-
PDF
- Split View
-
Views
-
Cite
Cite
Daniel Golparian, Susanne Jacobsson, Concerta L Holley, William M Shafer, Magnus Unemo, High-level in vitro resistance to gentamicin acquired in a stepwise manner in Neisseria gonorrhoeae, Journal of Antimicrobial Chemotherapy, Volume 78, Issue 7, July 2023, Pages 1769–1778, https://doi.org/10.1093/jac/dkad168
- Share Icon Share
Abstract
Gentamicin is used in several alternative treatments for gonorrhoea. Verified clinical Neisseria gonorrhoeae isolates with gentamicin resistance are mainly lacking and understanding the mechanisms for gonococcal gentamicin resistance is imperative. We selected gentamicin resistance in gonococci in vitro, identified the novel gentamicin-resistance mutations, and examined the biofitness of a high-level gentamicin-resistant mutant.
Low- and high-level gentamicin resistance was selected in WHO X (gentamicin MIC = 4 mg/L) on gentamicin-gradient agar plates. Selected mutants were whole-genome sequenced. Potential gentamicin-resistance fusA mutations were transformed into WT strains to verify their impact on gentamicin MICs. The biofitness of high-level gentamicin-resistant mutants was examined using a competitive assay in a hollow-fibre infection model.
WHO X mutants with gentamicin MICs of up to 128 mg/L were selected. Primarily selected fusA mutations were further investigated, and fusAR635L and fusAM520I + R635L were particularly interesting. Different mutations in fusA and ubiM were found in low-level gentamicin-resistant mutants, while fusAM520I was associated with high-level gentamicin resistance. Protein structure predictions showed that fusAM520I is located in domain IV of the elongation factor-G (EF-G). The high-level gentamicin-resistant WHO X mutant was outcompeted by the gentamicin-susceptible WHO X parental strain, suggesting lower biofitness.
We describe the first high-level gentamicin-resistant gonococcal isolate (MIC = 128 mg/L), which was selected in vitro through experimental evolution. The most substantial increases of the gentamicin MICs were caused by mutations in fusA (G1560A and G1904T encoding EF-G M520I and R635L, respectively) and ubiM (D186N). The high-level gentamicin-resistant N. gonorrhoeae mutant showed impaired biofitness.
Introduction
Gonorrhoea is an important public health concern as the second most common global bacterial sexually transmitted infection (STI) with 82.4 million new cases estimated among adults aged 15–49 years annually.1 The causative bacterium, Neisseria gonorrhoeae (gonococcus), has developed resistance to all introduced gonorrhoea therapies since the introduction of antibiotics.2–4 Current first-line treatment for uncomplicated gonorrhoea is ceftriaxone, an extended-spectrum cephalosporin (ESC), either in a high-dose monotherapy or given in combination with azithromycin.5–8 Since 2018, sporadic N. gonorrhoeae strains with resistance to ceftriaxone combined with high-level resistance to azithromycin causing treatment failures have been reported.9–11 Furthermore, international transmission of the ceftriaxone-resistant gonococcal FC428 clone has been verified since 2015.12–21 National and international antimicrobial surveillance reports have also documented increases in azithromycin resistance and expansions of azithromycin-resistant clones in the gonococcal population.22–25 This spread of N. gonorrhoeae strains resistant to ceftriaxone and/or azithromycin jeopardize the last remaining gonorrhoea therapies.
In 2017, the WHO published a list of 12 antimicrobial-resistant (AMR) pathogens including N. gonorrhoeae, which pose the greatest threat to human health,26 promoting research and development of new antimicrobials, but equally important, repurposing and optimized use of existing drugs. Aminoglycosides, such as gentamicin and tobramycin, in combination therapy or monotherapy remain active against several of the Gram-negative priority pathogens published by the WHO, e.g. Pseudomonas aeruginosa and Escherichia coli.27 Verified clinical N. gonorrhoeae isolates expressing in vitro resistance to gentamicin (MIC > 16 mg/L)28 have also been mainly lacking.28–30 Accordingly, several alternative treatment regimens for uncomplicated gonorrhoea recommended by the WHO, and in European, UK and US guidelines include gentamicin [240 mg intramuscularly (IM)] as an alternative treatment in combination with azithromycin single oral 2 g dose.5–8,22
In the advent of more widespread resistance to ceftriaxone, the use of gentamicin for treatment of gonorrhoea may rapidly increase. Consequently, it is imperative to understand how N. gonorrhoeae could potentially develop gentamicin resistance. In different bacterial species, the resistance determinants for gentamicin have involved enzymatic modifications,31 ineffective transport into the cell (although not fully understood),32 and rare ribosomal modifications causing resistance to streptomycin and spectinomycin (an aminocyclitol).33 A recent report described for the first time a resistance determinant for low-level resistance to gentamicin (MIC = 32 mg/L) in N. gonorrhoeae, i.e. SNPs in the fusA gene resulting in amino acid alterations, especially A563V, but also G564D and V651F in the elongation factor G (EF-G).34
