-
PDF
- Split View
-
Views
-
Cite
Cite
Bradley G Stevens, The ups and downs of traps: environmental impacts, entanglement, mitigation, and the future of trap fishing for crustaceans and fish, ICES Journal of Marine Science, Volume 78, Issue 2, March 2021, Pages 584–596, https://doi.org/10.1093/icesjms/fsaa135
- Share Icon Share
Abstract
Fishing has many types of impacts on the marine environment. Degradation of seafloor habitats by trawling has been widely studied, along with bycatch mortality, and ghost fishing by traps. Traps or pots are commonly used for catching crustaceans and other organisms, but little research has been conducted on the environmental impacts of trap fishing. Trap fishing causes direct impacts on benthic habitats during setting and retrieval, including dragging along the seafloor, which can lead to the damage and destruction of habitat components such as corals, sponges, and other epifauna. Lines connecting multiple traps increase the overall footprint and cause additional damage. Lost traps and debris can cause damage to coral, submerged aquatic vegetation, and epifauna, especially if moved by storms. Although the trap footprint is small, movement of the trap can expand the impact footprint by an order of magnitude. Trap lines cause whale entanglement and death, and there is much interest in reducing the number of vertical lines as well as their potential for entanglement. New technologies for deploying, locating, and recovering traps are now being developed. The future of trap fisheries will require research on new ways to reduce their negative impacts on benthic and pelagic resources.
Introduction
“Some Cupid kills with arrows, some with traps” W. Shakespeare
Most fisheries are managed for a single species, but increasing overexploitation of populations and degradation of ecosystems has supported a shift towards ecosystem-based fishery management (EBFM). The goals of EBFM are to obtain sustainable long-term socio-economic benefits without degrading species assemblages, environmental quality, or ecosystem integrity (Pikitch et al., 2004). This would require managers to “shift the burden of proof so that fishing would not take place unless it could be shown not to harm key components of the ecosystem” (Pikitch et al., 2004).
Fishing has many types of ecosystem impacts including habitat degradation, mortality of bycatch species, and changes in population demographics and ecosystem structure (Pikitch et al., 2004). Among these, the direct impacts of fishing gear on habitats may be the most serious and have been studied by numerous authors. However, most such studies have concentrated on the impacts of mobile fishing gear such as trawls, and their impacts on seafloor soft-bottom ecosystems (Auster et al., 1996; Watling and Norse, 1998; Collie et al., 2000; Kaiser et al., 2003; Simpson and Watling, 2006), hard-bottom habitats (Enrichetti et al., 2019), and especially corals (Freese et al., 1999). Few, if any, studies have examined the role of fixed gear such as traps or pots on benthic ecosystems.
Traps are one of the most commonly used types of fishing gear, especially in fisheries for crabs, lobsters, shrimp, crayfish, fish, whelks, and conchs (Figure 1). A primary benefit of using traps for catching crustaceans is that they can be highly species specific, due to variations in size, shape, mesh size, and the design of entries and exits. Thus, the volume and diversity of bycatch can usually be reduced. With some exceptions, most traps are small enough that they can be easily handled by one or two people, and most can be easily stacked, stored, or moved.
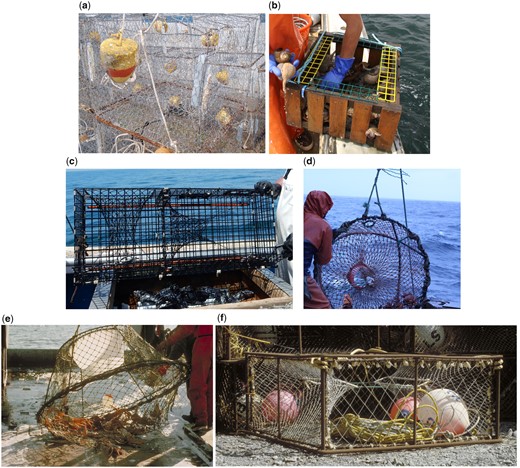
Traps for species commonly caught in the United States: (a) Blue crab; (b) Channeled whelk (Conch); (c) Black sea bass or American lobster; (d) Red deep-sea crab; (e) Tanner Crab; and (f) Red king crab. All photos by author.
Probably the most serious environmental problem caused by crustacean traps is entanglement with marine mammals. While this is of concern to management agencies for population viability, it is also increasingly seen by the public as a threat to animal welfare in general, and to the sustainability of seafood harvests as well (Moore and van der Hoop, 2012). Although the major population threat of commercial whaling has been largely removed, the growth of recreational whale watching to an industry worth $2 Billion per annum has increased public interest in whale conservation (Knowlton et al., 2016).
The goal of this discussion is to highlight research on the impacts of traps on physical and biological habitat components, methods to reduce or eliminate such impacts, and current and future directions in designs of traps for the fishing of crustaceans and other species.
Hierarchy of impacts
Before undertaking a study on the impacts of trap fishing, it is valuable to identify potential impacts and categorize them. The impacts of trap fishing can first be divided among systems, i.e. impacts on captured organisms (both target and non-target) vs. those on the environment, including non-captured and non-target species (Figure 2). A second level further separates impacts on catch into target (retained) and non-target (discarded) organisms, and impacts on environment among physical and biological categories, including benthic/epibenthic and pelagic organisms (mostly whales). Direct impacts on any of these are either reduced population size of target-retained catch, or mortality of non-target and bycatch-discarded species. Indirect impacts include changes in rates of reproduction, feeding or growth, genetic selection, or predator-prey relationships, as well as loss of habitat structure that supports hiding, feeding, or mating refugia.
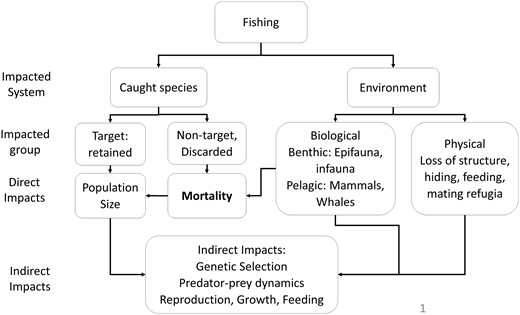
Some of these impacts have measurable economic value, such as bycatch of target and non-target commercial species, and some have unmeasurable economic impacts, including impacts to habitats, epifauna, and whales. Measuring the value of such impacts, whether economic or not, requires a series of value judgements and binary comparisons, and perceived value depends on the background and societal role of the evaluator. Using an analytic hierarchy process (AHP), Innes and Pascoe (2010) studied the impacts of mobile fishing gear by asking stakeholders to rank various binary pairs of impacts, e.g. bycatch of commercial fish vs. that of commercial invertebrates. Fishers and gear technologists tended to assign the greatest value to reductions in commercial fish discarding, and much less to reducing mortality of invertebrates (commercial or not) or habitat change, whereas ecologists, economists, and managers tended to assign equal value to reducing discards and habitat damage (Innes and Pascoe, 2010). Value differences between fishers and scientists are partially due to their concepts of environmental “health”; fishers tend to view “health” of an environment in terms of the amount of a target species produced, whereas scientists tend to view environmental health in terms of the diversity of species, habitats, and services supported (Innes and Pascoe, 2010).
