-
PDF
- Split View
-
Views
-
Cite
Cite
Shivaghami Shamugam, Michael A Kertesz, Bacterial interactions with the mycelium of the cultivated edible mushrooms Agaricus bisporus and Pleurotus ostreatus, Journal of Applied Microbiology, Volume 134, Issue 1, January 2023, lxac018, https://doi.org/10.1093/jambio/lxac018
- Share Icon Share
Abstract
The cultivated edible mushrooms Agaricus bisporus and Pleurotus ostreatus are valuable food crops and an important source of human nutrition. Agaricus bisporus is the dominant cultivated species in the western hemisphere and in Australia, while in Asian countries P. ostreatus is more prevalent. These two mushroom species are grown on fermented-pasteurized substrates, and bacteria and fungi play an important role in converting feedstocks into a selective medium for the mushroom mycelium. The mushrooms are usually introduced to the substrate as grain spawn, and the actively growing hyphae form a range of direct interactions with the diverse bacterial community in the substrate. Of these interactions, the most well studied is the removal of inhibitory volatile C8 compounds and ethylene by pseudomonads, which promotes mycelium growth and stimulates primordia formation of both A. bisporus and P. ostreatus. Bacterial biomass in the substrate is a significant nutrition source for the A. bisporus mycelium, both directly through bacteriolytic enzymes produced by A. bisporus, and indirectly through the action of extracellular bacterial enzymes, but this is less well studied for P. ostreatus. Apart from their role as a food source for the growing mycelium, bacteria also form extensive interactions with the mycelium of A. bisporus and P. ostreatus, by means other than those of the removal of inhibitory compounds. Although several of these interactions have been observed to promote mycelial growth, the proposed mechanisms of growth promotion by specific bacterial strains remain largely uncertain, and at times conflicting. Bacterial interactions also elicit varying growth-inhibitory responses from A. bisporus and P. ostreatus. This review explores characterized interactions involving bacteria and A. bisporus, and to a lesser degree P.ostreatus, and whilst doing so identifies existing research gaps and emphasizes directions for future work.
Introduction
Cultivated edible mushrooms are a significant source of nutrition and an economically important commodity in many regions of the world, but particularly in Asian countries such as China and Korea (Chang and Wasser 2017, Royse et al. 2017). Two genera, Agaricus and Pleurotus, together represent approximately a third of the world's mushroom supply (Royse et al. 2017). The button mushroom Agaricus bisporus is the most widely cultivated species in Europe, America, and Australia (Sonnenberg et al. 2011), but is also widely grown in China, which produces more than half of the global Agaricus supply (Royse et al. 2017). Pleurotus (oyster mushroom) represents 19% of global mushroom production, with Pleurotus ostreatus being one of the two major cultivated species of this genus (Royse et al. 2017). Pleurotus is also mainly grown in China (87% of global production; Royse et al. 2017), though both Pleurotus and Agaricus production is overshadowed in China by mushrooms that use sawdust as growth substrate, including Lentinula(shiitake), Auricularia (wood ear), and Flammulina (enoki). Agaricus bisporus and P. ostreatus, by contrast, are mainly grown on straw-based substrates that are prepared by a fermentation–pasteurization process in which bacteria and fungi are crucial for converting feedstocks into a selective nutrient medium for mushroom growth, as well as for promoting mushroom mycelium growth and stimulating fructification (Kertesz and Thai 2018, Carrasco et al. 2020).
Bacteria are present at every step of A. bisporus and P. ostreatus production. A broad range of bacterial taxa is essential at three stages: production of the mushroom growth substrate; growth of the mushroom mycelium; and fruit body production. In recent years, most focus has been placed on understanding the bacterial community that is responsible for production of the mushroom compost, since these interact with Agaricus or Pleurotus mycelium during hyphal proliferation.