Herein, we selected in vitro stepwise gentamicin-resistance determinants in N. gonorrhoeae, causing the gentamicin-susceptible WHO X reference strain35 to express high-level gentamicin resistance, identified the novel gentamicin-resistance mutations, and examined the biofitness of the WHO X mutant with high-level gentamicin resistance using competitive coculture in a dynamic hollow-fibre infection model (HFIM) for gonorrhoea.36
Materials and methods
N. gonorrhoeae isolate and antimicrobial susceptibility testing
The N. gonorrhoeae reference strain WHO X35 (gentamicin MIC of 4 mg/L) was cultured and subcultured once on a GCAGP agar plate (3.6% Difco GC Medium Base agar; BD Diagnostics, Sparks, MD, USA) supplemented with 1% haemoglobin (BD Diagnostics), 1% IsoVitaleX (BD Diagnostics) and 10% horse serum37 prior to 100 serial culture passages (subcultures) on gentamicin-gradient GCAGP agar plates. All incubations were performed in a 5% CO2-enriched humid atmosphere at 36 ± 1°C for 24 h. MICs (mg/L) of gentamicin, kanamycin, streptomycin, tobramycin, amikacin, ceftriaxone, cefixime, ciprofloxacin, ampicillin, tetracycline, erythromycin, spectinomycin and azithromycin were determined using Etest (bioMérieux, Marcy-l’Étoile, France), as previously described.37 The agar dilution method, in accordance with recommendations by CLSI,38 was used to further verify the MICs (mg/L) of gentamicin, kanamycin and amikacin. No gonococcal resistance breakpoints for gentamicin have been stated by EUCAST or CLSI. Consequently, in the present study a gentamicin-resistance breakpoint (MIC > 16 mg/L) suggested for N. gonorrhoeae in previous studies was used.28
In vitro selection for gentamicin resistance
GCAGP agar was prepared in two batches, of which one additionally included gentamicin. Gentamicin solution (10 mg/mL) in deionized water (Sigma–Aldrich, Saint Louis, USA) was used to prepare different gentamicin concentrations. Gentamicin-gradient agar plates were prepared as previously described.39 Briefly, the first layer of GCAGP agar including gentamicin (approximately 13 mL) was poured into a Petri dish placed on a slight slope and this layer was allowed to solidify prior to adding the second GCAGP agar layer without gentamicin (approximately 13 mL) when the Petri dish was placed on an even surface. The agar when solidified contained up to 8× the gentamicin MIC of WHO X.
The first culture passage was performed by suspending the WHO X reference strain in sterile saline solution to McFarland 0.5 and 100 µL of this bacterial solution was inoculated on the gentamicin-gradient GCAGP agar plate followed by incubation (36 ± 1°C, 5% CO2-enriched humid atmosphere, 24 h). A single colony growing at the highest gentamicin concentration was picked and subcultured on GCAGP agar plate without gentamicin (36 ± 1°C, 5% CO2-enriched humid atmosphere, 24 h). This type of culture passage was repeated and Etest for gentamicin was performed at passages 0, 2, 4, 6, 8, 10, 30, 40, 50, 60, 80 and 100, referred to as WHO X0–WHO X100. As a control, the WHO X reference strain was also serially cultured for 100 passages on a GCVIT agar plate [3.6% Difco GC Medium Base agar (BD) supplemented with 1% IsoVitaleX (BD)] without gentamicin.
WGS and analysis
DNA was extracted from 12 WHO X0–100 isolates using the automated QIAsymphony DSP Virus/Pathogen kit (QIAGEN, Hilden, Germany). Illumina DNA Prep (Illumina, Inc., San Diego, CA, USA) libraries were quality controlled using Qubit (Thermo Fisher Scientific, Waltham, MA, USA) and TapeStation (Agilent Technologies, Santa Clara, CA, USA), according to manufacturer instructions prior to library normalization. Illumina MiSeq reagent kit v2 (Illumina) was used to produce 250 paired-end reads with an average coverage of >100×.
All reads were quality controlled, trimmed (Phred quality score Q30), and assembled using a customized CLC Genomics Workbench (v22.0.1).3 Furthermore, a pipeline to generate a multiple sequence alignment, phylogenetic tree and additional quality control reports was performed using Nullarbor (https://github.com/tseemann/nullarbor) with WHO X (accession: NZ_LT592155.1) as reference with the following parameters; –ref NZ_LT592155.1.fasta –assembler spades –assembler-opt ‘–careful’ –taxoner kraken2. The multiple sequence alignment was used to compute pairwise distances between the WHO X reference genome35 and WHO X0–100 using MEGA (v11.0.10).40 The phylogenetic tree was visualized using Microreact.41 All raw sequence reads are available through the European Nucleotide Archive (project accession number: PRJEB56638).
Mutations found in the gentamicin-resistance selected isolates were also queried for in an in-house database with 33 306 publicly available gonococcal genomes (downloaded 31 January 2023) to investigate the occurrences in the gonococcal population, as previously described.42
Transformation of fusA
To examine whether the novel fusA mutations contributed to the high-level resistance to gentamicin in WHO X, the full-length fusA gene was PCR amplified using the primers fusA_F (CCACGACGACAGAACGTATT) and fusA_R (TTACGGGCTTCAGTTACAGC). The amplified product was transformed into WHO M35 and WHO X35 using spot transformation with 0.1 μg of purified fusA PCR product. Transformants with increased MICs of gentamicin were selected on gentamicin-containing GCVIT agar plates (16 mg/L). WGS, as described above, was performed to verify all fusA transformants.