Impacts of trap fishing on benthic ecosystems
Fishing with traps has both benefits and impacts. One of the primary benefits is that trap catches are highly specific, with little bycatch of non-target organisms, compared to mobile gear such as trawls or dredges (Stevens, 2014b). Nonetheless, trap fishing for crustaceans and other organisms does have detrimental impacts, including mortality due to handling, discarding, and exposure, all of which have been studied extensively, so are not addressed here (Stevens, 2014b). Similarly, ghost fishing by derelict traps for crabs and lobsters has also been extensively reported (High and Worlund, 1979; Breen, 1987; Stevens et al., 2000; Bullimore et al., 2001; Hébert et al., 2001; Godoy et al., 2003; Adey et al., 2008; Macfadyen et al., 2009; Antonelis et al., 2011; Arthur et al., 2014; Uhrin et al., 2014; Butler and Matthews, 2015). Economic impacts due to ghost fishing have also been examined (Hébert et al., 2001; Antonelis et al., 2011; Arthur et al., 2014; DelBene et al., 2019). Despite the number of studies that focus on the impact of lost pots to captured organisms, few studies have examined the impact of traps on benthic habitats and ecosystems.
In many crustacean fisheries, each trap is attached to a single buoy line. For example, Dungeness crab Metacarcinus magister, blue crab Callinectes sapidus, king crab Paralithodes camtschaticus, Tanner and snow crab Chionoecetes bairdi and Chionoecetes opilio, respectively, and American lobster Homarus americanus are all fished with single-line traps, and most are fished in waters <100 m depth (Figure 1). Fisheries that occur in deeper water, however, often employ strings of traps (often called “trawls”, “fleets”, or “rigs”) attached to a single ground line with one buoy line at each end. The fishery for red deep-sea crab Chaceon quinquedens occurs at depths of 500–700 m and uses 100–200 traps attached to each ground line (author’s personal observation). The fishery for golden king crabs Lithodes aequispina in the Aleutian Islands employs strings of 10–90 pots, with the whole string weighing in excess of 30 t (Stone and Shotwell, 2007). Other non-crab fisheries also use strings of traps; fishers targeting black sea bass Centropristis striata in the Mid-Atlantic region of the US typically attach 10–20 traps to each “rig” (Schweitzer et al., 2018) and deep-water fisheries for American lobster use up to 50 traps per trawl (Partan and Ball, 2016).
The importance of structure
Structural complexity is an important characteristic of habitats used by crustaceans and other species. Juvenile red king crabs are highly dependent on the presence of complex structural habitats that may be biogenic, e.g. mussels, bryozoans, and worm colonies (Sundberg and Clausen, 1977; McMurray et al., 1986; Stevens and MacIntosh, 1991; Stevens, 2003) or physical (shell hash and cobble) (Armstrong et al., 1985; Tapella et al., 2009), and some of these habitats are associated with vertically emergent epifauna such as the ascidian Boltenia ovifera (Stevens, 2014a). Seamounts in the Gulf of Alaska and other locations are prime locations for biogenic emergent epifauna, including corals and sponges (Hoff and Stevens, 2005), and are home to at least ten species of large crabs including king crabs (Lithodes spp.), spider crabs (Chionoecetes spp., Macroregonia macrocheira), and others (Stevens, 2002). Some of these structures are important for reproduction as well. During dives in the submersible DSV Alvin on the upper slopes of Alaskan seamounts, grasping pairs, i.e. in pre-mating embrace, of scarlet king crabs L. couesi were observed attached to both the inside and outside of large vase sponges (author’s personal observation). Grasping pairs of golden king crabs Lithodes aequispinus have been observed associated with the understory of Primnoa spp. coral colonies in the Gulf of Alaska (Krieger and Wing, 2002). In sandy-mud habitats such as those in the Bering Sea where most fishing for red king crab occurs, crabs also associate with other organisms that do not provide much apparent shelter. In muddy substrata, Tanner crabs C. bairdi have been observed “backed up” to large pink anemones Cribrinopsis fernaldi as well as various types of marine debris (especially tires; authors personal observation), and several species of caridean shrimps shelter beneath the tentacles of these same anemones (Stevens and Anderson, 2000).
Structured habitats are also important to non-crustacean species. In the Mid-Atlantic Bight, CPUE of black sea bass caught by hook and line was three times greater, and video CPUE (mean number per sampled frame) was 2–50 times greater on rock and live bottom habitats than on bare sand (Cullen and Stevens, 2017; Cullen and Stevens, 2020). In the Gulf of Alaska, 97% of juvenile rockfish (Sebastes spp.) and 96% of juvenile golden king crabs were associated with emergent epifauna such as large gorgonians, and the crabs were commonly observed within the spongocoel of the hexactinellid sponges Aphrocallistes vastus and Heterochone calyx (Stone, 2006). During submersible dives in the Gulf of Alaska, Krieger and Wing (2002) observed that 85% of all large rockfish were associated with corals.
Impacts of traps on seafloor habitats
Most studies of fishing impacts have focused on mobile gear such as trawls and dredges, which can have significant impacts on biogenic habitats ranging from the removal of emergent epifauna to completely resorting the underlying substrata (Freese et al., 1999; Kaiser et al., 2003; van Denderen et al., 2014). In contrast, few studies have examined the impact of pot or trap fishing, which is generally assumed to have little impact on benthic habitats (Eno et al., 2001). The greatest potential for damage occurs where traps are set in rocky habitats that are home to corals, sponges, sea whips, crinoids and many other large emergent epifauna, some of which may be important prey items for crustaceans. Fisheries for king crabs in the Aleutian Islands, Pribilof Islands, and Southeast Alaskan fjords are most likely to encounter such habitats. Setting of traps onto live or emergent epifauna may cause damage by crushing or breaking them, especially corals and sponges. In soft sediments, the most likely impact is the removal of emergent epifauna such as cnidarians, ascidians (e.g. Boltenia), or anemones. Brittle stars and crinoids are common on seamounts and breakage or damage to them could make them available to other predators, reducing their abundance.
