-
PDF
- Split View
-
Views
-
Cite
Cite
Felix Lange, Niels Pfennigwerth, Lisa-Marie Höfken, Sören G Gatermann, Martin Kaase, Characterization of mutations in Escherichia coli PBP2 leading to increased carbapenem MICs, Journal of Antimicrobial Chemotherapy, Volume 74, Issue 3, March 2019, Pages 571–576, https://doi.org/10.1093/jac/dky476
- Share Icon Share
Abstract
To examine the impact on carbapenem resistance of mutations in Escherichia coli PBP2 detected in clinical isolates showing increased MICs of imipenem, but not of meropenem.
The mutations in the PBP2-encoding gene mrdA were introduced into E. coli DH5α using the helper plasmid pTKRED. β-Lactam MICs were determined by broth microdilution and interpreted according to EUCAST. Mutants were screened for secondary mutations by WGS. To detect a possible fitness cost related to these mutations, the generation times of the mutants and E. coli DH5α were measured and their cell morphology was examined.
The 10 mutation patterns introduced into mrdA increased the MICs of imipenem and doripenem in all cases and of meropenem and mecillinam in some cases, but had no effect on ertapenem resistance. While no significant alteration of the generation time of the mutants could be detected, several mutants showed increased transverse diameters and thus altered cell morphology.
Here we describe mutation patterns in PBP2 that contribute to increased MICs of carbapenems for clinical isolates of E. coli. We show that different mutations affect carbapenem MICs differently and that the mutations do not impact generation time, although some mutations affect cell morphology.
Introduction
Escherichia coli is the most prevalent cause of urinary tract infections and also often accounts for bacteraemia in hospitalized patients.1 Especially in the treatment of the latter, carbapenem resistance imposes a serious limitation on available treatment options. Carbapenem resistance among Enterobacterales is, in many cases, based upon carbapenemase production or loss of porins in combination with AmpC or ESBL hyperproduction.2 The most prevalent carbapenemases in German isolates of E. coli are OXA-48, VIM-1, KPC-2 and NDM-1.3 Increased MICs of carbapenems, especially imipenem, due to amino acid alterations in PBP2 have also been reported for isolates of E. coli.4 Between 2011 and 2016, 2840 isolates of E. coli that were non-susceptible to imipenem, meropenem or ertapenem according to EUCAST clinical breakpoints were referred to the German national reference laboratory for Gram-negative MDR bacteria. Fifty-two of these isolates showed inhibition zone diameters for imipenem close to the breakpoint or below, while the inhibition zone diameters for meropenem clearly indicated susceptibility. The cause of this difference in susceptibility for these two agents was investigated.
Materials and methods
Strains
The 52 isolates of E. coli that were investigated showed inhibition zone diameters for imipenem ≤26 mm and inhibition zones ≥26 mm for meropenem.
Detection of carbapenemases
For carbapenemase detection, a modified Hodge test with imipenem, meropenem and ertapenem was performed. Combined disc tests with EDTA and boronic acid and disc diffusion for temocillin were performed in addition to PCR and subsequent sequencing for blaKPC, blaOXA-48 like, blaVIM, blaIMP and blaNDM as previously described.5
Susceptibility testing
Susceptibility testing was performed by disc diffusion and broth microdilution according to EUCAST (Breakpoint Tables v_8.1, 16 May 2018). MIC increases of one dilution step were considered insignificant.
DNA preparation for PCR
Bacterial cells were grown overnight in 4 mL of LB broth and DNA was prepared using the NucleoSpin Tissue Kit (Macherey-Nagel, Düren, Germany) following the manufacturer’s instructions.
PCR for mrdA
All 52 strains were screened by PCR and subsequent Sanger sequencing using the primers mrdA_flnk_fwd (ACCGTGAGTGATAAGGGAGC) and mrdA_flnk_rev (TGGCGCTCCAGATAACCAGG) for amino-acid-altering mutations in mrdA, the gene coding for PBP2.