Biofitness experiments using competitive coculture in an HFIM
To evaluate the biofitness of the WHO X mutant with high-level gentamicin resistance (WHO X100 with EF-G R635L and M520I substitutions) selected on the gentamicin-gradient GCAGP37 agar plates compared with the gentamicin-susceptible WHO X parent strain, competition experiments using coculture were performed in an HFIM, as earlier described.36,43 Briefly, bacteria were harvested from GCAGP agar plates and suspended in modified fastidious broth (mFB)36,43 to a quantity of ∼105–106 cfu/mL. Equal volumes (5 mL) of the suspensions of each strain were inoculated into the same HFIM cartridge. Aliquots (1 mL) were sampled at 24, 48, 72, 96, 120, 144 and 168 h, serially diluted in mFB and quantitatively plated on GCAGP agar plates and GCAGP agar plates containing 3×MIC of gentamicin, as previously described.36,43 Colony counts (log10 cfu/mL) were quantified after incubation for up to 72 h at 36°C in a humidified 5% CO2-enriched humid atmosphere using an automated colony counter (Scan 4000, Interscience, Saint-Nom-la-Bretèche, France). The competitive index (CI)36,43,44 was determined by dividing the ratio of the WHO X100 (EF-G R635L and M520I) mutant to WHO X parent strain at each timepoint with the ratio of the WHO X100 (EF-G R635L and M520I) mutant to WHO X parent strain in the initial inoculum.
Protein structure prediction
The protein structure of WHO X EF-G was predicted using the primary structure of fusA as input to AlphaFold (v2.1.0) (DeepMind, London, UK) executed using Colaboratory (Google Research, San Francisco, USA). It was analysed as a monomer and queried using the full database (https://github.com/deepmind/alphafold).45 Pfam was used to identify protein domains46 and CASTp was used to identify binding pockets.47 PyMOL was used to visualize the protein, amino acids and used for mutagenesis analysis (v2.0) (Schrödinger, LLC).
Results
Long-term in vitro gentamicin exposure causes high-level resistance in N. gonorrhoeae
The MICs of gentamicin for WHO X and several other aminoglycosides after serial culture passages (subcultures) on gentamicin-gradient agar plates are summarized in Table 1. As documented earlier,30 the gentamicin MIC determined using Etest is frequently approximately one gentamicin MIC doubling dilution lower compared with the agar dilution gentamicin MIC. The first 10 passages resulted in only minor increases in the antimicrobial MICs (one MIC doubling dilution) in the isolates WHO X0–10 (Table 1). For example, the Etest MIC of gentamicin only increased from 4 mg/L in WHO X0 to 8 mg/L in WHO X10. A more substantial increase in gentamicin MIC was documented after 30 passages (WHO X30) when low-level gentamicin resistance (MIC = 32 mg/L) and substantially increased MICs of also the additional aminoglycosides (MIC = 32 to >256 mg/L) were determined. The WHO X30 mutant appeared to be relatively stable in both growth and MICs of gentamicin and the additional aminoglycosides. Accordingly, no further increases in the MICs of gentamicin were recorded until after 100 serial culture passages (WHO X100), when high-level gentamicin resistance (MIC = 128 mg/L) and Etest MICs ≥ 256 mg/L of kanamycin, tobramycin and amikacin were identified. The MIC of streptomycin was also substantially increased, i.e. 32 mg/L. Notably, the WHO X MICs of ceftriaxone (4 mg/L), cefixime (8 mg/L), azithromycin (1 mg/L), ciprofloxacin (>32 mg/L), ampicillin (2 mg/L), tetracycline (2 mg/L), erythromycin (2 mg/L) and spectinomycin (16 mg/L) did not change during the 100 serial culture passages.
Changes in MICs (mg/L) of five aminoglycosides for N. gonorrhoeae reference strain WHO X35 during 100 serial culture passages (subcultures) on gentamicin-gradient agar plates
. | Etest MIC (agar dilution MIC), mg/La,b . | ||||
---|---|---|---|---|---|
Passage | Gentamicin | Kanamycin | Streptomycin | Tobramycin | Amikacin |
WHO X0 | 4 (8) | 16 (16) | 8 | 4 | 32 (128) |
WHO X2 | 4 (8) | 16 (32) | 16 | 8 | 64 (64) |
WHO X4 | 4 (16) | 16 (32) | 16 | 8 | 128 (128) |
WHO X6 | 4 (16) | 32 (32) | 16 | 8 | 64 (64) |
WHO X8 | 4 (16) | 32 (32) | 16 | 8 | 64 (64) |
WHO X10 | 8 (16) | 32 (32) | 16 | 8 | 64 (128) |
WHO X30 | 32 (64) | 128 (128) | 32 | 32 | >256 (256) |
WHO X40 | 32 (64) | >256 (128) | 32 | 64 | >256 (256) |
WHO X50 | 32 (64) | >256 (128) | 32 | 64 | >256 (256) |
WHO X60 | 32 (32) | >256 (128) | 32 | 64 | >256 (256) |
WHO X80 | 32 (64) | >256 (256) | 32 | 64 | >256 (256) |
WHO X100 | 128 (128) | >256 (512) | 32 | 256 | >256 (256) |
. | Etest MIC (agar dilution MIC), mg/La,b . | ||||
---|---|---|---|---|---|
Passage | Gentamicin | Kanamycin | Streptomycin | Tobramycin | Amikacin |
WHO X0 | 4 (8) | 16 (16) | 8 | 4 | 32 (128) |
WHO X2 | 4 (8) | 16 (32) | 16 | 8 | 64 (64) |
WHO X4 | 4 (16) | 16 (32) | 16 | 8 | 128 (128) |
WHO X6 | 4 (16) | 32 (32) | 16 | 8 | 64 (64) |
WHO X8 | 4 (16) | 32 (32) | 16 | 8 | 64 (64) |
WHO X10 | 8 (16) | 32 (32) | 16 | 8 | 64 (128) |
WHO X30 | 32 (64) | 128 (128) | 32 | 32 | >256 (256) |
WHO X40 | 32 (64) | >256 (128) | 32 | 64 | >256 (256) |
WHO X50 | 32 (64) | >256 (128) | 32 | 64 | >256 (256) |
WHO X60 | 32 (32) | >256 (128) | 32 | 64 | >256 (256) |
WHO X80 | 32 (64) | >256 (256) | 32 | 64 | >256 (256) |
WHO X100 | 128 (128) | >256 (512) | 32 | 256 | >256 (256) |
MICs (mg/L) were determined using both Etest and agar dilution method (in parentheses).