Commercial-scale production of A. bisporus compost takes place in three distinct stages: an initial thermophilic Phase I, pasteurization and conditioning in Phase II, and hyphal proliferation in Phase III (Fig. 1). The principal raw materials are wheat straw or stable bedding, poultry manure, and gypsum, and the initial bacterial community of the compost is composed of mesophilic species that are present in the raw materials (e.g. Acinetobacter, Solibacillus, Comamonas, Pseudomonas, and Sphingomonas), often supplemented by wetting with compost leachate, which contains high numbers of Thermus (Safianowicz et al. 2018). The first stage of composting is characterized by a rapid succession of dominant taxa, as these utilize readily accessible carbon sources, and the increase of compost temperature to around 80°C during Phase I then selects for thermophilic bacteria, with a high proportion of Firmicutes and Proteobacteria, followed by Bacteroidetes, Thermus, and to a lesser extent Actinobacteria (Straatsma et al. 2000, Thai et al. 2022). During Phase II, the compost is first pasteurized at 58–60°C in a closed tunnel and then conditioned at 48–51°C, with cooling to ∼25°C before addition of the mushroom spawn (Straatsma et al. 2000). The key roles of bacterial and fungal populations in Phase II compost are to incorporate the cellulose and hemicellulose from the feedstocks into microbial biomass, and to assimilate excess ammonia, which is toxic to A. bisporus mycelium (Kertesz and Thai 2018). The most abundant bacterial taxon is often Pseudoxanthomonas taiwanensis (Vieira and Pecchia 2018, Thai et al. 2022), though its role in this cellulose-rich environment is unclear, since it lacks cellulase activity (Du et al. 2015). At the end of Phase II, the microbial community in the compost is dominated by the cellulose-degrading ascomycete Mycothermus thermophilus and bacterial taxa including Bacillus spp., actinomycetes such as Thermopolyspora or Microbispora, and heterotrophic nitrifiers such as Chelatococcus or Pseudoxanthomonas. These organisms support the growth of the Agaricus mycelium during Phase III. Agaricus bisporus is introduced into the compost as grain spawn, and the mushroom mycelium utilizes microbial biomass as its main nutrient source to support mycelium growth during initial colonization of the substrate (Jurak et al. 2015). By the end of Phase III, A. bisporus has degraded most of the M. thermophilus biomass, as well as a significant portion of bacterial biomass (Vos et al. 2017, Thai et al. 2022).
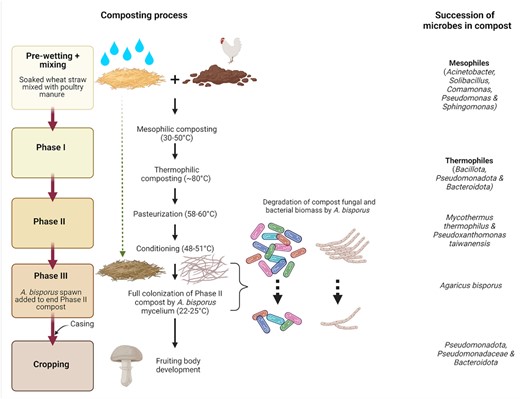
Overview of compost preparation for A. bisporus cultivation and the succession of dominant microbial taxa in the compost.
Substrate preparation for P. ostreatus is slightly simpler. Pleurotus ostreatus is cultivated on lignocellulosic substrates, most commonly chopped wheat straw or hardwood sawdust supplemented with nitrogen sources such as cereal bran, cottonseed hulls, or urea (Sánchez 2010). The mixed raw materials are subjected to partial composting followed by indoor pasteurization/conditioning before the addition of grain spawn (Bánfi et al. 2021). Proteobacteria (Pseudomonas and Sphingomonas) are dominant at the start of composting, but these are replaced by Bacillus, Pseudoxanthomonas, and Thermobispora by the end of the partial composting process (Vajna et al. 2012, Bánfi et al. 2021). The mature compost contains several actinobacterial genera, Thermusspp., and Firmicutes (Bacillus,Geobacillus,Thermobacillus,Ureibacillus) (Vajna et al. 2012). Colonization of the substrate by the P. ostreatus mycelium leads to a reduction of Thermus spp. and actinobacteria, possibly due to lower temperatures, and an increase in Bacillales and Halomonas spp., which demonstrate protective effects against competitor moulds (Bánfi et al. 2021).
As the mushroom mycelium grows through the prepared substrate, the hyphae interact with the bacteria in the compost, which colonize the hyphae, mobilize nutrients for hyphal uptake, and stimulate hyphal growth through removal of growth inhibitors. The shift in compost bacterial composition during proliferation of the A. bisporus mycelium strongly suggests that A. bisporus influences the bacterial population in the substrate through a host of underlying interactions with many different bacterial taxa (Carrasco et al. 2020). By contrast, the interactions that are important for fruit body production occur in the casing layer, in which the bacterial community is dominated by Pseudomonadaceae and the relative abundance of Pseudomonas spp. increases to very high levels during cropping (Carrasco et al. 2019, Vieira and Pecchia 2021). Most of the studies of these later interactions have therefore been carried out with Pseudomonas species, especially Pseudomonas putida and Pseudomonas fluorescens, and this is reflected in the analysis presented below.
