesel oil is an extremely complex mixture of alkanes, cycloalkanes, and aromatic hydrocarbons, including alcohols. Diesel oil and its derivatives are known soil pollutants that are phytotoxic to a range of plants and crops. Bio-remediation using bacteria like Acinetobacter to remove toxins from the environment, can mitigate the consequences (Czarny et al. 2020, Ho et al. 2020). One of the first studies on Acinetobacter strains, A. haemolyticus and A. johnsonii, that break down diesel oil was published in 2012. Over 90% of diesel oil could be degraded by either species (Yakasai et al. 2022). In course of two weeks, A. junii VA2 decomposed more than 75% of the applied diesel (Zhang et al. 2014). Another study revealed that A. calcoaceticus CA16 could grow in minimal medium, including diesel as the sole source of carbon, resulting in the breakdown of more than 90% of the aliphatic hydrocarbons and alkanes in diesel (Ho et al. 2020). According to a report, a bio-emulsion produced by A. lwoffii selectively dissolves various chain-length hydrocarbons in diesel (Imron et al. 2019). The degradation process of crude oil, diesel, petrol, n-alkanes, and other hydrocarbons by members of Acinetobacter genus have been included in Figs 2 and 3.
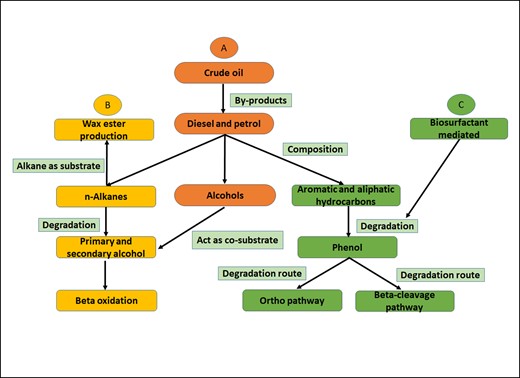
Chart for degradation of various contaminants like crude oil, diesel, petrol, n-alkanes, and other hydrocarbons by members of Acinetobacter genus. (A) The by-products of crude oil are diesel and petrol, which are composed of n-alkanes, alcohols, and aromatic and aliphatic hydrocarbons (Lee et al. 2012, Imron et al. 2019). (B) n-Alkanes can be used by Acinetobacter species for wax ester production (e.g.: Acinetobacter sp. strain M-1), whereas the degradation of n-alkanes forms intermediates like primary and secondary alcohols, which enter β-oxidation pathway (Arvay et al. 2021, Luo et al. 2022). (C) The aliphatic and aromatic hydrocarbons from crude oils can be degraded into phenol with the help of bacterial biosurfactant (e.g.: A. junii, A lwoffii, A. calcoaceticus CA16). Phenol is further degraded via ortho (A. lwoffii, A. calcoaceticus) and β-cleavage pathway (Acinetobacter sp. strain AQ5NOL1), as shown in Fig. 3 (Czarny et al. 2020, Ho et al. 2020, Liu et al. 2020, Tan et al. 2022).
Dye degradation
Reactive dyes are manufactured and used at a rate of more than 8 × 104 tonnes annually because of their chemical stability and adaptability. Dyes are employed in textile dying as well as in tattoos, cosmetics, printing, and consumer goods. Nevertheless, once the dyes are discharged into the environment due to their endurance, contamination results. The most hazardous pollutants are synthetic textile dyes, which contaminate wastewater as part of industrial effluents. Bioremediation is more affordable and environment-friendly in comparison to chemical and physical decomposition methods. A total of 20 different types of textile dyes were previously discovered to be decolored by A. calcoaceticus NCIM 2890 (Wadhwani et al. 2018). Numerous other Acinetobacter species have demonstrated their effectiveness in bioremediation and decolorization of pollutant dyes. Acinetobacter pittii, for instance, has the ability to break down methylene blue. Within a few days of incubation, the organism showed more than 70% decolorization of the contaminated effluent as well as methylene blue degradation (Ogunlaja et al. 2020). A similar investigation on A. baumannii by Unnikrishnan and group in 2018 demonstrated above 85% decolorization in reactive red and over 90% degradation rate in dyes like Congo red and gentian violet within three days of incubation (Unnikrishnan et al. 2018). Recent research has shown that A. haemolyticus may degrade dyes like methylene green, basic violet, and acid blue with an efficiency of more than 75% (Hossain et al. 2022).
Plant-based applications
Many species of the genus Acintobacter are known to be involved in phytostimulation based on the production of hormones that promote plant growth as well as the solubilization of phosphate. Acinetobacter calcoaceticus has the ability to stimulate plant growth and metabolism via a positive effect on plant-produced abscisic acid and gibberellic acid (GA), amino acids, and crude protein, indicating a wider application as a biofertilizer for increased crop production and environment friendly farming practices (Gowtham et al. 2022). Acinetobacter calcoaceticus SE370 is a unique GA producer since it secretes ten different GAs into its environment, including a higher concentration of bioactive GA1, GA3, and GA4 (Pirog et al. 2021). Acinetobacter calcoaceticus has been found to colonize on plant surfaces and boost the chlorophyll content of both monocot and dicot plants. Similarly, A. junii can dissolve phosphate and also produces ammonia, indole acetic acid, GA, and hydrogen cyanide, all of which promote plant growth. Arbuscular mycorrhiza, together with A. junii, act as effective biofertilizers and promote the growth of tomato and bell pepper plants. An increase in absorbent surface area improves nutrient absorption, which in turn has improved plant development (Raimi et al. 2020). Additionally, A. rhizosphaerae strain BIHB 723 stimulates plant growth by solubilization of phosphate (Faria et al. 2021). Some Acinetobacter strains indirectly promote plant growth by suppressing the growth of phytopathogenic microorganisms, including Phytophthora capsici and Ralstonia solanacearum (Volynchikova et al. 2022). A recent study revealed that Acinetobacter sp. strain BRSI56 and ACRH80 subsequently reduce antioxidative stress in maize plant growing in hydrocarbon-contaminated environment (Ummara et al. 2022). Various plant-based applications of Acinetobacter species have been depicted in Fig. 4.