For in vitro selected mutations associated with increased aminoglycosides MICs, see Figure 1.
Changes in MICs (mg/L) of five aminoglycosides for N. gonorrhoeae reference strain WHO X35 during 100 serial culture passages (subcultures) on gentamicin-gradient agar plates
. | Etest MIC (agar dilution MIC), mg/La,b . | ||||
---|---|---|---|---|---|
Passage | Gentamicin | Kanamycin | Streptomycin | Tobramycin | Amikacin |
WHO X0 | 4 (8) | 16 (16) | 8 | 4 | 32 (128) |
WHO X2 | 4 (8) | 16 (32) | 16 | 8 | 64 (64) |
WHO X4 | 4 (16) | 16 (32) | 16 | 8 | 128 (128) |
WHO X6 | 4 (16) | 32 (32) | 16 | 8 | 64 (64) |
WHO X8 | 4 (16) | 32 (32) | 16 | 8 | 64 (64) |
WHO X10 | 8 (16) | 32 (32) | 16 | 8 | 64 (128) |
WHO X30 | 32 (64) | 128 (128) | 32 | 32 | >256 (256) |
WHO X40 | 32 (64) | >256 (128) | 32 | 64 | >256 (256) |
WHO X50 | 32 (64) | >256 (128) | 32 | 64 | >256 (256) |
WHO X60 | 32 (32) | >256 (128) | 32 | 64 | >256 (256) |
WHO X80 | 32 (64) | >256 (256) | 32 | 64 | >256 (256) |
WHO X100 | 128 (128) | >256 (512) | 32 | 256 | >256 (256) |
. | Etest MIC (agar dilution MIC), mg/La,b . | ||||
---|---|---|---|---|---|
Passage | Gentamicin | Kanamycin | Streptomycin | Tobramycin | Amikacin |
WHO X0 | 4 (8) | 16 (16) | 8 | 4 | 32 (128) |
WHO X2 | 4 (8) | 16 (32) | 16 | 8 | 64 (64) |
WHO X4 | 4 (16) | 16 (32) | 16 | 8 | 128 (128) |
WHO X6 | 4 (16) | 32 (32) | 16 | 8 | 64 (64) |
WHO X8 | 4 (16) | 32 (32) | 16 | 8 | 64 (64) |
WHO X10 | 8 (16) | 32 (32) | 16 | 8 | 64 (128) |
WHO X30 | 32 (64) | 128 (128) | 32 | 32 | >256 (256) |
WHO X40 | 32 (64) | >256 (128) | 32 | 64 | >256 (256) |
WHO X50 | 32 (64) | >256 (128) | 32 | 64 | >256 (256) |
WHO X60 | 32 (32) | >256 (128) | 32 | 64 | >256 (256) |
WHO X80 | 32 (64) | >256 (256) | 32 | 64 | >256 (256) |
WHO X100 | 128 (128) | >256 (512) | 32 | 256 | >256 (256) |
MICs (mg/L) were determined using both Etest and agar dilution method (in parentheses).
For in vitro selected mutations associated with increased aminoglycosides MICs, see Figure 1.
Mutations in several genes including fusA cause high-level gentamicin resistance
The results of the pairwise genome comparisons between WHO X and the 11 WHO X2–100 isolates from serial culture passages on gentamicin-gradient agar plates are summarized in Table 2. In total, 19 SNPs were found in the seven WHO X10–100 isolates. However, 9 of these 19 SNPs (all in opa and pilE genes) were also detected in the gentamicin-unexposed WHO X control strain, which also passed 100 serial culture passages. This gentamicin-unexposed strain did not show any increased MIC of gentamicin and, accordingly, these SNPs were considered as normal SNPs in repetitive regions of the gonococcal genome and excluded from further analysis. Interestingly, a stepwise accumulation of SNPs in the isolates with gentamicin exposure was observed and could be phylogenomically discerned (Figure 1). The mutations had been acquired stepwise in the following order: the pilE gene, followed by ubiM, lptD, pglD, opa, unknown membrane protein, pgsA, fusA and finally cysB. The mutations fusA G1560A (EF-G M520I) and ubiM D186N resulted in the most substantial increase in gentamicin MICs (Figure 1).