The importance and mechanisms of bacterial–fungal interactions in the environment have been discussed in several excellent reviews (Frey-Klett et al. 2011, Deveau et al. 2018), and the interactions between mushrooms and bacteria that act as mushroom pathogens have also been discussed elsewhere (Largeteau and Savoie 2010, Savoie et al. 2016). This review focuses specifically on key aspects of the interactions between bacteria and the vegetative mycelium of A. bisporus and P. ostreatus, which are important for mycelial growth and primordia development (Fig. 2). These include (i) the bacteriolytic breakdown of bacterial biomass by the fungal hyphae, (ii) the attachment of bacteria to the mycelium mediated by chemotaxis, (iii) the promotion of hyphal growth through the secretion of extracellular enzymes and exudates by bacteria, or the stimulation of fungal enzyme activities, and (iv) bacterial–hyphal interactions that are critical for primordia development during fructification, mediating the removal of inhibitory compounds or modifying mycelial architecture to promote strand development. This allows us to identify existing research gaps and emphasize directions for future work that will increase our understanding of these essential interactions and increase the productivity of the mushroom industry.
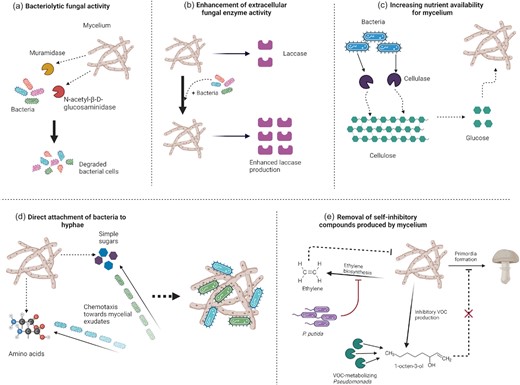
Interactions between bacteria and mushroom mycelium. (a) Bacteriolytic enzyme production by mycelium (β-N-acetylmuramidase and N-acetyl-β-d-glucosaminidase). (b) Enhancement of extracellular fungal ligninolytic enzyme (laccase) activity in the presence of bacteria. (c) Increased nutrient availability for the mycelium through bacterial cellulase secretion. (d) Direct attachment of bacteria to the mycelium through chemotaxis towards hyphal exudates (sugars and amino acids). (e) Removal of self-inhibitory compounds produced by the mycelium: (i) inhibition of the ethylene biosynthetic pathway by Ps. putida relieves mycelium growth inhibition by ethylene and (ii) pseudomonads metabolize the volatile C8 compound, 1-octen-3-ol, which inhibits primordia formation.
Fungal bacteriolytic activity during growth of the mushroom mycelium
Bacterial biomass in the compost represents a major nutrient source for mycelial growth of both Agaricus and Pleurotus. This is reflected in the 4-fold decrease in bacterial biomass observed during proliferation of A. bisporus (Vos et al. 2017), and a 5–10-fold decrease in bacterial 16S rRNA levels during growth of P. ostreatus (Bánfi et al. 2021). Pleurotus ostreatus hyphae have been shown to actively penetrate bacterial colonies of Ps. fluorescens in vitro, followed by profuse proliferation of short hyphae and lysis of the colonies (Tsuneda and Thorn 1995). In similar studies, the mycelium of A. bisporus degraded both living and dead Bacillus subtilis cells by secreting extracellular enzymes to degrade the peptidoglycan cell wall of bacteria (Fermor and Wood 1981, Fermor et al. 1991). This ability to release essential nutrients from bacterial biomass is one of the proposed reasons for the selective growth in compost of A. bisporus over competitor microorganisms such as green moulds, which rely on the availability of free nutrients within the compost (Fermor et al. 1991). In an in vitro setting, the cellular components of bacteria provide a sufficient source of carbon and nitrogen for mycelium growth, and are assimilated more efficiently than cellulose (Fermor et al. 1991). The two key extracellular bacteriolytic enzymes produced by A. bisporus mycelium are β-N-acetylmuramidase and N-acetyl-β-d-glucosaminidase (NAG) (Grant et al. 1984, Lincoln et al. 1997). Muramidase is a glycoside hydrolase that targets muramyl glycosidic linkages unique to peptidoglycan, and is the primary bacteriolytic enzyme produced by A. bisporus mycelium (Grant et al. 1984). Maximum activity of β-N-acetylmuramidase is induced in the presence of bacterial cells and enzyme production is repressed by addition of readily available carbon sources such as glucose and fructose (Grant et al. 1984, Lincoln et al. 1997). Although this repression is not a concern for Phase III where hardly any xylan and cellulose are degraded to free glucose (Jurak et al. 2015), it will be interesting to study whether the breakdown products of xylan and glucan have a similar repressive effect on muramidase activity during fruit body formation, when A. bisporus mycelium consumes ∼40% of total carbohydrates in compost (Kabel et al. 2017). If so, this would significantly reduce the ability of A. bisporus to continue obtaining any of its nutrition from bacterial biomass at this stage. In contrast to muramidase, fungal production of NAG is lower when A. bisporus mycelium is grown solely on bacterial cells as carbon source, and is increased when a combination of glucose and bacterial cells is utilized as the growth medium in vitro (Lincoln et al. 1997). NAG is important in recycling nitrogen from chitin and from the