During submersible dives in the Aleutian Islands, disturbance to the seafloor was observed on 22 of 25 transects, affecting ∼38.6% of the seafloor, but was primarily due to trawls and longlines (with hooks) (Stone, 2006). Disturbance from crab pot longlines was observed on one dive over a span of 147 m (Stone, 2006). At a nearby site, the seafloor was scoured bare of epifauna (including corals, gorgonians, sponges, and crinoids) along 17 strips, ranging from 2 to 9 m in width, possibly the result of crab pots being dragged in parallel across the seafloor. Multiple observations at depths between 61 and 337 m showed that 8.5% of all corals observed were damaged including 20.2% of hydrocorals and 7.4% of gorgonians (Stone, 2006). Pots for golden king crab are connected with 25 mm or larger diameter polypropylene lines that may range from 3 to 9 km in length (Stone and Shotwell, 2007). Stone and Shotwell (2007) observed that “… under certain conditions the [longline trap] gear can be dragged like a plough across the seafloor”. Gorgonians (Primnoa spp.) were reported to disappear in an area where prawn Pandalus platyceros pots were set because of coral entanglement in the mesh of the pots. This is most likely to happen when fishing in strong winds and currents.
Eno et al. (2001) studied the impacts of Norway lobster creels on benthic habitats using cameras and divers. Creels were fished in small fleets of 3–5 traps laid in parallel lines. The likelihood of a creel landing on top of sea pens (Virgularia sp., Funiculina sp., and Pennatula sp.) was estimated to be 2.3% based on density and fishing effort. As the creel landed, the pressure wave often caused the sea pen to bend away from the trap, preventing major damage. Divers dragged creels across the seafloor and noted that sea pens that were uprooted or smothered usually reburied or recovered within 144 h (Eno et al., 2001). On hard-bottom substrata, some species of sponges actually increased in abundance after traps were set and recovered, but this may have been an artefact of increased detectability or reduced cover (Eno et al., 2001). Some damage to large colonies of Ross coral Pentapora foliacea was evident; these are large, slow-growing colonies for which recovery could be slow. While these impacts appear to be minor or temporary, it is difficult to determine the long-term cumulative impact from repeated fishing on either the habitat or the target species.
In the New England and mid-Atlantic regions of the United States, standard wire traps are used to catch American lobster, Jonah crab Cancer borealis, and black sea bass, depending on the shape of the escape port. Traps are fished in “rigs” of 20 traps per line, and fishers primarily target small patches of hard-bottom reef with emergent epifauna. The gorgonian sea whip Leptogorgia virgulata is one of the largest and most prominent components of benthic epifauna in this region, and its presence is associated with abundance of black sea bass (Schweitzer and Stevens, 2019). Schweitzer et al. (2018) studied the impact of trap fishing on benthic habitats in the offshore waters of Maryland and Delaware, at depths of 25–35 m, by placing cameras on traps, and fishing them under standard commercial operating procedures. Only 5% of traps encountered live epibenthic organisms on deployment, but the likelihood of such encounters increased to 50% during retrieval, as traps were dragged across the seafloor, during which they encountered sea whips, corals, seastars, or other organisms. Encounters with sea whip corals included running over them, which can cause breakage, damage to external tissues, or removal (Schweitzer et al., 2018). Drag time and number of epifaunal encounters (hits) increased with the sequential position of traps on the line, ranging from ca. 10 s for the first trap retrieved to a mean of 60 s for the last trap (# 20) (Figure 3). While epifaunal contact generally increased with position on the line, the relationship between hits and drag time was inconsistent, possibly due to “clearing” of the seafloor by previous traps (Schweitzer et al., 2018). Movement of traps during extended soak times was not assessed, but it is known that traps can be displaced by storms (W. Townsend, pers. comm.). Such movements may also cause damage to epifauna either by direct contact with traps or the ground lines connecting them. Fishers often use grappling gear to recover lost traps, which can add another source of damage, due either to the grapple, or the recovered traps and lines (Sheridan et al., 2003).
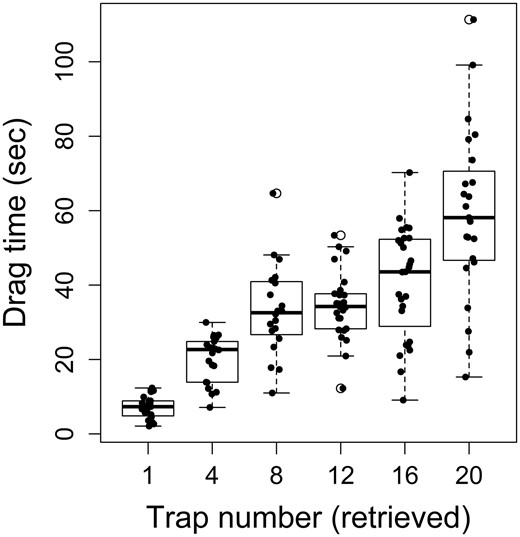
Drag time of traps on a multi-trap line, as a function of retrieval order. Redrawn from data in Schweitzer et al. (2018).
Dragging of traps during recovery probably occurs due to misalignment of the fishing vessel with the gear. The first trap is located close to the first surface buoy, so fishers can more easily position the boat near it for recovery. Following this, the fisher attempts to move the boat towards the next trap to be recovered, balancing his estimate of distance between traps with surface current conditions (personal observation). This is an “inexact science”, and dragging occurs due to a number of conditions. The boat may not move towards the next trap at the same speed that the hydraulic block pulls the trap towards the boat; the boat may move faster, overshooting the trap position; the distance between traps is less than the depth, so that as each trap is recovered, it pulls the next one (or more) off bottom before the boat moves to that position. This mismatch increases with depth of the fishing gear; during recovery of red deep-sea crabs from depths of 600 m or more, up to 10 traps may be suspended in the water at any time during haulback.