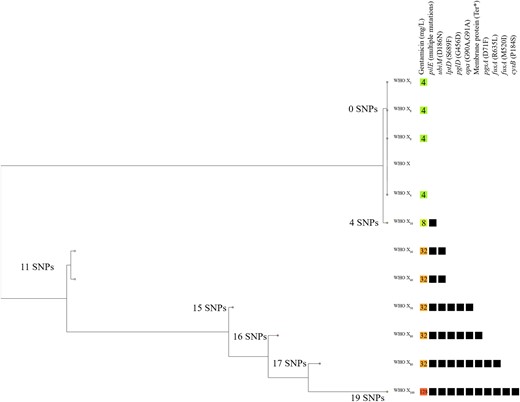
Phylogenomic tree of the gentamicin-susceptible N. gonorrhoeae WHO X35 parent strain and the in vitro gentamicin-exposed WHO X2–100 mutants, including the gentamicin-resistant WHO X30–100 mutants. The coloured boxes show the different MICs (mg/L) of gentamicin and the black boxes represent presence of mutations in the genes. This figure appears in colour in the online version of JAC and in black and white in the print version of JAC.
All SNPs (n = 19) selected by gentamicin exposure in the WHO X10–100 mutants
UniProt locus tag/gene . | Mutation (genetic alterations) . | Protein/function . | Function . |
---|---|---|---|
pglD | G1367A (G456D) | Protein-PII uridylyltransferase | Pilin glycosylation protein PglD |
pgsA | C211T (D71F) | CDP-diacylglycerol–glycerol-3-phosphate 3-phosphatidyltransferase | Phospholipid biosynthesis |
NGO_0974 | C1008A (premature stop-codon) | Membrane protein | Lysophospholipid transporter |
Opa | G90A, G91A (-25 and -26 opa mutation) | Opacity protein | Outer membrane protein |
pilE | A812C, C813G, T815C, T816A, G817T, A818C, G824A, C825A, G827A (N121T, F122S, D123S, S125K) | Fimbrial protein | Type IV major pilin protein PilE |
cysB | C550T (P184S) | Transcriptional regulator CysB | |
ubiM | G556A (D186N) | 5-demethoxyubiquinol-8 5-hydroxylase UbiM | Ubiquinone synthesis involved in electron transport and oxidative stress defence |
lptD | C2066T (S689F) | LOS-assembly protein | LPS synthesis |
fusA | G1560A, G1904T (M520I, R635L) | Elongation factor G | Central role in protein synthesis |
UniProt locus tag/gene . | Mutation (genetic alterations) . | Protein/function . | Function . |
---|---|---|---|
pglD | G1367A (G456D) | Protein-PII uridylyltransferase | Pilin glycosylation protein PglD |
pgsA | C211T (D71F) | CDP-diacylglycerol–glycerol-3-phosphate 3-phosphatidyltransferase | Phospholipid biosynthesis |
NGO_0974 | C1008A (premature stop-codon) | Membrane protein | Lysophospholipid transporter |
Opa | G90A, G91A (-25 and -26 opa mutation) | Opacity protein | Outer membrane protein |
pilE | A812C, C813G, T815C, T816A, G817T, A818C, G824A, C825A, G827A (N121T, F122S, D123S, S125K) | Fimbrial protein | Type IV major pilin protein PilE |
cysB | C550T (P184S) | Transcriptional regulator CysB | |
ubiM | G556A (D186N) | 5-demethoxyubiquinol-8 5-hydroxylase UbiM | Ubiquinone synthesis involved in electron transport and oxidative stress defence |
lptD | C2066T (S689F) | LOS-assembly protein | LPS synthesis |
fusA | G1560A, G1904T (M520I, R635L) | Elongation factor G | Central role in protein synthesis |
All SNPs (n = 19) selected by gentamicin exposure in the WHO X10–100 mutants
UniProt locus tag/gene . | Mutation (genetic alterations) . | Protein/function . | Function . |
---|---|---|---|
pglD | G1367A (G456D) | Protein-PII uridylyltransferase | Pilin glycosylation protein PglD |
pgsA | C211T (D71F) | CDP-diacylglycerol–glycerol-3-phosphate 3-phosphatidyltransferase | Phospholipid biosynthesis |
NGO_0974 | C1008A (premature stop-codon) | Membrane protein | Lysophospholipid transporter |
Opa | G90A, G91A (-25 and -26 opa mutation) | Opacity protein | Outer membrane protein |
pilE | A812C, C813G, T815C, T816A, G817T, A818C, G824A, C825A, G827A (N121T, F122S, D123S, S125K) | Fimbrial protein | Type IV major pilin protein PilE |
cysB | C550T (P184S) | Transcriptional regulator CysB | |
ubiM | G556A (D186N) | 5-demethoxyubiquinol-8 5-hydroxylase UbiM | Ubiquinone synthesis involved in electron transport and oxidative stress defence |
lptD | C2066T (S689F) | LOS-assembly protein | LPS synthesis |
fusA | G1560A, G1904T (M520I, R635L) | Elongation factor G | Central role in protein synthesis |
UniProt locus tag/gene . | Mutation (genetic alterations) . | Protein/function . | Function . |
---|---|---|---|
pglD | G1367A (G456D) | Protein-PII uridylyltransferase | Pilin glycosylation protein PglD |