products of muramidase action (Rogers et al. 1979), and is therefore in common use as a soil health indicator (Acosta-Martinez et al. 2019). It seems likely that A. bisporus senses differences in the chemical composition of the substrate and secretes different concentrations of extracellular enzymes to balance its C and N requirements. This theory could explain why consistently higher levels of β-N-acetylmuramidase activity in A. bisporus has been found in the presence of lower numbers of bacteria (Grant et al. 1984, Lincoln et al. 1997). The A. bisporus mycelium also produces extracellular peptidases (though not peptidoglycan-degrading peptidases; Grant et al. 1984), but it is not known whether these attack the secreted muramidase, and they do not have an inhibitory effect on NAG activity (Fermor and Wood 1981, Savoie 1998). The possibility that bacteriolytic enzyme production by the mycelium is regulated through feedback mechanisms involving bacterial metabolites or by-products of bacterial activity should be explored to modify substrate composition for optimal bacterial degradation by A. bisporus. Identifying such relationships could improve the ability of A. bisporus to utilize bacterial biomass and potentially promote mycelial growth during spawn run.
The preferential degradation of certain bacterial taxa by the A. bisporus mycelium is also currently an area of uncertainty. Gram-positive bacterial populations increase and Gram-negative bacteria decrease during mycelial growth in mushroom compost (Vos et al. 2017), indicating either that the compost nutrient environment favours Gram-positive bacterial proliferation, or that the Agaricus mycelium preferentially degrades Gram-negative bacteria. The latter may be the case as A. bisporus secretes low levels of serine peptidase and metallopeptidase (Grant et al. 1984, Semenova et al. 2017), which could favour the degradation of thinner peptidoglycan cell walls. However, this does not explain the preferential mycelial degradation of both live and dead bacteria from different phyla, e.g. the preferred assimilation of Priestia megaterium (Firmicutes) over Alcaligenes faecalis (Betaproteobacteria), followed by Niallia circulans (Firmicutes) (Ahlawat et al. 2002). Stable isotope measurements of wood-degrading fungi related to Pleurotus have shown that the fungal hyphae specifically target diazotrophic bacteria for their nitrogen content (Weisshaupt et al. 2011), and similar methods could be used to examine whether differences in bacterial degradation in compost correspond to differences in nutrient assimilation by the mycelium. Bacterial taxa that are preferentially degraded could be investigated further for use as a compost bioinoculum.
Direct attachment of casing bacteria to mushroom mycelium
Because the hyphal density of the cultivated mushroom is at its peak during fructification, most of the bacterial species identified in recent cropping studies (Bánfi et al. 2021, Vieira and Pecchia 2021) are likely to be physically associated with the mushroom mycelium. This applies in principle to all the different bacterial taxa present, but the dominant bacterial species attached to A. bisporus hyphae in the casing layer are members of the fluorescent Pseudomonas group (Preece and Wong 1982). Pseudomonads comprise up to 80% of the bacteria in casing environments, including the basidiome-initiating bacteria Ps. putida and Ps. fluorescens and the mushroom pathogen Pseudomonas tolaasii (Preece and Wong 1982, Siyoum et al. 2016, Vieira and Pecchia 2021). Because these are easy to study in vitro, almost all research on these growth-promoting bacterial–hyphal interactions has concentrated on Ps. putida and Ps. fluorescens, and the next section therefore focuses very much on these two species. Further work is needed to extend these findings to other taxa that associate with Agaricus and Pleurotus hyphae in compost and casing.
Bacteria interact with A. bisporus mycelium by forming direct attachments to the hyphal surface (Masaphy et al. 1987, Grewal and Rainey 1991, Rainey 1991b). This is actively promoted by bacterial chemotaxis towards hyphal exudates of A. bisporus. These exudates consist mainly of simple sugars and free amino acids (Grewal and Rainey 1991, Rainey 1991b), with amino acids playing the major role in eliciting the chemotactic response in Ps. putida (Grewal and Rainey 1991). Fibrillar structures and amorphous material, possibly polysaccharide slime, aid in the self-association and anchoring of bacterial cells to the hyphal surface of A. bisporus (Rainey 1991b). The attachment of Ps. putida is probably enhanced through the addition of CaCl2, due to the importance of calcium as a bridging ion that ensures mechanical stability of extracellular polysaccharide matrices during biofilm formation (Körstgens et al. 2001). This should be confirmed through the addition of calcium compounds to the casing and measuring the effect on attachment rates of Ps. putida to mushroom hyphae.