Shester and Micheli (2011) examined the impacts of lobster traps and gill nets on seafloor habitats in Baja California (Mexico). Divers simulated fishing by dropping individual traps (size and shape not described) onto gorgonian corals, which caused minor damage (<1% of body tissue affected) in 1 of 37 (3%) trials. Because fishers commonly drag traps across the seafloor for short distances during setting, divers also simulated this behaviour by dragging traps by hand. Gorgonian corals were impacted during 40% of these trials (but the sample size was not stated), causing damage to ca. 5% of body tissue, but not causing detachment. In contrast, set gillnets impacted 22% of gorgonians and removed 17% of gorgonians, within 1 m of the net. Despite the use of presumably smaller and lighter traps, and shorter drag events, these results are similar to the estimate of 50% impact (of any organism) of Schweitzer et al. (2018) when dragging is included. Impacts to gorgonians were probably underestimated by Shester and Micheli (2011) because divers cannot generate the forces produced by a moving boat with a winch, which are more likely to cause gouging of the seafloor and breakage of corals. If they had also studied trap recoveries (with associated dragging), the incidence of coral impacts would probably have been much greater than 40%.
Schweitzer et al. (2018) used an ROV to examine sea whips at three sites near the experimental fishing site for signs of damage, including necrosis and overgrowth by hydroids and bryozoans. Sea whips at all sites were damaged, with a mean 37% of the area of 22 colonies showing damage. In contrast, damage indices of sea whips examined by scuba divers at locations that were lightly or moderately fished by recreational fishers (using hook and line) ranged from 0.02 to 0.26 and increased with habitat patch size (Schweitzer and Stevens, 2019). Although fishing effort was not assessed, these results suggest that damage may be related to the size of the habitat patches and thus ease of locating them, leading to increased effort. These two studies are not directly comparable, however, since damage from trap impacts and fishing line entanglement is different, but they do indicate that a significant proportion of emergent epifauna show signs of impact where fishing occurs, although comparable unfished sites were not examined.
Stephenson et al. (2017) used a before–after/control–impact design to study the impacts of pot fishing (“potting”) on rocky bottom habitats off the coast of Northumberland. Quadrat photos were used to estimate percent cover of organisms before and after experimental sets of single pots on the seafloor, and repeated three times. Community structure changed similarly at both the experimental and non-fished control sites, indicating no impact from fishing, and sensitivity analysis showed that the method could detect a 5% change (Stephenson et al., 2017). The authors concluded that potting was unlikely to cause changes in benthic community structure. Their methods, however, involved pulling up single pots, rather than pots on long lines, which tend to drag across the seafloor causing more damage (Eno et al., 2001; Schweitzer et al., 2018). Furthermore, most of the species present were low-relief species adapted to disturbance and the authors stated that “…rocky reef habitats in Northumberland may already be in a degraded state due to decades of fishing” (Stephenson et al., 2017).
Surveys of traps for Caribbean spiny lobster Panulirus argus showed that about 50% of traps in the US Virgin Islands caused damage to the underlying substrata, but the extent of damage covered only about 3.3% of the underlying area for traps in the Florida Keys (Sheridan et al., 2005). Species most affected were sponges and gorgonians, with less impact on hard corals. Barnette (2001) reported that several studies found 82–86% of lobster traps were set upon live substrate (including seagrasses, hard corals, or soft corals), often killing submerged aquatic vegetation (SAV) and creating “haloes” around the gear; dislocation of vase sponges by traps could result in complete deterioration. One Dungeness crab trap set in an eelgrass bed caused the destruction of the eelgrass immediately beneath it and created a 1 m × 1.8 m scour hole 0.3 m deep behind it that was also devoid of eelgrass (June and Antonelis, 2009), thus creating an impact footprint three times the size of the trap. In La Parguera, Puerto Rico, 44% of lobster traps were set on hard bottom or reef, causing damage to corals, gorgonians, and sponges, including flattening and breakage, which can lead to subsequent death, overgrowth, or disease infection (Appeldoorn et al., 2000). During haulback, 30% of lobster traps inflicted additional damage to live substrata. Lines connecting multiple traps on a string can also inflict damage, and the cumulative impact of connecting lines can exceed that of the traps.
In addition to being sources of disturbance, traps can be an ecological distraction for some species. In the Mediterranean, cuttlefish are known to lay eggs on traps used to catch them and most such eggs are destroyed when the traps are recovered and cleaned (Melli et al., 2014). In Maryland, we have also observed fish/lobster traps covered with squid eggs (Figure 4). They probably suffer little harm if the traps are fished and returned to the water quickly, but if cleaned or removed, they may constitute a non-viable sink for squid reproduction.
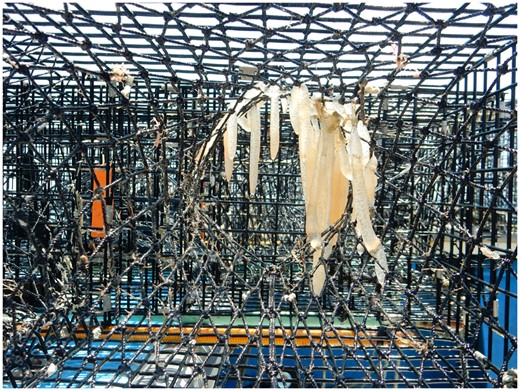
Fish/lobster trap with squid eggs, an example of distraction disturbance (B. Stevens).
Impacts of derelict traps
Although habitat damage may occur during setting and retrieval of traps, damage may also occur due to lost traps that move or disintegrate after loss. Bycatch in lost “ghost” traps has received the greatest attention, but little attention has been paid to other types of disturbance.
After loss, traps can remain in place and intact for extensive periods, exceeding 400 days for crab traps in Wales (Bullimore et al., 2001). Condition of king and Tanner crab pots was classified as “poor” or “worse” for 33% of 133 derelict pots recovered in Kodiak, Alaska, indicating that they had probably been lost for >2 years (Stevens et al., 2000). The effective fishing life of traps was estimated to be 2.2 years for Dungeness crab traps (Breen, 1987), and up to 15 years for king crab traps (High and Worlund, 1979), whereas Stevens (2014b) hypothesized that fishing ability would decline exponentially and estimated the effective half-life of a king crab trap at ca. 4 years. In a survey of Florida reef sites, lobster traps and associated debris accounted for 63–78% of all anthropogenic debris encountered (Uhrin et al., 2014), and density of ghost and non-fishing traps was 2.7 ha−1. Density of derelict king and Tanner crab traps in Chiniak Bay, Alaska, was estimated by side-scan sonar to be 0.42 ha−1 in 1996, and numbers of “effective” ghost pots in the Bering Sea were predicted to stabilize between 40 000 and 1.7 million depending on assumptions about loss and degradation rates (Stevens et al., 2000). For comparison, the density of actively fished lobster traps in Maine was estimated at 1.7–7.5 traps·ha−1 (Kelly, 1993).