pgsA | C211T (D71F) | CDP-diacylglycerol–glycerol-3-phosphate 3-phosphatidyltransferase | Phospholipid biosynthesis |
NGO_0974 | C1008A (premature stop-codon) | Membrane protein | Lysophospholipid transporter |
Opa | G90A, G91A (-25 and -26 opa mutation) | Opacity protein | Outer membrane protein |
pilE | A812C, C813G, T815C, T816A, G817T, A818C, G824A, C825A, G827A (N121T, F122S, D123S, S125K) | Fimbrial protein | Type IV major pilin protein PilE |
cysB | C550T (P184S) | Transcriptional regulator CysB | |
ubiM | G556A (D186N) | 5-demethoxyubiquinol-8 5-hydroxylase UbiM | Ubiquinone synthesis involved in electron transport and oxidative stress defence |
lptD | C2066T (S689F) | LOS-assembly protein | LPS synthesis |
fusA | G1560A, G1904T (M520I, R635L) | Elongation factor G | Central role in protein synthesis |
Transformation experiments were focused on fusA because an EF-G A563V substitution was recently shown to cause low-level resistance to gentamicin (MIC = 32 mg/L) in N. gonorrhoeae34 and the two EF-G amino acid substitutions selected in the present study appeared to substantially increase the MICs of gentamicin. Accordingly, the impact of these identified amino acid alterations in EF-G on the MICs of gentamicin and other aminoglycosides was examined, i.e. by transforming R635L alone (fusA G1904T) and R635L (fusA G1904T) in combination with M520I (fusA G1560A) to the WHO M and WHO X reference strains.35 The MICs of gentamicin and the other aminoglycosides in the transformants are summarized in Table 3. Briefly, the R635L transformants, i.e. WHO MR635L and WHO XR635L, showed a 2- to 3-fold increase in the gentamicin MIC (to 16 and 32 mg/L, respectively) as well as in the MICs of kanamycin, tobramycin, amikacin and streptomycin. The M520I + R635L transformants were both resistant to gentamicin (MIC = 32 mg/L) and showed high MICs (≥256 mg/L) of kanamycin, amikacin and streptomycin (Table 3).
. | Etest (mg/L) . | ||||
---|---|---|---|---|---|
Isolate . | Gentamicin . | Kanamycin . | Amikacin . | Tobramycin . | Streptomycin . |
WHO M | 4 | 16 | 32 | 8 | 16 |
WHO MR635L | 16 | 128 | 256 | 32 | 128 |
WHO MM520I + R635L | 32 | 256 | 256 | 32 | 256 |
WHO X | 4 | 16 | 32 | 4 | 16 |
WHO XR635L | 32 | 128 | 128 | 32 | 128 |
WHO XM520I + R635L | 32 | >256 | >256 | 32 | >256 |
. | Etest (mg/L) . | ||||
---|---|---|---|---|---|
Isolate . | Gentamicin . | Kanamycin . | Amikacin . | Tobramycin . | Streptomycin . |
WHO M | 4 | 16 | 32 | 8 | 16 |
WHO MR635L | 16 | 128 | 256 | 32 | 128 |
WHO MM520I + R635L | 32 | 256 | 256 | 32 | 256 |
WHO X | 4 | 16 | 32 | 4 | 16 |
WHO XR635L | 32 | 128 | 128 | 32 | 128 |
WHO XM520I + R635L | 32 | >256 | >256 | 32 | >256 |
. | Etest (mg/L) . | ||||
---|---|---|---|---|---|
Isolate . | Gentamicin . | Kanamycin . | Amikacin . | Tobramycin . | Streptomycin . |
WHO M | 4 | 16 | 32 | 8 | 16 |
WHO MR635L | 16 | 128 | 256 | 32 | 128 |
WHO MM520I + R635L | 32 | 256 | 256 | 32 | 256 |
WHO X | 4 | 16 | 32 | 4 | 16 |
WHO XR635L | 32 | 128 | 128 | 32 | 128 |
WHO XM520I + R635L | 32 | >256 | >256 | 32 | >256 |
. | Etest (mg/L) . | ||||
---|---|---|---|---|---|
Isolate . | Gentamicin . | Kanamycin . | Amikacin . | Tobramycin . | Streptomycin . |
WHO M | 4 | 16 | 32 | 8 | 16 |
WHO MR635L | 16 | 128 | 256 | 32 | 128 |
WHO MM520I + R635L | 32 | 256 | 256 | 32 | 256 |
WHO X | 4 | 16 | 32 | 4 | 16 |
WHO XR635L | 32 | 128 | 128 | 32 | 128 |
WHO XM520I + R635L | 32 | >256 | >256 | 32 | >256 |
The genes (locus_tag) cysB (C7S01_RS08790), fusA (C7S01_RS1176), lptD (C7S01_RS10025), pglD (C7S01_RS00555), pgsA (C7S01_RS01250) and ubiM (C7S01_RS09765) were queried among 33 306 publicly available gonococcal genomes for the in vitro selected mutations associated with gentamicin resistance (Table 2). None of the mutations selected in cysB, lptD, pglD, pgsA and ubiM were found. However, two isolates from Ontario, Canada contained a fusA R635G mutation,48 and these isolates would be of interest to investigate further.