It is interesting that several phenotypic variants of Ps. putida exhibit the ability to respond more quickly to mycelial exudates under nutrient-depleted conditions, and that this improves their attachment rates to A. bisporus mycelium (Rainey 1991b). This faster chemotactic behaviour results in greater attachment by these strains and highlights their potential as bioinoculants in the nutrient-depleted casing environment, where Ps. putida is generally recognized as important for removing growth-inhibiting compounds produced by the mycelium. This may also explain why a large proportion of bacteria that display mushroom growth-promoting abilities have been isolated from the casing layer and fruiting bodies, and not from the compost (Zarenejad et al. 2012, Xiang et al. 2017).
At present, it is unclear whether bacterial chemotaxis only occurs in response to hyphal exudates, or whether the bacterial cells also recognize gaseous products of the A. bisporus mycelium (Baars et al. 2020). Agaricus bisporus produces a range of volatile organic compounds (VOCs), most notably ethylene and several C8 compounds, which are metabolized by pseudomonads in the casing layer (Baars et al. 2020). Although several Pseudomonas species, including Ps. putida, exhibit positive chemotaxis towards ethylene in vitro (Kim et al. 2007), in the context of A. bisporus mycelium this is unlikely to be important, as several studies have found that the ethylene precursor 1-aminocyclopropane-1-carboxylate (ACC) serves as the primary chemoattractant for Ps. putida (Chen et al. 2013, Li et al. 2019). Chemotactic behaviour towards ACC is not likely to be limited exclusively to Ps. putida and other pseudomonads, as 20% of the total culturable bacterial population in casing soil has been found to produce ACC deaminase (AcdS) to utilize ACC as a nitrogen source (Chen et al. 2013). This highlights again the need for more work on bacterial species other than Ps. putida in studies of interactions with A. bisporus mycelium. During fruiting, ethylene levels in the casing are ∼10 times lower than in the compost (Baars et al. 2020). A possibility is that Pseudomonas strains in the casing layer might be phenotypic variants with increased levels of chemotaxis towards ACC and ACC degradation, thus interfering with ethylene production more efficiently than their counterparts in the compost. Future efforts directed at bacteria that form direct attachments to A. bisporus mycelium should focus on screening for new Pseudomonas strains as well as phenotypic variants that exhibit greater chemotactic ability towards ACC, as these bacteria could be important as inoculants for non-peat casing in the future.
Growth-promoting bacterial exudates and extracellular enzymes
Quite a few different bacterial species have been reported to simulate hyphal growth in compost, casing, and in vitro, but the most effective mycelium growth-promoting species is probably Ps. putida (Kertesz and Thai 2018). A primary mechanism by which this species promotes mycelium growth of A. bisporus is by relieving the inhibitory effects of ethylene (Fig. 3) (Chen et al. 2013, Zhang et al. 2016), which is produced from its precursor 1-aminocyclopropane-1-carboxylic acid (ACC) by the mycelium (Turner et al. 1975). After direct attachment to the hyphae, inoculation with strains of Ps. putida that can produce the enzyme ACC deaminase (AcdS) leads to a reduction in ethylene production by the mycelium and faster hyphal extension rates (Rainey 1991a, Cho et al. 2003). Interestingly, AcdS-deficient mutants are not only inhibitory to mycelium growth but also unable to attach to A. bisporus hyphae (Chen et al. 2013). ACC has been shown to be a strong chemoattractant for AcdS-producing Ps. putida (Li et al. 2019), which suggests the involvement of ACC/AcdS in bacterial chemotaxis and attachment to A. bisporus mycelium. This was confirmed by transformation of A. bisporus with the Pseudomonas acdSgene, as the transgenic hyphae not only showed enhanced extension rates but also reduced colonization by Pseudomonas cells (Zhang et al. 2019).
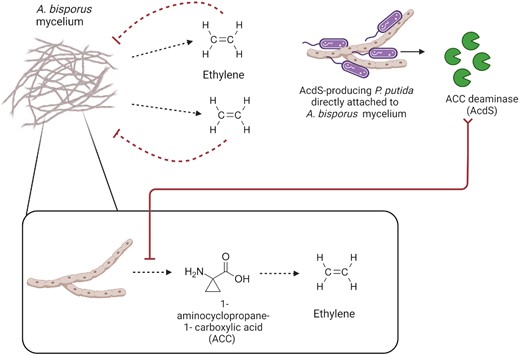
Reduced ethylene production by A. bisporus mycelium in the presence of Ps. putida strains that produce ACC deaminase (AcdS). Ethylene produced by A. bisporus mycelium is self-inhibitory to its growth. AcdS-producing Ps. putida cells co-cultured with A. bisporus mycelium are capable of direct attachment to A. bisporus hyphae and proceed to cleave ACC (immediate precursor to ethylene produced by the mycelium) through bacterial ACC deaminase (AcdS) activity.