Traps do not always remain in the area where they were lost. Derelict spiny lobster traps and debris were most abundant in coral reef habitats despite the fact that the most intensive fishing occurs in seagrass habitats; weather and boat traffic probably contributed more to the distribution of debris than direct loss (Uhrin et al., 2014). Fish/lobster traps have been observed entangled and abutted against shipwreck sites during scuba dives in Maryland (author’s personal observation), even though commercial fishers try to avoid setting traps in such locations due to the potential for loss.
Traps that remain on the seafloor can move, and such movements can create scouring of substrate in the immediate area. Lobster traps fished for several days in the Florida Keys caused damage to a mean of 3.27 organisms (primarily stony corals, octocorals, and sponges) and cumulative damaged surface area on organisms was 198 cm2 per trap (Lewis et al., 2009). Buoyed traps moved when sustained surface winds exceeded 7.7 m s−1 for 2 d or more; mean distance moved ranged from 0.6 to 3.6 m at depths from 12 to 4 m, respectively, with maximum movement of 30 m. The footprint of a lobster trap is ca. 0.6 m2, but movement during storms increased that by 1–2 orders of magnitude; in one case a trap impacted 9.5 m2 of seafloor, or 16 times its own footprint (Lewis et al., 2009). The authors estimated that ∼1 million lobster and crab traps were used annually in south Florida. Clark et al. (2012) reported that experimental fish traps set in <7 m of water in the US Virgin Islands were moved from 3 to 150 m during a hurricane, although damage to the substrate was not detected, and some traps were moved and never found. Surveys such as this probably underestimate actual damage, as broken or detached organisms may be swept away by currents and not observed. Estimates of lobster trap loss range from 10 to 20% per year, but losses were reported to be ca. 60% during the hurricane season of 2005. Due to the high numbers of traps deployed and history of the fishery (>50 years), it is likely that traps have significantly affected the structure of coral reefs and hard-bottom ecosystems in the Florida Keys (Lewis et al., 2009). A survey of marine debris in the Florida Keys revealed that remnants of lobster traps accounted for 64% of the stony corals impacted, 22% of gorgonians, and 29% of sponges (Chiappone et al., 2002). Crab traps in the Bering Sea have occasionally been dragged considerable distances when buoys became trapped in surface sea ice. Such dragging can scour seafloor sediments or impact other crabs during the dragging process. Even though the footprint of individual traps may be small, repeated retrieval and setting of traps in the same areas and the large number of derelict traps in certain locations probably exacerbate these impacts and contribute to increased levels of damage to benthic organisms and habitats (Guillory et al., 2001).
Programmes to recover lost traps have been implemented in certain regions with varying rates of success. Traps are often recovered by dragging a grappling hook, which can inflict further damage if done on hard-bottom or coral habitats (Barnette, 2001). The 189 traps detected in an area of 4.5 km2 in Alaska by Stevens et al. (2000) probably represented only a small fraction of traps in the local vicinity. A few thousand traps were recovered by dedicated programmes in Texas and Mississippi before funding was cut; 4600 traps were voluntarily collected in North Carolina in 1995, but these represented <2% of the 250 000 traps that are reported to be lost annually (Guillory et al., 2001). A dedicated recovery programme in the Virginia portion of the Chesapeake Bay recovered 32 000 traps over 4 years, but still a fraction of the estimated 50 000 traps lost in that region annually (Havens et al., 2008; Bilkovic et al., 2014). Heavy colonization of some traps by oysters prompted the authors to suggest that leaving disabled traps in place might actually be beneficial for structure-oriented organisms. During 5 years of trap-removal efforts in Biscayne National Park, recovery rates did not decline with successive search days either within or between years, despite inter-annual differences in methods used (Martens and Huntington, 2012). The authors concluded that the supply of derelict traps, either annually, or due to historic accumulation, exceeded the ability to remove them on an annual basis. A variety of localized trap-removal projects have been supported by the NOAA Marine Debris Program, but the effectiveness of these has yet to be evaluated (https://marinedebris.noaa.gov/current-efforts/removal). The conclusion that must be drawn from most of these efforts is that recovery programmes are not particularly successful solutions to the problem of trap loss, because they tend to be poorly funded, active only for short time periods, and cannot keep up with annual rates of loss.
Impacts to different types of habitat-forming organisms are summarized in Table 1, and given arbitrary values of 0, 1, or 2, depending on whether such impacts were rated as absent, low, or high, respectively, by their authors. Soft corals, including gorgonians and pennatulaceans, are most often cited as being impacted, though impacts are typically low (mean 1.22). Impacts to stony corals and sponges were less often cited, but ranked higher (1.29 and 1.33, respectively). Impacts to motile epifauna and macroalgae were cited rarely and ranked lowest (0.5 and 1.0, respectively). Impacts to SAV were ranked high in two studies, giving that habitat the highest mean impact level of 2.0. The number of citations for each habitat-organism most likely reflects the amount of concern for those particular habitats, rather than the amount of fishing at those locations. Although many crustacean fisheries (e.g. snow crab) take place in unstable, unconsolidated sediments (e.g. sand or mud), this author found no studies on the impacts of trap fishing to those habitats.
Studies reporting impacts of trap or pot fishing gear on benthic habitats and organisms, indicating trap type, region, and type of organism.