In vitro selected high-level gentamicin-resistant N. gonorrhoeae isolates have impaired biofitness
In general, compared with colonies of the gentamicin-susceptible WHO X35 parent strain the colonies of the high-level gentamicin-resistant WHO X100 mutant were substantially smaller and slow-growing on the GCAGP agar plates, i.e. grew as SCVs. The WHO X100 mutant and WHO X parent strain were subsequently cultured in separate HFIM cartridges as well as cocultured in the same HFIM cartridge for 7 days to evaluate if the in vitro selected high-level gentamicin-resistant WHO X100 mutant showed any impaired bacterial growth and biofitness. The growth of WHO X was maintained at approximately 1010 cfu/mL during the 7 day experiment, while the growth of the WHO X100 mutant was substantially lower from 24 h and to the end of the 7 day experiment (Figure 2a). The CI further verified that the WHO X parent strain outcompeted the high-level gentamicin-resistant WHO X100 mutant. Accordingly, despite the WHO X100 mutant starting to recover in growth after 96 h, which may indicate emergence of compensatory mutations restoring biofitness, it clearly suffered from impaired biofitness compared to the WHO X parent strain (Figure 2b).
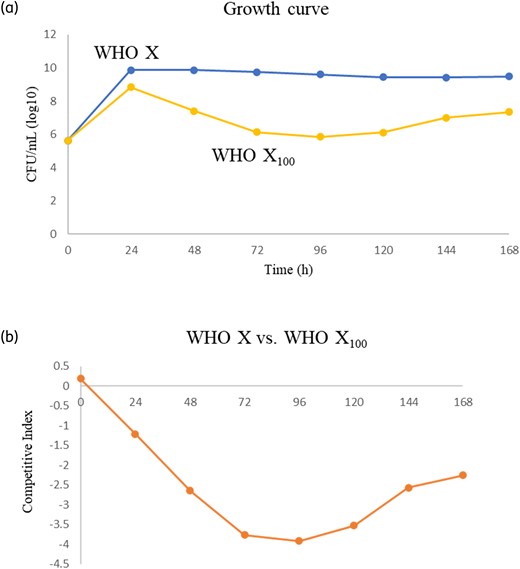
Growth curves for WHO X35 parent strain and the in vitro selected high-level gentamicin-resistant WHO X100 mutant and competition experiment using coculture in an HFIM for gonorrhoea.36,43 (a) shows the cfu/mL of WHO X compared with WHO X100 during growth for 7 days and (b) displays the CI36,43 during coculture for 7 days, which illustrates how WHO X is outcompeting WHO X100 (especially during the first 4 days). A negative value indicates that the in vitro selected high-level gentamicin-resistant WHO X100 mutant has a lower biofitness compared with the gentamicin-susceptible WHO X35 parent strain. This figure appears in colour in the online version of JAC and in black and white in the print version of JAC.
Low- and high-level gentamicin resistance is associated with alterations in domain IV and V in EF-G
The 3D structure of EF-G has five domains (Figure 3). The amino acid substitutions M520I and R635L are found in domains IV and V, respectively. EF-G M520 primarily interacts with V497 and mutagenesis analysis shows that the M520I amino acid substitution in domain IV has a similar interaction with V497 and both methionine (M) and isoleucine (I) are part of the aspartate family of amino acids. Interestingly, the M520I substitution occurs in the ribosomal A-site, which is essential for EF-G as well as for gentamicin. Similarly, R635 in domain V interacts with L631 and N632 via two hydrogen bonds, while the amino acid substitution R635L results in a loss of one hydrogen bond and only interacts with L631 (Figure S1, available as Supplementary data at JAC Online). Both M520I and R635L could potentially cause conformational changes in the protein structure indicated by the number of steric clashes that the mutated amino acids present (Figure S2). Furthermore, a single binding pocket was identified between domain III and domain V where ribosomal protein S12 directly interacts with EF-G, a critical element for the stabilization of EF-G while bound to the ribosome49,50 (Figure S3).
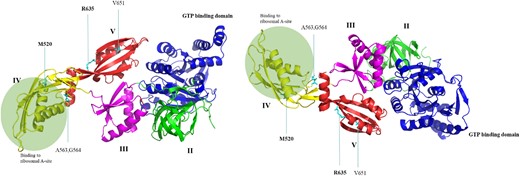
Two views of the same elongated EF-G protein structure predicted using AlphaFold. The domains II–V as well as the GTP binding domain are coloured. The amino acid substitutions causing low-level gentamicin resistance in a previous report34 are pointed out and amino acids found to be altered in the present study are in bold. This figure appears in colour in the online version of JAC and in black and white in the print version of JAC.