Generation of mutants
Mutants of E. coli DH5α carrying the aberrant mrdA alleles were generated as previously described.6 Briefly, the helper plasmid pTKRED was transformed into E. coli DH5α, rendering the cells resistant to spectinomycin. Transformed cells were cultured in LB medium with 50 mg/L spectinomycin and 0.1 mM IPTG to an OD600 of 0.5. Cells were harvested and incubated in ice-cold 10% glycerol on ice for 30 min. The cells were pelleted, resuspended in 10% glycerol and stored at −80°C. PCR products were purified using the NucleoSpin PCR and Gel Cleanup-Kit (Macherey-Nagel) and were transformed by electroporation into the competent DH5α-pTKRED cells. The transformed cells were then selected for integration of the mutated mrdA allele on LB agar containing 0.5 mg/L imipenem.
Growth experiments
Microscopic inspection of cell morphologies
E. coli DH5α and mutant strains were inoculated into LB broth and incubated at 37°C for 24 h. Samples from stationary-phase cultures were taken and cells were harvested, streaked out on a microscope slide and stained with safranine. Cells were examined and photographed using an Olympus BX40 microscope (Olympus K.K., Hamburg, Germany)
WGS and data analysis
The mutant strains and E. coli DH5α were cultured in LB broth at 37°C until an OD600 of 0.7 was reached. High-molecular-weight genomic DNA was isolated using the Genomic Tips 100/G Kit (Qiagen, Hilden, Germany) according to the manufacturer’s instructions. A Qubit 2.0 fluorometer was used to determine the DNA concentration. The integrity of high-molecular-weight DNA was assessed by agarose gel electrophoresis. Libraries were prepared using the Illumina Nextera XT Library Prep Kit (Illumina, Eindhoven, Netherlands). WGS was performed on an Illumina MiSeq platform by Macrogen (Seoul, South Korea) with 2 × 300 bp paired-end reads. Furthermore, genomic DNA of E. coli DH5α was sequenced using the MinION device (Oxford Nanopore Technologies, Oxford, UK). A reference genome of E. coli DH5α was assembled from the Nanopore data and the Illumina data using the hybridSPAdes assembler.7,8
Illumina reads of the mutants were mapped on the reference genome using Bowtie2 with standard parameters and mutations in the genome were detected using SAMtools and BCFtools, each with standard parameters.9–11 The detected SNPs were filtered using a minimum quality score of 40 and a ratio of the number of reads advocating for an SNP to the number of reads advocating for no SNP of at least 2.
Results and discussion
Between 2011 and 2016, 52 isolates of E. coli referred to the German national reference laboratory for Gram-negative MDR bacteria showed decreased inhibition zone diameters for imipenem (≤26 mm), whereas their inhibition zone diameters for meropenem were larger (≥26 mm). Carbapenemase production was excluded as a possible cause of this phenotype for all of these strains. As it was previously shown that decreased susceptibility to imipenem can be caused by mutations in PBP2, these strains were screened for mutations in mrdA, the gene coding for PBP2. Among these 52 strains, 34 exhibited amino-acid-altering mutations in mrdA. In PBP2 of these 34 strains, 23 unique amino acid substitution patterns were detected, of which 11 could be introduced into E. coli DH5α. Five of the 12 that could not be introduced into E. coli DH5α exhibited very similar mutation patterns to the ones that could be introduced and additionally contained one mutation outside of the transpeptidase domain of PBP2 (amino acid positions 271–609).12 When attempting to introduce these mutation patterns, partial recombination of the mutated mrdA alleles occurred. This introduced the mutations inside the transpeptidase domain without introducing those outside it. It is therefore questionable whether the mutations outside the transpeptidase domain contributed to increased β-lactam MICs for the clinical isolates.