Source . | Trap type . | Region . | Soft corals . | Stony corals . | Sponges . | Motile epifauna . | SAV . | Macroalgae . |
---|---|---|---|---|---|---|---|---|
Stone (2006) | King crab | Alaska | 1 | 1 | – | – | – | – |
Stone and Shotwell (2007) | King crab | Alaska | 1 | – | – | – | – | – |
Eno et al. (2001) | Nephrops | Scotland | 1 | 1 | 1 | – | – | – |
Stephenson et al. (2017) | “Pots” | North Sea | – | – | – | 0 | – | – |
June and Antonelis (2009) | Dungeness crab | Washington | – | – | – | – | 2 | – |
Schweitzer et al. (2018) | Black sea bass | Maryland | 2 | 1 | 1 | – | – | |
Shester and Micheli (2011) | Spiny lobster | Mexico | 1 | – | – | – | – | 1 |
Sheridan et al. (2005) | Spiny lobster | Florida | 1 | 1 | 1 | – | – | – |
Barnette (2001) | Spiny lobster | Florida | – | – | 2 | – | 2 | – |
Appeldoorn et al. (2000) | Spiny lobster | Puerto Rico | 2 | 2 | 2 | – | – | – |
Chiappone et al. (2002) | Spiny lobster (derelict) | Florida | 1 | 2 | 1 | – | – | – |
Lewis et al. (2009) | Spiny lobster (derelict) | Florida | 1 | 1 | 1 | – | – | – |
Count | 9 | 7 | 6 | 2 | 2 | 1 | ||
Sum | 11 | 9 | 8 | 1 | 4 | 1 | ||
Mean | 1.22 | 1.29 | 1.33 | 0.50 | 2.00 | 1.00 |
Source . | Trap type . | Region . | Soft corals . | Stony corals . | Sponges . | Motile epifauna . | SAV . | Macroalgae . |
---|---|---|---|---|---|---|---|---|
Stone (2006) | King crab | Alaska | 1 | 1 | – | – | – | – |
Stone and Shotwell (2007) | King crab | Alaska | 1 | – | – | – | – | – |
Eno et al. (2001) | Nephrops | Scotland | 1 | 1 | 1 | – | – | – |
Stephenson et al. (2017) | “Pots” | North Sea | – | – | – | 0 | – | – |
June and Antonelis (2009) | Dungeness crab | Washington | – | – | – | – | 2 | – |
Schweitzer et al. (2018) | Black sea bass | Maryland | 2 | 1 | 1 | – | – | |
Shester and Micheli (2011) | Spiny lobster | Mexico | 1 | – | – | – | – | 1 |
Sheridan et al. (2005) | Spiny lobster | Florida | 1 | 1 | 1 | – | – | – |
Barnette (2001) | Spiny lobster | Florida | – | – | 2 | – | 2 | – |
Appeldoorn et al. (2000) | Spiny lobster | Puerto Rico | 2 | 2 | 2 | – | – | – |
Chiappone et al. (2002) | Spiny lobster (derelict) | Florida | 1 | 2 | 1 | – | – | – |
Lewis et al. (2009) | Spiny lobster (derelict) | Florida | 1 | 1 | 1 | – | – | – |
Count | 9 | 7 | 6 | 2 | 2 | 1 | ||
Sum | 11 | 9 | 8 | 1 | 4 | 1 | ||
Mean | 1.22 | 1.29 | 1.33 | 0.50 | 2.00 | 1.00 |
Impact levels are denoted as absent (0), low (1), or high (2). Count of studies reporting impacts, sum of impact levels, and mean impact level are shown.
Studies reporting impacts of trap or pot fishing gear on benthic habitats and organisms, indicating trap type, region, and type of organism.
Source . | Trap type . | Region . | Soft corals . | Stony corals . | Sponges . | Motile epifauna . | SAV . | Macroalgae . |
---|---|---|---|---|---|---|---|---|
Stone (2006) | King crab | Alaska | 1 | 1 | – | – | – | – |
Stone and Shotwell (2007) | King crab | Alaska | 1 | – | – | – | – | – |
Eno et al. (2001) | Nephrops | Scotland | 1 | 1 | 1 | – | – | – |
Stephenson et al. (2017) | “Pots” | North Sea | – | – | – | 0 | – | – |
June and Antonelis (2009) | Dungeness crab | Washington | – | – | – | – | 2 | – |
Schweitzer et al. (2018) | Black sea bass | Maryland | 2 | 1 | 1 | – | – | |
Shester and Micheli (2011) | Spiny lobster | Mexico | 1 | – | – | – | – | 1 |
Sheridan et al. (2005) | Spiny lobster | Florida | 1 | 1 | 1 | – | – | – |
Barnette (2001) | Spiny lobster | Florida | – | – | 2 | – | 2 | – |
Appeldoorn et al. (2000) | Spiny lobster | Puerto Rico | 2 | 2 | 2 | – | – | – |
Chiappone et al. (2002) | Spiny lobster (derelict) | Florida | 1 | 2 | 1 | – | – | – |
Lewis et al. (2009) | Spiny lobster (derelict) | Florida | 1 | 1 | 1 | – | – | – |
Count | 9 | 7 | 6 | 2 | 2 | 1 | ||
Sum | 11 | 9 | 8 | 1 | 4 | 1 | ||
Mean | 1.22 | 1.29 | 1.33 | 0.50 | 2.00 | 1.00 |
Source . | Trap type . | Region . | Soft corals . | Stony corals . | Sponges . | Motile epifauna . | SAV . | Macroalgae . |
---|---|---|---|---|---|---|---|---|
Stone (2006) | King crab | Alaska | 1 | 1 | – | – | – | – |
Stone and Shotwell (2007) | King crab | Alaska | 1 | – | – | – | – | – |
Eno et al. (2001) | Nephrops | Scotland | 1 | 1 | 1 | – | – | – |
Stephenson et al. (2017) | “Pots” | North Sea | – | – | – | 0 | – | – |
June and Antonelis (2009) | Dungeness crab | Washington | – | – | – | – | 2 | – |
Schweitzer et al. (2018) | Black sea bass | Maryland | 2 | 1 | 1 | – | – | |
Shester and Micheli (2011) | Spiny lobster | Mexico | 1 | – | – | – | – | 1 |
Sheridan et al. (2005) | Spiny lobster | Florida | 1 | 1 | 1 | – | – | – |
Barnette (2001) | Spiny lobster | Florida | – | – | 2 | – | 2 | – |
Appeldoorn et al. (2000) | Spiny lobster | Puerto Rico | 2 | 2 | 2 | – | – | – |
Chiappone et al. (2002) | Spiny lobster (derelict) | Florida | 1 | 2 | 1 | – | – | – |
Lewis et al. (2009) | Spiny lobster (derelict) | Florida | 1 | 1 | 1 | – | – | – |
Count | 9 | 7 | 6 | 2 | 2 | 1 | ||
Sum | 11 | 9 | 8 | 1 | 4 | 1 | ||
Mean | 1.22 | 1.29 | 1.33 | 0.50 | 2.00 | 1.00 |
Impact levels are denoted as absent (0), low (1), or high (2). Count of studies reporting impacts, sum of impact levels, and mean impact level are shown.