Many strains of Ps. putida also possess the ability to secrete organic acids, which can solubilize inorganic phosphate and also increase the bioavailability of microelements for assimilation by A. bisporus hyphae (Zarenejad et al. 2012). However, it is important to note that phosphorus-solubilizing and nitrogen-fixing bacteria isolated from casing soil of Agaricus blazei neither increased the phosphorus and nitrogen content of mushrooms nor promoted mycelium growth (Young et al. 2013). Therefore, caution should be exercised when describing compost bacteria that exhibit these characteristics as ‘mushroom growth-promoting’, as these abilities may not necessarily benefit mycelium growth. In contrast, 50% of isolates that do promote mycelium growth secreted cellulase (Young et al. 2013). The effect of cellulase secretion by these bacteria on mycelial growth promotion has not yet been directly confirmed, since A. bisporus is of course able to degrade cellulose independently (Chow et al. 1994). It is possible that bacterial cellulase could aid in degrading cellulose and hemicellulose in the substrate to increase carbon availability for the mycelium, but cellulase activity may also simply select for bacteria that are able to survive well in compost and promote mycelial growth by other means.
Bacterial growth promotion is also known for P. ostreatus. Glutamicibacter arilaitensis adheres to the mycelial surface of P. ostreatus and exhibits AcdS activity similar to Ps. putida (Kumari and Naraian 2021), while Bradyrhizobium japonicum is thought to promote mycelium growth by nitrogen fixation (Zhu et al. 2013). The direct attachment of rhizobia to P. ostreatus mycelium has not yet been confirmed, but rhizobia are known to attach to hyphae of, e.g. arbuscular mycorrhizal fungi (AMF) through the production of extracellular polysaccharides (Bianciotto et al. 2001). Several mechanisms have been proposed to explain growth promotion of A. bisporus and P. ostreatus mycelium by bacteria, but these have been derived mostly from screening studies that identified growth-promoting bacteria based on cellular characteristics that have not been well studied directly in relation to mushroom mycelium growth in vitro or in situ.
It is interesting to note that in P. ostreatus, bacterial isolates from fruiting bodies exhibited lower enzymatic activities but promoted hyphal extension, whereas bacterial isolates from the vegetative mycelium produced higher levels of hydrolytic enzymes (peptidase and lipase) and inhibited hyphal growth (Suarez et al. 2020). Bacteria that promoted mycelium growth also produced chitinase, while inhibitory bacteria did not, which strongly suggests that growth-promoting bacteria may act by partial degradation of hyphal cell walls to obtain sugars and amino acids from the mushroom mycelium while providing other nutrients in exchange (Suarez et al. 2020). It is unclear what drives the difference in hydrolytic enzyme production between bacteria on fruiting bodies and vegetative mycelium as well as its effect on mycelium growth, and whether different nutrient environments elicit different enzymatic responses from bacteria.
There is also uncertainty in the growth-promoting role of bacterial indoleacetic acid (IAA) on P. ostreatus mycelium growth. Production of IAA by Ps. putida and Bacillus spp. compost isolates caused mycelial extension in P. ostreatus, whereas bacterial isolates from P. ostreatus fruiting bodies (predominantly Bacillus and Paenibacillus) that promoted mycelial growth did not produce IAA (Suarez et al. 2020). This once again emphasizes the variety of mycelium growth-promoting mechanisms, as well as phenotypic differences between bacteria from nutrient-depleted environments (casing and fruit body) compared with nutrient-rich environments (compost). It is interesting to note that strains of Ps. fluorescens isolated from fruiting bodies of P. ostreatus are classified as growth promoting due to bacterial IAA production (Xiang et al. 2017), while a Ps. fluorescens strain from A. bisporus casing soil had no effect on the rate of mycelium growth (Mohammad and Sabaa 2015). This suggests that the A. bisporus response to IAA may differ from that of P. ostreatus, but this does not appear to have been studied in detail.