The PBP2 alterations detected most frequently in this set of strains comprised the mutations A388S, T331P, M574I/L, A329G and T390P (Table 1). The mutations M574I, A329G and T331P were shown to contribute to increased carbapenem MICs alone while the mutations M574L, A388S and T390P only occurred in combination with other mutations, as shown in Table 1. Therefore, the effect of the latter mutations alone on carbapenem MICs is not known. All mutation patterns that could be introduced into E. coli DH5α caused an increase in MICs of imipenem and doripenem of at least 4-fold for both imipenem and doripenem and up to 8-fold and 16-fold for imipenem and doripenem, respectively. Many mutation patterns also increased the MICs of meropenem, by up to 8-fold. The original isolates carrying these mutations showed decreased susceptibility to imipenem, but not to meropenem. However, these mutations influenced meropenem MICs to a similar extent as imipenem MICs. This phenomenon is likely caused by the fact that the EUCAST clinical breakpoint is identical for imipenem and meropenem, although the median of the WT MIC distribution of meropenem is significantly lower than that of imipenem.13,14 Therefore, an identical increase in MIC of imipenem and meropenem edges the imipenem MIC closer to the breakpoint, while the MIC of meropenem clearly remains in the susceptible range. Interestingly, none of the mutation patterns increased the MIC of ertapenem. The penicillin mecillinam, which is not a carbapenem, binds exclusively to PBP2 in E. coli.15 Some mutations in PBP2 introduced into E. coli DH5α, such as T331P or W370_K371insK, affected the MIC of mecillinam on their own, while other mutations, such as A329G or M574I, only did so in combination with other mutations (Table 1). Other mutations again did not affect mecillinam MICs at all. Although the mutations T331P, W370_K371insK, A329G and M574I/L also affected the MICs of imipenem and doripenem, there was no obvious correlation between the MIC increase for mecillinam and MIC increase for carbapenems. MICs of β-lactams other than these were not altered by any of the mutation patterns. Furthermore, multiple amino acid substitutions tended to have a greater impact on carbapenem MICs than a single mutation (Table 1). The effect of the amino acid alterations hence appeared to be cumulative. The distribution of the mutations over the sequence of PBP2 reveals two possible mutation hotspots. The first comprises the amino acid residues 329–390 and the other comprises the residues 543–602.
Amino acid alterations introduced into E. coli DH5α and MICs (mg/L) of carbapenems and mecillinam for the respective mutants determined by broth microdilution
Strain . | Amino acid alteration(s) in PBP2 . | Imipenem . | Meropenem . | Ertapenem . | Doripenem . | Mecillinam . |
---|---|---|---|---|---|---|
DH5α | – | 0.125 | ≤0.03125 | ≤0.0625 | ≤0.03125 | ≤1 |
MUT9030 | M574I | 0.5 | 0.0625 | ≤0.0625 | 0.125 | ≤1 |
MUT3551 | M574I, A388S | 1 | 0.0625 | ≤0.0625 | 0.25 | 2 |