Recovery of damaged habitats
Understanding the long-term impacts of fishing on marine benthic habitats requires an understanding of the processes of recovery, recruitment, and regrowth. Recovery periods for substrata and epibenthic organisms that are impacted by trap fishing can range from hours to centuries. Semi-motile organisms such as sea pens that were uprooted or smothered by fishing activities were able to rebury within 144 h (Eno et al., 2001). Sea whips L. virgulata that were damaged by fish traps (Schweitzer et al., 2018) have estimated lifetimes of 15–20 years but appear to recruit sporadically at decadal intervals, possibly following substrate clearance by major storms (Wenker and Stevens, 2020). Thus, insular populations on small habitat patches, if removed or damaged by repeated fishing, may not recover to pre-fishing age distributions for decades. Corals living in deeper water or on seamounts live to extreme ages, ranging from 140 years for Antipatharian corals from 100 m depth in California (Love et al., 2007) to 320 years for Primnoa resedaeformis from 450 m near George’s Bank (Risk et al., 2002). Colonies of Lophelia pertusa can be hundreds of years old and individual reefs may have accumulated over 50 000 years (Stone and Shotwell, 2007). Although many other coral species also reach advanced age, the species listed here are commonly impacted by traps set for lobster, crab, or fish. While live corals are highly valued for their contribution to structured habitats for fish and other organisms, standing dead corals are also valuable habitats; Love et al. (2007) counted 2554 individual invertebrates living on a single dead colony of Antipathes dendrochristos.
Grabowski et al. (2014) conducted a meta-analysis of 97 studies on impacts of different fishing gear on benthic habitats, but only one (the previously cited Eno et al., 2001) addressed impacts of traps. Susceptibility of biological features to damage and estimated recovery times were deemed higher in granule–pebble–boulder habitats than in mud or sand habitats, and estimated recovery times for biological communities were estimated to be lower for damage caused by traps than for other types of gear. It was not possible to distinguish impacts of traps from that of longlines and gillnets, however, due to lack of studies (Grabowski et al., 2014). Furthermore, impacts on corals were specifically excluded, because they were not considered endemic to the New England marine shelf ecosystem. Grabowski et al. (2014) stated that the ability to detect impacts of fixed gear would benefit from “a greater understanding of the effective footprint of each fixed gear [and] the degree to which these gears drift and are dragged”. Experimental studies have shown that recovery of disturbed marine benthic ecosystems is dependent on many factors, including size of the habitat patch, similarity to surrounding habitats, season, and hydrodynamic connection to patches with similar community composition (Thrush et al., 2013). Fishing of individual traps may represent a short-term (pulse) impact, but it is commonly considered that long-term (press) impacts will have more serious consequences. Even after impacts are removed, by the end of fishing seasons, for example or redistribution of fishing effort to alternative locations, disturbed ecosystems may never recover to fully functional status. Compared to their initial states, ecosystems recovering from disturbance (including marine ecosystems) run mean annual deficits or “recovery debt” equivalent to 50% of organism abundance, and 30% of species diversity, regardless of the ecological biome (Moreno-Mateos et al., 2017). Four months after removal of a derelict crab pot, the adjacent scour hole had filled in with sediment, and new eelgrass shoots had spread from the surrounding bed into 30% of the affected area, but no further change had occurred after 8 months (June and Antonelis, 2009). Even artificially restored or recreated ecosystems have lower abundance and diversity than undisturbed ecosystems, and “complete recovery” of ecosystem structure and function may take many decades or may never occur (Moreno-Mateos et al., 2017). For this reason, it is both ecologically and economically more sensible to protect habitats from disturbance rather than try to restore them with lower-quality systems.
Impacts of trap fishing on pelagic ecosystems
The impacts of traps on benthic habitat have received little attention, and thus few efforts at mitigation. In contrast, impacts of traps on pelagic organisms including marine mammals, pinnipeds, otters, and turtles have stimulated a variety of research into methods for reducing entrapment and entanglement (Hamilton and Baker, 2019). In South Australia, entrapment of pinnipeds in pots for southern rock lobster Jasus edwardsii is a major issue; Mackay and Goldsworthy (2017) showed that collars on the entrances were more effective at preventing entry than spikes that were previously required. Diamondback terrapin Malaclemys terrapin, especially juveniles, are often captured in blue crab traps, and estimates of overall mortality due to trap bycatch range from 15 to 78% of the terrapin population (Roosenburg, 2004; Dorcas et al., 2007). Modifications in shape or size of crab traps to prevent terrapin mortality were impractical, but bycatch reduction devices (BRDs), consisting of square collars placed on the entrance to blue crab traps, were found to be more effective (Roosenburg, 2004). In one study, BRDs reduced the bycatch of terrapins by a factor of 20, while increasing mean size and biomass of crabs caught, relative to traps without BRDs (Rook et al., 2010). California sea otters Enhydra lutris nereis are often captured in traps for lobster, crabs, and fish, but the extent of the problem is unknown due to the lack of observers or mandatory reporting (Hatfield et al., 2011; Worton et al., 2016). Otter entrapment can be prevented by a slight reduction in the size of the trap opening without reducing crab catch (Hatfield et al., 2011). Sea ducks and muskrats have also been found in blue crab traps (Bilkovic et al., 2014).
The larger problem, however, is due to the entanglement of cetaceans, primarily whales. Entanglement with fishing gear, primarily lobster and crab trap lines, causes 82% of whale mortalities (Baumgartner et al., 2019; Moore, 2019) and is second only to vessel strikes as a cause of death for North Atlantic right whales (NARW) (Vanderlaan et al., 2011). The Atlantic Large Whale Take Reduction Team (ALWTRT) has estimated that 85% of NARW have been entangled at least once, and 26% of the population are entangled every year (ALWTRT, 2019). Methods for mitigating marine mammal entanglement in fishing gear have been reviewed by a number of authors (How et al., 2015; Laverick et al., 2017; Hamilton and Baker, 2019), but it is not my intent to review them all here. Proposed solutions fall into three categories including: (i) modifying fishing gear; (ii) reducing the amount or number of lines in the water (i.e. effort); and (iii) reducing rates of encounter between mammals and fishing gear by use of time/area closures (Moore, 2019).
The primary sources of the problem are floating buoy lines associated with trap fisheries (Moore, 2019) and floating ground lines connecting multiple traps. Despite requirements for weak links and/or sinking ground lines on lobster and fish traps in the Northeast, this has not produced a decrease in entanglements (Moore, 2019). Weak links are not practical for the deep-water American lobster fishery, which typically fishes in depths to 300 m, with vertical lines of up to 900 m in length, and up to 50 traps per trawl (Partan and Ball, 2016). In addition, the requirement for weak links may have led fishers to use stronger ropes for hauling more traps. Furthermore, when activated, weak links allow the whale to break free while still entangled, and free roaming whales are much harder to find and disentangle than whales still attached to fishing gear. Acoustic pingers have also been suggested as a way of alerting whales to the presence of fishing gear, but field tests have not shown this to be an effective mechanism (How et al., 2015).