Enhancement of fungal lignin degradation
Pleurotus ostreatus and A. bisporus are lignin-degrading basidiomycetes that produce extracellular phenol oxidase enzymes such as laccase and manganese peroxidase (MnP) that help break down the lignocellulosic substrate on which they are grown (Bonnen et al. 1994, Shah and Nerud 2002, Doddapaneni et al. 2013). Genes encoding laccases and MnP are strongly expressed in A. bisporus during mycelium proliferation in the compost, and less strongly later in mushroom growth (Patyshakuliyeva et al. 2015). However, these enzymes are not only useful for nutrition, but at least in P. ostreatus and related fungi, they play an important interactive role (Baldrian 2004). This has been best characterized for exposure of the P. ostreatus mycelium to other fungi (Baldrian 2004), but it is also known for A. bisporus (Flores et al. 2009) and indeed co-cultivation of different basidiomycetes is a standard way to enhance industrial production of a range of enzymes and other products (Yu et al. 2021). Exposure of the fungal mycelium to bacteria can also stimulate an increased ligninolytic response. Co-cultivation of P. ostreatus with mixed soil bacteria, Bacillus spp., Paenibacillus polymyxa, or even Escherichia coli leads to an increase in laccase activity in the fungal mycelium (Baldrian 2004, Velázquez-Cedeño et al. 2008). This appears to be a defence response, since although Bacillus or Polymyxa inhibits the growth of Trichoderma spp. and other antagonists of Pleurotus growth (Velázquez-Cedeño et al. 2008), they also appear to be fungistatic towards P. ostreatus itself and lead to reduced fungal colonization of substrate and inhibition of mycelium growth in vitro (Velázquez-Cedeño et al. 2004, 2008, Bánfi et al. 2021).
The relationship between mycelium growth inhibition and the ability of certain bacteria to induce ligninolytic enzyme activity may be linked to the production of reactive oxygen species (ROS). The production of hydrogen peroxide (H2O2) is positively correlated with the increase in vegetative mycelial biomass of P. ostreatus and A. bisporus (Savoie et al. 2007), and oxidation reactions catalysed by laccases and ligninolytic peroxidases such as MnP also lead to H2O2 production by the mycelium (Martínez et al. 2005, Perna et al. 2019). In higher plants, ethylene and ROS species such as H2O2 are closely linked, with H2O2 being found to induce the endogenous production of ethylene (Ishibashi et al. 2013, Cui et al. 2019). This suggests that bacteria that increase laccase and MnP activity in P. ostreatus may lead to increased H2O2 as a by-product, and this could produce positive feedback on the downstream production of growth-inhibiting ethylene by the mycelium. Further work is required to determine the exact mechanism of laccase and MnP regulation of P. ostreatus by bacteria, and to identify bacterial strains that enhance ligninolytic enzyme activity without inhibiting mycelial growth.
Interactions affecting primordia development
The presence of bacteria in the casing layer is essential for primordia development by A. bisporus, as demonstrated by its inability to form fruiting bodies in sterilized casing (Hayes et al. 1969). The stimulatory effect of bacteria on primordium formation by A. bisporus has been studied in most detail for Ps. putida (Hayes et al. 1969, Rainey et al. 1990), but a similar effect is seen with many other taxa, including strains of Alcaligenes,Bacillus,Rhodopseudomonas,Azotobacter, and Rhizobium, and even by an alga (Scenedesmus) and a yeast (Lipomyces) (Baars et al. 2020). However, the effect of these individual strains is relatively weak compared with the native microbiota on the mushroom beds, and even the most effective pseudomonad tested only affords about a third as many primordia as seen with unsterilized casing (Noble et al. 2009). The ability of A. bisporus to produce primordia in axenic casing material after addition of adsorptive materials such as activated charcoal suggests that these microbes stimulate primordia development in a similar manner, through the removal of inhibitory chemicals (Noble et al. 2003).
The mycelium of A. bisporus produces self-inhibitory volatile C8 compounds, with the most inhibitory VOC identified as 1-octen-3-ol (Noble et al. 2009), produced from linoleic acid by the mushroom hyphae (Husson et al. 2001). Another VOC inhibitory to hyphal growth is 2-ethyl-1-hexanol, and although the source of this compound has been attributed to rye grain substrate (Noble et al. 2009), it is worth noting that several ascomycetes are capable of producing this compound (Ezeonu et al. 1994, Pasanen et al. 1997). The possibility that M. thermophilus produces this inhibitory VOC is not known and should be confirmed. Both 1-octen-3-ol and 2-ethyl-1-hexanol are metabolized by pseudomonad populations in the casing, and this process enables primordia formation by A. bisporus (Noble et al. 2009). The importance of this interaction between Ps. putida and A. bisporus mycelium is highlighted by the improved yield obtained from inoculating the casing with Ps. putida strains that are highly tolerant to 1-octen-3-ol (Zarenejad et al. 2012). Addition or removal of volatile 1-octen-3-ol to the airstream leads to down- or upregulation of primordia formation (Noble et al. 2009, Eastwood et al. 2013). Future work should identify the genes associated with the ability to remove inhibitory C8 compounds, and conduct knockout experiments to confirm that induction of primordia formation is due to this mechanism.