Discussion
In the absence of a gonococcal vaccine, management and control of gonorrhoea rely on effective, affordable and accessible antimicrobial treatment. As ceftriaxone- and azithromycin-resistant clones are spreading internationally and the accumulation of AMR determinants in N. gonorrhoeae to these current as well as previous first-line empirical treatments for gonorrhoea continues,2–4,9–25 there is an urgent need for novel antimicrobials and/or repurposed and optimized use of existing antimicrobials. Gentamicin, recommended in combination with azithromycin as an alternative treatment for gonorrhoea,5–8,22 might become much more frequently used and verified clinical N. gonorrhoeae isolates with in vitro resistance to gentamicin (>16 mg/L) have also been mainly lacking. In fact, the in vitro susceptibility to gentamicin has remained remarkably stable in the global gonococcal population with a narrow MIC range (4–8 mg/L) over time.28–30,51 In Malawi, gentamicin (240 mg IM) plus doxycycline (100 mg two times per day for 7 days) have been used as first-line empirical treatment for urethritis and cervicitis for more than 25 years and although a recent study identified several gonorrhoea treatment failures, no gonococcal isolates with substantially elevated MICs of gentamicin were detected.29 Nevertheless, the surveillance of gonococcal susceptibility to gentamicin internationally remains scarce. Accordingly, enhanced, quality-assured surveillance of gentamicin MICs in N. gonorrhoeae is crucial internationally. Furthermore, it would be exceedingly valuable if EUCAST or CLSI recommended evidence-based clinical gentamicin resistance breakpoints for N. gonorrhoeae.
To predict gentamicin-resistance mutations that might evolve in N. gonorrhoeae if gentamicin becomes more widely used internationally, we selected for gentamicin resistance in WHO X35 in vitro using serial culture passages on gentamicin-gradient agar plates. This resulted in mutants of WHO X that expressed up to 5-fold increases in the MICs of gentamicin as well as of other aminoglycosides (Table 1). We subsequently found several mutations in the WHO X mutants with increased MICs of the aminoglycosides (WHO X10–100) and showed that high-level gentamicin resistance in N. gonorrhoeae arises in vitro in a stepwise accumulative manner (Figure 1). The most substantial increases in the gentamicin MIC were caused by mutations in fusA and ubiM encoding EF-G and UbiM involved in the biosynthetic pathway of ubiquinone (also called coenzyme Q),52 respectively. Similarly, Holley et al.34 recently showed that the A563V alteration in domain IV of EF-G conferred low-level gentamicin resistance (MIC = 32 mg/L); however, a mutation in ubiB in the gentamicin-resistant mutant was found as well.
Herein, we found that the ubiM gene mutation D186N had evolved in WHO X mutants with low-level gentamicin resistance (MIC = 32 mg/L) without any fusA mutations. Subsequently, a stepwise accumulation of mutations in several genes were observed before the final fusA (EF-GM520I) mutation caused high-level gentamicin resistance (Figure 1). The coenzyme Q is involved in aerobic respiration53 with a highly conserved pathway for biosynthesis, which involves several ubi genes54 and plays a major role in the regulatory network that allows facultative anaerobic bacteria to sense various respiratory growth conditions. Furthermore, the proton-pump NADH-ubiquinone oxidoreductase (complex I) catalyses electron transfer via proton translocation from the negative inner to the positive outer side of the membrane, therefore generating a proton-motive force (PMF). PMF is essential for the import of antibiotics55–57 and consequently, ubi mutations cause low-level resistance to aminoglycosides by suboptimal transport of the positively charged antimicrobial molecule into the cell. For example, ubi mutations have led to resistance to aminoglycosides in E. coli, specifically gentamicin and streptomycin.58,59 Similar mutations in several ubi genes may be unrecognized AMR determinants also in N. gonorrhoeae and the potential roles of coenzyme Q and ubi gene mutations need to be further investigated.
During protein synthesis, EF-G binds the ribosomal complex and facilitates the movement (translocation) of tRNAs and mRNA by one codon. EF-G domain IV binds to the 30S ribosomal A-site and to ribosomal protein S12 via domains III and V, controlling the conformation of EF-G during translocation.60 Interestingly, gentamicin binds the 30S ribosomal A-site RNA and inhibits translocation61 and most likely competes with the binding of EF-G. We propose that mutations found in EF-G in domain IV (M520I), the binding site to the 30S ribosome, is the cause of high-level resistance to gentamicin by outcompeting the antimicrobial for the A-site. However, the high-level gentamicin resistance caused SCVs of WHO X, as previously reported in gentamicin-resistant S. aureus,62 and competitive coculture in an HFIM for gonorrhoea showed that these SCVs also had impaired biofitness compared with WHO X.
In conclusion, we describe the first high-level gentamicin-resistant N. gonorrhoeae isolate (MIC = 128 mg/L), which was selected in vitro through experimental evolution. The most substantial increases in the MICs of gentamicin and other aminoglycosides such as kanamycin, tobramycin and amikacin were caused by resistance mutations in fusA (G1560A and G1904T encoding EF-G M520I and R635L, respectively) and ubiM (D186N). However, the high-level gentamicin-resistant N. gonorrhoeae mutant showed impaired biofitness. Additional studies are required to confirm the role of all the mutations potentially involved in gentamicin resistance and to examine whether N. gonorrhoeae could restore biofitness through compensatory genetic events during continued exposure to gentamicin.
Funding
This project was supported by the Örebro County Council Research Committee and the Foundation for Medical Research at Örebro University Hospital, Örebro, Sweden. NIH grant AI47609 supports W.M.S. W.M.S. is the recipient of a Senior Research Career Scientist award from the Medical Research Service of the Department of Veterans Affairs.
Transparency declarations
None to declare. The contents of this article are solely the responsibility of the authors and do not necessarily reflect the official views of the National Institutes of Health, the Department of Veterans Affairs or the United States government.
Supplementary data
Figures S1–S3 are available as Supplementary data at JAC Online.