Use as bioreporters
Bacterial whole-cell bioreporters are live microorganisms that have been genetically modified to create signals in response to stress or certain substances, allowing for the quick and accurate identification of the bioavailable fractions in samples (Ali et al. 2021). Only small number of bacteria, like E. coli including certain species of Acinetobacter, might be utilized as bioreporters. Engineered A. baylyi ADP1 and ADPWH-alk can emulsify mineral and crude oils into oil droplets at the microlevel and cling to the oil-water interface. ADPWH-alk is able to overcome the limited solubility and accessibility of alkanes and therefore can easily detect oil spills in water and soil. The genotoxicity of phenolic chemicals in groundwater can be assessed using the bioluminescent bioreporter strain A. baylyi ADPWH-recA, which is capable of semi-quantitatively detecting genotoxic substances like mitomycin C and heavy metals (wu et al. 2023). In a contaminated environment where an E. coli-based reporter might not survive, Tetracycline can be detected by A. oleivorans strains that express bioreporters (Jiang and Song 2021). A report in 2021 suggested A. baylyi ADPWH recA as a potential bioreporter for detecting the effect of heavy metals like lead and cadmium on a contaminated environment (Li et al. 2021).
Future directions
The genus Acinetobacter contains more than 86 named species. The rise in the frequency and severity of infections, along with their multi-drug resistance ability, in the recent decade has been a big threat to mankind. Acinetobacter species are increasingly accumulating resistance mechanisms to regularly used group of antibiotics, including β-lactams, cephalosporin, carbapenem, and many more, which has continuously pressurized the scientific community to search for a potential therapeutic candidate against them. To address the rise in MDR Acinetobacter species, it is crucial to keep developing new antimicrobials and combining them with more established ones. Similarly, in order to guarantee the efficacy of future antimicrobial treatment, investigation of protocols to decrease incorrect prescribing and overuse of antimicrobial medicines is equally crucial (Lopez et al. 2019, Talebi et al. 2019).
The presence of antibiotic resistance genes in environmental species and their evolution as pathogens in humans and some vertebrates continue to attract the interest of researchers. However, their applications in biotechnology and bioremediation cannot be undermined. Very few bacteria, including some species of Acinetobacter and E. coli, can be used as bioreporters (Devi 2023). The combined impacts of heavy metals like Cd, Pb, and Cu have been evaluated using the luminescent bioreporter [Jiang et al 2021], A. baylyi ADPWH recA, and a suitable bioremediation technique has been suggested (Li et al. 2021). Therefore, it is important to investigate other Acinetobacter species as potential bioreporters. Similarly, numerous members of the genus have the ability to degrade crude oil and its byproducts, such as diesel, phenols, and other hydrocarbons. This ability can be further investigated by co-cultivating with fungi like Scedosporium, which might accelerate the degradation of these metabolites (Atakpa et al. 2022).
The development of an efficient method to track biomanufacturing productivity is crucial for enhancing pertinent bioprocesses. Fluorescent labeling of dicysteine-tagged peptide-like compounds can be used to monitor and optimize bioproduction in bacterial genera like Acinetobacter (Zheng et al. 2022). In Acinetobacter cultures, a high growth rate is typically attained by optimizing growth conditions. However, the maximum growth rate that can be achieved on the employed carbon source is constrained by Acinetobacter’s metabolism. This restriction can be overcome by metabolic engineering to commercialize more and more metabolites like lipase and wax esters (Ko et al. 2020). Numerous species in this genus have been linked to phytostimulation and solubilizing phosphate. Species like A. calcoaceticus SJ19 can greatly suppress mycelial growth in many species of fungi and therefore can be employed as bio-fungicides (Das et al. 2018, Dong et al. 2019). The nitrogen fixing capability (Ke et al. 2022), ability to attract insects for pollination, enhanced pollen bursting, and germination make the concerned species attractive candidates for further investigation in this direction (Christensen et al. 2021). The current review aims to strengthen the field by providing updated information on more than 50 species in the genus. A comprehensive compilation such as this would facilitate research on pathogens and non-pathogens, understanding their relevance with environment, and open avenues to utilize the ecologically important members for establishment of sustainable cities and communities.
Acknowledgement
S.G. and U.D. acknowledge Lovely Professional University, India for providing the necessary infrastructure facility. K.P. would like to thank DAV University, India for the infrastructure support.
Conflict of interest
There is no conflict of interest related to the present study. All the authors are agreed to submit the paper in this journal. No part of the said manuscript in any form is published or submitted to any other journals.
Author contributions
Ujwal Dahal (Data curation, Formal analysis, Resources, Validation, Visualization, Writing – original draft, Writing – review & editing), Karan Paul (Data curation, Investigation, Resources, Validation, Writing – review & editing), and Shelly Gupta (Conceptualization, Data curation, Formal analysis, Investigation, Resources, Supervision, Validation, Visualization, Writing – original draft, Writing – review & editing)
Data Availability
The data underlying this article are available in the article.