The attachment of bacteria to the hyphae of A. bisporus in casing causes the disappearance of calcium oxalate crystals surrounding the hyphae (Masaphy et al. 1987). Calcium oxalate crystals are thought to interfere with strand formation, which precedes primordial formation in A. bisporus (Masaphy et al. 1987). Several Pseudomonas species metabolize and utilize oxalate either as a growth substrate (Blackmore and Quayle 1970) or as a siderophore (Dehner et al. 2010). It is possible that the induction of strand formation through oxalate removal by Ps. putida provides additional stimulation for A. bisporus to commit its resources towards reproductive growth, possibly through the modification of mycelium architecture. This in turn would explain the reduced vegetative mycelial biomass that is observed after inoculation with Ps. putida and other fluorescent pseudomonads (Reddy and Patrick 1990, Rainey 1991a).
As discussed earlier, the mycelium of A. bisporus produces ethylene, which inhibits mycelium growth (Turner et al. 1975, Zhang et al. 2016), and AcdS-producing Ps. putida that bind to the hyphae can reduce ethylene levels and induce primordium formation (Chen et al. 2013). However, there is some uncertainty about the mechanism involved here, since several studies that investigated the effect of increased or reduced ethylene levels on primordia formation have come to contradictory conclusions, with both enhanced and lowered primordia formation observed (Baars et al. 2020).
The stimulation of primordia formation of P. ostreatus by Ps. putida (Cho et al. 2003, 2008) may well be occurring by mechanisms similar to those that are important for A. bisporus; however, further confirmation and research into the bacteria–mycelium interactions that induce fruiting body formation are necessary for P. ostreatus.
Conclusions and outlook
Interactions between bacteria and the mushroom mycelium can greatly influence hyphal extension and primordia formation. This review makes clear that our current knowledge in this area is largely limited to the interactions between fluorescent pseudomonads and A. bisporus, with the majority of studies using Ps. putida as a model organism to study these interactions. Although Ps. putida has been consistently isolated from the casing, it is not always the dominant bacterial species present in the casing layer, and is sometimes not present at all in either casing or compost (Kertesz et al. 2016, Carrasco et al. 2019, Vieira and Pecchia 2021, Thai et al. 2022). The time taken for primordia formation is relatively consistent across casing layers with varying relative abundance of pseudomonads (Kertesz et al. 2016, Carrasco et al. 2020), and mushroom compost inocula developed on the basis of the growth-promoting abilities of Ps. putida may therefore not be effective in increasing yields. This indicates that other native bacterial populations are additionally responsible for removing inhibitory chemicals produced by A. bisporus mycelium and inducing primordia development and mycelium growth, and more work is needed to develop effective microbial consortia, rather than using individual strains.
Apart from Ps. putida, there is very little work at present that has identified and characterized growth-promoting or inhibitory interactions between P. ostreatus mycelium and bacterial populations present during cultivation, though some progress has been made in the area of wood degradation. Bacteriolytic activity by P. ostreatus mycelium is an area of uncertainty, and it is currently unknown whether bacterial biomass is as important a nutrition source for mycelium growth of P. ostreatus as it is for A. bisporus. The role of bacteria in regulating ligninolytic enzyme production of P. ostreatus is also an important bacteria–mycelium interaction that requires further investigation, as increased lignocellulose degradation can optimize substrate productivity and improve nutrient assimilation by the mycelium. A better understanding of the interactions between mushroom mycelium and bacteria during the cultivation process is critical in using microbes as indicators in defining and assessing the ‘quality’ of the compost. From an industrial standpoint, bacteria–mycelium interactions that promote both vegetative and reproductive growth have the potential to reduce the time needed for mycelium to colonize the substrate, enhance nutrient assimilation, and ultimately improve mushroom yields.
Acknowledgements
Work in our laboratory was supported by Hort Innovation, using the Australian Mushroom Research and Development Levy and contributions from the Australian Government. Hort Innovation is the grower-owned, not-for-profit research and development corporation for Australian horticulture. Figures were created with BioRender.com.
Conflict of interest
No conflict of interest declared.
Author contributions
S.S. planned and drafted the work. M.A.K. and S.S. wrote the manuscript.
Data availability
Data sharing not applicable to this review article as no datasets were generated or analysed during the current study.