MUT_A* | M574L, L573Q | 0.5 | 0.125 | ≤0.0625 | 0.25 | 2 |
MUT_B* | M574L, A388S, L573Q | 1 | 0.25 | ≤0.0625 | 0.5 | ≤1 |
MUT_C* | T390P, A388S | 0.5 | 0.125 | ≤0.0625 | 0.25 | ≤1 |
MUT_D* | T390P, A388S, L378V | 1 | 0.125 | ≤0.0625 | 0.25 | ≤1 |
MUT26913 | T390P, A388S, A329G | 1 | 0.125 | ≤0.0625 | 0.5 | 4 |
MUT29144 | A329G | 0.5 | 0.125 | ≤0.0625 | 0.125 | ≤1 |
MUT12997 | A329G, A543V | 1 | 0.0625 | ≤0.0625 | 0.25 | 2 |
MUT3415 | A329G, A388S | 1 | 0.0625 | ≤0.0625 | 0.25 | 2 |
MUT8846 | T331P | 1 | 0.0625 | ≤0.0625 | 0.125 | 4 |
MUT3865 | T331P, A388S | 1 | 0.125 | ≤0.0625 | 0.25 | 4 |
MUT10167 | G601_T602insTR | 0.5 | ≤0.03125 | ≤0.0625 | 0.125 | ≤1 |
MUT10902 | W370_K371insK | 0.5 | 0.0625 | ≤0.0625 | 0.25 | 2 |
Strain . | Amino acid alteration(s) in PBP2 . | Imipenem . | Meropenem . | Ertapenem . | Doripenem . | Mecillinam . |
---|---|---|---|---|---|---|
DH5α | – | 0.125 | ≤0.03125 | ≤0.0625 | ≤0.03125 | ≤1 |
MUT9030 | M574I | 0.5 | 0.0625 | ≤0.0625 | 0.125 | ≤1 |
MUT3551 | M574I, A388S | 1 | 0.0625 | ≤0.0625 | 0.25 | 2 |
MUT_A* | M574L, L573Q | 0.5 | 0.125 | ≤0.0625 | 0.25 | 2 |
MUT_B* | M574L, A388S, L573Q | 1 | 0.25 | ≤0.0625 | 0.5 | ≤1 |
MUT_C* | T390P, A388S | 0.5 | 0.125 | ≤0.0625 | 0.25 | ≤1 |
MUT_D* | T390P, A388S, L378V | 1 | 0.125 | ≤0.0625 | 0.25 | ≤1 |
MUT26913 | T390P, A388S, A329G | 1 | 0.125 | ≤0.0625 | 0.5 | 4 |
MUT29144 | A329G | 0.5 | 0.125 | ≤0.0625 | 0.125 | ≤1 |
MUT12997 | A329G, A543V | 1 | 0.0625 | ≤0.0625 | 0.25 | 2 |
MUT3415 | A329G, A388S | 1 | 0.0625 | ≤0.0625 | 0.25 | 2 |
MUT8846 | T331P | 1 | 0.0625 | ≤0.0625 | 0.125 | 4 |
MUT3865 | T331P, A388S | 1 | 0.125 | ≤0.0625 | 0.25 | 4 |
MUT10167 | G601_T602insTR | 0.5 | ≤0.03125 | ≤0.0625 | 0.125 | ≤1 |
MUT10902 | W370_K371insK | 0.5 | 0.0625 | ≤0.0625 | 0.25 | 2 |
Mutation patterns that were introduced into E. coli DH5α by partial recombination, but were not detected in any clinical isolates, are marked with asterisks. The transpeptidase domain of PBP2 comprises amino acid positions 271–609.
Amino acid alterations introduced into E. coli DH5α and MICs (mg/L) of carbapenems and mecillinam for the respective mutants determined by broth microdilution
Strain . | Amino acid alteration(s) in PBP2 . | Imipenem . | Meropenem . | Ertapenem . | Doripenem . | Mecillinam . |
---|---|---|---|---|---|---|
DH5α | – | 0.125 | ≤0.03125 | ≤0.0625 | ≤0.03125 | ≤1 |
MUT9030 | M574I | 0.5 | 0.0625 | ≤0.0625 | 0.125 | ≤1 |
MUT3551 | M574I, A388S | 1 | 0.0625 | ≤0.0625 | 0.25 | 2 |
MUT_A* | M574L, L573Q | 0.5 | 0.125 | ≤0.0625 | 0.25 | 2 |
MUT_B* | M574L, A388S, L573Q | 1 | 0.25 | ≤0.0625 | 0.5 | ≤1 |
MUT_C* | T390P, A388S | 0.5 | 0.125 | ≤0.0625 | 0.25 | ≤1 |
MUT_D* | T390P, A388S, L378V | 1 | 0.125 | ≤0.0625 | 0.25 | ≤1 |
MUT26913 | T390P, A388S, A329G | 1 | 0.125 | ≤0.0625 | 0.5 | 4 |
MUT29144 | A329G | 0.5 | 0.125 | ≤0.0625 | 0.125 | ≤1 |
MUT12997 | A329G, A543V | 1 | 0.0625 | ≤0.0625 | 0.25 | 2 |
MUT3415 | A329G, A388S | 1 | 0.0625 | ≤0.0625 | 0.25 | 2 |
MUT8846 | T331P | 1 | 0.0625 | ≤0.0625 | 0.125 | 4 |
MUT3865 | T331P, A388S | 1 | 0.125 | ≤0.0625 | 0.25 | 4 |
MUT10167 | G601_T602insTR | 0.5 | ≤0.03125 | ≤0.0625 | 0.125 | ≤1 |
MUT10902 | W370_K371insK | 0.5 | 0.0625 | ≤0.0625 | 0.25 | 2 |
Strain . | Amino acid alteration(s) in PBP2 . | Imipenem . | Meropenem . | Ertapenem . | Doripenem . | Mecillinam . |
---|---|---|---|---|---|---|
DH5α | – | 0.125 | ≤0.03125 | ≤0.0625 | ≤0.03125 | ≤1 |
MUT9030 | M574I | 0.5 | 0.0625 | ≤0.0625 | 0.125 | ≤1 |
MUT3551 | M574I, A388S | 1 | 0.0625 | ≤0.0625 | 0.25 | 2 |
MUT_A* | M574L, L573Q | 0.5 | 0.125 | ≤0.0625 | 0.25 | 2 |
MUT_B* | M574L, A388S, L573Q | 1 | 0.25 | ≤0.0625 | 0.5 | ≤1 |
MUT_C* | T390P, A388S | 0.5 | 0.125 | ≤0.0625 | 0.25 | ≤1 |
MUT_D* | T390P, A388S, L378V | 1 | 0.125 | ≤0.0625 | 0.25 | ≤1 |
MUT26913 | T390P, A388S, A329G | 1 | 0.125 | ≤0.0625 | 0.5 | 4 |
MUT29144 | A329G | 0.5 | 0.125 | ≤0.0625 | 0.125 | ≤1 |
MUT12997 | A329G, A543V | 1 | 0.0625 | ≤0.0625 | 0.25 | 2 |
MUT3415 | A329G, A388S | 1 | 0.0625 | ≤0.0625 | 0.25 | 2 |
MUT8846 | T331P | 1 | 0.0625 | ≤0.0625 | 0.125 | 4 |
MUT3865 | T331P, A388S | 1 | 0.125 | ≤0.0625 | 0.25 | 4 |
MUT10167 | G601_T602insTR | 0.5 | ≤0.03125 | ≤0.0625 | 0.125 | ≤1 |
MUT10902 | W370_K371insK | 0.5 | 0.0625 | ≤0.0625 | 0.25 | 2 |
Mutation patterns that were introduced into E. coli DH5α by partial recombination, but were not detected in any clinical isolates, are marked with asterisks. The transpeptidase domain of PBP2 comprises amino acid positions 271–609.
While none of the mutations increased the MIC of any carbapenem above the EUCAST clinical breakpoint in E. coli DH5α, six mutation patterns increased the MIC of imipenem above the epidemiological cut-off as defined by EUCAST. Furthermore, it is possible that mutations in PBP2 may work synergistically with carbapenemase production, diminished porin production and AmpC hyperproduction, thereby conferring clinically relevant resistance to carbapenems. However, this requires further investigation. Detailed knowledge of these factors contributing to carbapenem resistance and how they interact could provide better understanding of how carbapenem resistance forms in E. coli and Gram-negative bacteria in general.
As PBP2 plays an important role in bacterial growth and cell shape regulation, impacts of the mutations on the generation time of mutants were possible.16 Therefore, growth experiments with the mutants and E. coli DH5α were conducted. These experiments did not indicate significant differences in generation times between the mutants and E. coli DH5α (Table 2). Hence, no profound fitness cost of the PBP2 mutations in terms of increased generation time could be detected in vitro. To detect possible impacts of the mutations on cell morphology, cells of E. coli DH5α and the mutants generated were inspected microscopically. The transverse diameter of the cells of several mutants was significantly increased compared with E. coli DH5α (Figure 1). This indicates that at least some of the alterations in PBP2 have an impact on the physiological function of PBP2 leading to altered cell morphology, but not to an increase in generation time. It has previously been described that a deletion of mrdA leads to formation of misshaped, spherical cells in E. coli.17 While the cell morphology is not entirely spherical in the mutants at hand, the increase in transverse diameter may indicate that these mutations lead to decreased activity of PBP2 in peptidoglycan synthesis.
Strain . | Mean generation time (min) . | Significant difference from E. coli DH5α . |
---|---|---|
DH5α | 53.4 ± 1.79 | – |
MUT3415_2 | 53.2 ± 0.93 | no |
MUT3551_1 | 52.3 ± 3.61 | no |
MUT3865f | 54.5 ± 3.66 | no |
MUT8846_1 | 51.1 ± 2.95 | no |
MUT9030_9 | 55.3 ± 0.49 | no |
MUT10167_1 | 56.2 ± 2.10 | no |
MUT10902_2 | 54.4 ± 2.06 | no |
MUT12997_5 | 53.3 ± 3.27 | no |
MUT26913_1 | 50.5 ± 3.61 | no |
MUT29144_4 | 53.6 ± 0.95 | no |
Strain . | Mean generation time (min) . | Significant difference from E. coli DH5α . |
---|---|---|
DH5α | 53.4 ± 1.79 | – |
MUT3415_2 | 53.2 ± 0.93 | no |
MUT3551_1 | 52.3 ± 3.61 | no |
MUT3865f | 54.5 ± 3.66 | no |
MUT8846_1 | 51.1 ± 2.95 | no |
MUT9030_9 | 55.3 ± 0.49 | no |
MUT10167_1 | 56.2 ± 2.10 | no |
MUT10902_2 | 54.4 ± 2.06 | no |
MUT12997_5 | 53.3 ± 3.27 | no |
MUT26913_1 | 50.5 ± 3.61 | no |
MUT29144_4 | 53.6 ± 0.95 | no |
Strain . | Mean generation time (min) . | Significant difference from E. coli DH5α . |
---|---|---|
DH5α | 53.4 ± 1.79 | – |
MUT3415_2 | 53.2 ± 0.93 | no |
MUT3551_1 | 52.3 ± 3.61 | no |
MUT3865f | 54.5 ± 3.66 | no |
MUT8846_1 | 51.1 ± 2.95 | no |
MUT9030_9 | 55.3 ± 0.49 | no |
MUT10167_1 | 56.2 ± 2.10 | no |
MUT10902_2 | 54.4 ± 2.06 | no |
MUT12997_5 | 53.3 ± 3.27 | no |
MUT26913_1 | 50.5 ± 3.61 | no |
MUT29144_4 | 53.6 ± 0.95 | no |
Strain . | Mean generation time (min) . | Significant difference from E. coli DH5α . |
---|---|---|
DH5α | 53.4 ± 1.79 | – |
MUT3415_2 | 53.2 ± 0.93 | no |
MUT3551_1 | 52.3 ± 3.61 | no |
MUT3865f | 54.5 ± 3.66 | no |
MUT8846_1 | 51.1 ± 2.95 | no |
MUT9030_9 | 55.3 ± 0.49 | no |
MUT10167_1 | 56.2 ± 2.10 | no |
MUT10902_2 | 54.4 ± 2.06 | no |
MUT12997_5 | 53.3 ± 3.27 | no |
MUT26913_1 | 50.5 ± 3.61 | no |
MUT29144_4 | 53.6 ± 0.95 | no |
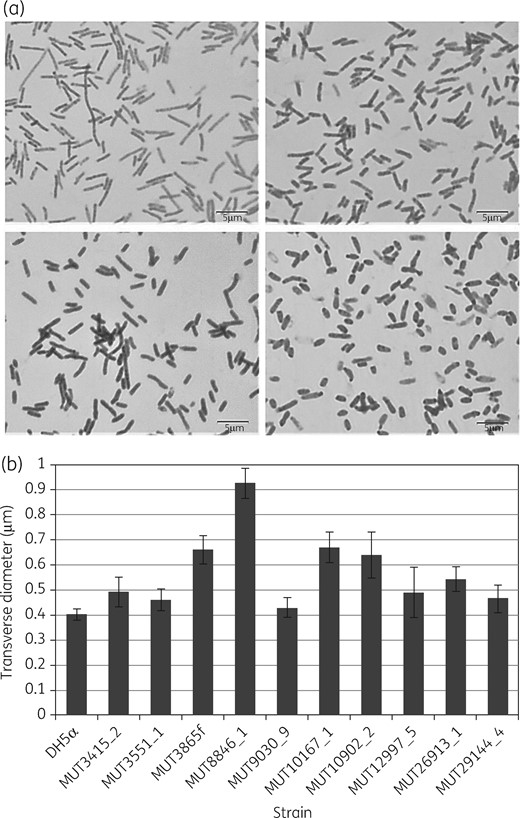
Morphological differences between PBP2 mutants and E. coli DH5α. (a) Microscopic photographs of E. coli DH5α (top left), MUT10167 (top right), MUT3865 (bottom left) and MUT8846 (bottom right). (b) Transverse diameters of E. coli DH5α and the PBP2 mutants (standard error indicated).
As mutants were selected using imipenem, selection of secondary mutations contributing to carbapenem resistance may have occurred. Therefore, the E. coli DH5α strain used for generation of mutants and all mutants were subjected to WGS. Mapping of the reads of the mutants’ genomes on the reference genome E. coli DH5α revealed that all mutations introduced into the mutants were present and that secondary mutations occurred in some of the mutants (Table 3).
Secondary mutations detected in the mutants by WGS that either altered the amino acid sequence of a gene product or were close to annotated genetic features, thereby possibly changing expression levels
Mutant . | Mutated locus . | Alteration of gene product . |
---|---|---|
MUT3415 | – | – |
MUT3551 | IS1 family transposase | insertion of QIQVLP |
MUT3865 | IS1 family transposase | insertion of QNQVIPLNSLRMP |
MUT8846 | IS1 family transposase | insertion of QIQVLP |
MUT_B | d-Ala-d-Ala dipeptidase | A131V |
MUT9030 | – | – |
MUT10167 | IS1 family transposase | insertion of QIQVLP |
MUT10902 | – | – |
MUT12997 | IS1 family transposase | insertion of QNQVIPLNSLRMP |
MUT26913 | 9 bp insertion directly upstream of transcription factor close to lacR | – |
13 bp insertion directly downstream of lacI | – | |
28 bp insertion in recombination regulator recX | frameshift | |
recombinase RecA | D161G | |
arabinose operon regulatory protein AraC | N16I | |
arabinose operon regulatory protein AraC | V65N | |
arabinose operon regulatory protein AraC | G118P | |
arabinose operon regulatory protein AraC | R231T | |
arabinose operon regulatory protein AraC | I232D | |
arabinose operon regulatory protein AraC | S233A | |
arabinose operon regulatory protein AraC | T242G | |
arabinose operon regulatory protein AraC | R263P | |
arabinose operon regulatory protein AraC | F276E | |
Mut29144 | – | – |
Mutant . | Mutated locus . | Alteration of gene product . |
---|---|---|
MUT3415 | – | – |
MUT3551 | IS1 family transposase | insertion of QIQVLP |
MUT3865 | IS1 family transposase | insertion of QNQVIPLNSLRMP |
MUT8846 | IS1 family transposase | insertion of QIQVLP |
MUT_B | d-Ala-d-Ala dipeptidase | A131V |
MUT9030 | – | – |
MUT10167 | IS1 family transposase | insertion of QIQVLP |
MUT10902 | – | – |
MUT12997 | IS1 family transposase | insertion of QNQVIPLNSLRMP |
MUT26913 | 9 bp insertion directly upstream of transcription factor close to lacR | – |
13 bp insertion directly downstream of lacI | – | |
28 bp insertion in recombination regulator recX | frameshift | |
recombinase RecA | D161G | |
arabinose operon regulatory protein AraC | N16I | |
arabinose operon regulatory protein AraC | V65N | |
arabinose operon regulatory protein AraC | G118P | |
arabinose operon regulatory protein AraC | R231T | |
arabinose operon regulatory protein AraC | I232D | |
arabinose operon regulatory protein AraC | S233A | |
arabinose operon regulatory protein AraC | T242G | |
arabinose operon regulatory protein AraC | R263P | |
arabinose operon regulatory protein AraC | F276E | |
Mut29144 | – | – |
Secondary mutations detected in the mutants by WGS that either altered the amino acid sequence of a gene product or were close to annotated genetic features, thereby possibly changing expression levels
Mutant . | Mutated locus . | Alteration of gene product . |
---|---|---|
MUT3415 | – | – |
MUT3551 | IS1 family transposase | insertion of QIQVLP |
MUT3865 | IS1 family transposase | insertion of QNQVIPLNSLRMP |
MUT8846 | IS1 family transposase | insertion of QIQVLP |
MUT_B | d-Ala-d-Ala dipeptidase | A131V |
MUT9030 | – | – |
MUT10167 | IS1 family transposase | insertion of QIQVLP |
MUT10902 | – | – |
MUT12997 | IS1 family transposase | insertion of QNQVIPLNSLRMP |
MUT26913 | 9 bp insertion directly upstream of transcription factor close to lacR | – |
13 bp insertion directly downstream of lacI | – | |
28 bp insertion in recombination regulator recX | frameshift | |
recombinase RecA | D161G | |
arabinose operon regulatory protein AraC | N16I | |
arabinose operon regulatory protein AraC | V65N | |
arabinose operon regulatory protein AraC | G118P | |
arabinose operon regulatory protein AraC | R231T | |
arabinose operon regulatory protein AraC | I232D | |
arabinose operon regulatory protein AraC | S233A | |
arabinose operon regulatory protein AraC | T242G | |
arabinose operon regulatory protein AraC | R263P | |
arabinose operon regulatory protein AraC | F276E | |
Mut29144 | – | – |
Mutant . | Mutated locus . | Alteration of gene product . |
---|---|---|
MUT3415 | – | – |
MUT3551 | IS1 family transposase | insertion of QIQVLP |
MUT3865 | IS1 family transposase | insertion of QNQVIPLNSLRMP |
MUT8846 | IS1 family transposase | insertion of QIQVLP |
MUT_B | d-Ala-d-Ala dipeptidase | A131V |
MUT9030 | – | – |
MUT10167 | IS1 family transposase | insertion of QIQVLP |
MUT10902 | – | – |
MUT12997 | IS1 family transposase | insertion of QNQVIPLNSLRMP |
MUT26913 | 9 bp insertion directly upstream of transcription factor close to lacR | – |
13 bp insertion directly downstream of lacI | – | |
28 bp insertion in recombination regulator recX | frameshift | |
recombinase RecA | D161G | |
arabinose operon regulatory protein AraC | N16I | |
arabinose operon regulatory protein AraC | V65N | |
arabinose operon regulatory protein AraC | G118P | |
arabinose operon regulatory protein AraC | R231T | |
arabinose operon regulatory protein AraC | I232D | |
arabinose operon regulatory protein AraC | S233A | |
arabinose operon regulatory protein AraC | T242G | |
arabinose operon regulatory protein AraC | R263P | |
arabinose operon regulatory protein AraC | F276E | |
Mut29144 | – | – |
The mutants MUT3551, MUT3865, MUT8846, MUT10167 and MUT12997 yielded amino-acid-altering mutations in a gene coding for an IS1 family transposase. Mutant MUT_B showed a mutation causing an A131V amino acid substitution in a d-alanyl-d-alanine (d-Ala-d-Ala) dipeptidase. It is known that similar enzymes expressed by Enterobacterales hydrolyse d-Ala-d-Ala dipeptides. In combination with other factors, this can enable the expressing bacterium to use d-Ala-d-Ala dipeptides as a carbon source.18 Although d-Ala-d-Ala dipeptides occur in the immature oligopeptide of the peptidoglycan, a function of such enzymes in the context of peptidoglycan metabolism is not described. Mutant MUT26913 yielded a frameshift mutation in the recombination regulator protein gene recX, a D161G amino acid substitution in the recombinase RecA and nine amino acid substitutions in the arabinose operon regulation protein AraC (N16I, V65N, G118P, R231T, I232D, S233A, T242G, R263P and F276E). Furthermore, two sequence alterations were found in an intergenic sequence without annotated genetic features in mutant MUT3415. Other mutations detected outside mrdA and the genes named above did not alter the protein sequence of their gene products. Although it can never be entirely excluded that alterations in proteins affect seemingly unrelated processes, none of the altered genes and proteins are obviously related to peptidoglycan metabolism or antibiotic resistance. The observed alterations in carbapenem resistance are hence most likely caused by the introduced mutations in PBP2.
Conclusions
Here we describe several previously unknown mutations in PBP2 of E. coli that lead to decreased susceptibility to carbapenems. Furthermore, we describe a cumulative effect of these mutations on carbapenem MICs and provide data indicating that while the physiological function of PBP2 may be impaired, no profound effect on generation time is associated with these mutations. While mutations in PBP2 alone do not confer clinically relevant carbapenem resistance, they may contribute to it together with decreased porin production, AmpC hyperproduction or production of carbapenemases. This adds yet another piece of information contributing to a better understanding of the multifactorial cause of carbapenem resistance in Enterobacterales.
Acknowledgements
We would like to thank Jasmin Krulias, Anja Kaminski, Susanne Friedrich, Doris Jaromin, Anke Albrecht, Brigitte Hemmerle, Marion Schmidt, Nadine Frey and Svenja Hirle for excellent technical assistance.
Funding
This work was supported by the Robert Koch Institute with funds provided by the German Ministry of Health (grant no. 1369–402).
Transparency declarations
N. P. has received speaker fees from bioMérieux. S. G. G. has received speaker fees from bioMérieux and Bio-Rad. M. K. has received speaker or consultancy fees or research grants from Amplex, AstraZeneca, Bayer Vital, Becton Dickinson, bioMérieux, Bio-Rad, Bruker Daltonics, Cepheid, Infectopharm, MSD, Pfizer, Roche Diagnostics and Siemens Healthcare. F. L. and L.-M. H.: none to declare.
References
EUCAST. EUCAST Clinical Breakpoints. http://www.eucast.org/clinical_breakpoints/.
EUCAST. MIC and Zone Diameter Distributions and ECOFFs. http://www.eucast.org/mic_distributions_and_ecoffs/.