To reduce impacts on whales, fishers in the Northeast United States have been required to reduce the number of vertical lines on lobster and fish traps. In April 2019, the Atlantic States Marine Fisheries Council lobster board voted to reduce the number of vertical lines by 20–40% for each Lobster Catch Management Area (LCMA) exclusive of area 6, and in October, 2019, the Atlantic Large Whale Take Reduction Team (ALWTRT) recommended a 50% reduction in vertical lines within the Maine lobster fishery, and a 30% reduction in Massachusetts and New Hampshire (ALWTRT, 2019). A 50% reduction in Maine would amount to ca. 11 miles of rope for individual boats. Myers et al. (2007), however, estimated that the Maine lobster population is overexploited by a factor of 50 and should be able to catch the same number of lobsters with the number of traps reduced by a factor of 10, and the season by 50%. Reducing vertical lines requires connecting multiple traps to a single groundline with only two vertical lines at either end. Floating ground lines were initially used to reduce entanglement on rocks, but regulations now require these to be replaced with weighted, or sinking, groundlines to further reduce whale entanglement, but increasing the amount of bottom contact and subsequent disturbance.
The problem of floating lines on individual buoys, or at the end of strings or “trawls” of multiple traps, still remains to be solved. Equipment designed to reduce the amount of vertical lines and floating buoys is being developed in a number of directions (Myers et al., 2007; Moore and van der Hoop, 2012; Baumgartner et al., 2019; Myers et al., 2019). Ropeless trap fishing systems have been designed that release a trap buoy either after a specified time by use of a simple GTR (Jankiewicz, 1981) or on demand via an acoustic release (Baumgartner et al., 2019; Myers et al., 2019). Tests of potential methods for reducing whale entanglements in the Western rock lobster (Jasus spp.) fishery of New Zealand were conducted with nine fishers, and results evaluated based on perceived lowest cost and greatest practicality (How et al., 2015). Acoustic pingers were the preferred alternative, followed by modifications to ropes (e.g. sinking or biodegradeable), whereas the least preferred method was using bottom-stored ropes released by anode or acoustic release mechanisms (though only one trial of the latter occurred). All of these mechanisms failed on some occasions, resulting in lost traps. At present, there are no published studies on the effectiveness or potential costs/benefits of using ropeless traps.
One example of a ropeless fishing gear recovery system is the Edgetech Model 5112 Ropeless Fishing System (RFS) (https://www.edgetech.com/fisheries-science-underwater-research/), consisting of a release cage integrating the acoustic release, rope, and flotation that can be attached to a single trap, trawl, or longline, and a “Trap Tracker” application for IOS and Android to control the system (Figure 5a). The RFS is rated to 500 m depth but is not yet permitted for use in fishing activities. Current cost is US$2500 for the deck control unit (only one required) and US$3750 for each release unit. The Ropeless Consortium (Baumgartner et al., 2019) has developed a design for an “on-call” release mechanism, consisting of a large 154 kg (340 lb) spool that floats just above bottom, and contains up to 600 m of retrieval line. A prototype design was developed by The Consortium for Wildlife Bycatch Reduction specifically to meet the needs of the offshore, deep-water American lobster fishery (Partan and Ball, 2016). It includes 900 m of 12-mm (1/2 inch) line (allowing a 3:1 scope) on a spool with 180 lbs of flotation that encloses a central release mechanism using a timer to activate a motor-driven release (Partan and Ball, 2016). This prototype system uses a simple clock-driven release (to reduce cost and improve reliability) but could be upgraded in the future to incorporate a more expensive acoustic release. It also incorporates the ability to provide encrypted identification information in response to an authorized query. In efforts to encourage whale-safe fishing, the California Department of Fish and Wildlife held a “Demonstration Day” on 6 December 2019, during which six companies demonstrated products designed to avoid whale entanglement to a group of fishers and regulators (CDFW, 2019). Products demonstrated included pop-up buoys, buoy tracking systems, delayed-release bait containers, and acoustic release mechanisms for traps.
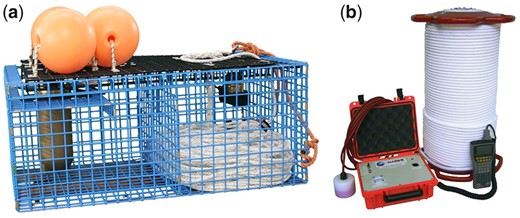
(a) Edgetech Ropeless fishing system (Edgetech Corp., Wareham, MA, USA; https://www.edgetech.com/fisheries-science-underwater-research/). (b) Fiobuoy AC-200 trap release system (FioBuoy, FioMarine, Australia; http://www.fiomarine.com/).
At present, most ropeless fishing systems are too expensive for widespread adoption. In addition, deployment of ropeless or buoyless fishing systems has been inhibited due to reluctance on the part of NOAA to accept it as a viable alternative to traditional buoys (ALWTRT, 2019). Use of ropeless systems is supported by conservation groups, however, and proposals have been made to develop a transition plan by requiring their adoption among the American lobster fishery in high-risk areas within 5 years, and everywhere else within 10 years, although these have not been approved or implemented (ALWTRT, 2019).
While ropeless fishing systems have obvious advantages for whales, they may also solve some of the problems associated with benthic impacts of traps, although their ability to mitigate such impacts is currently unknown. The ability to retrieve individual pots remotely would reduce the amount of groundline in contact with benthic habitats, thus reducing scouring and damage to emergent epifauna. It also seems likely that retrieval of individual traps is less likely to cause dragging than retrieval of trap “trawls” or long rigs, as evidenced by shorter drag times for the first trap retrieved (Schweitzer et al., 2018). With better positioning equipment, it may be possible to locate each individual trap on the chart plotter; this could help prevent overlaying traps by other fishers, and subsequent trap loss; it could also allow keeping the vessel more closely aligned with the trap during recovery in order to prevent dragging. Development of sensors to measure trap content, e.g. density of catch, would be extremely useful and could allow fishers to adjust deployment time to optimize use of resources and fuel. Sector scanning sonar can detect the presence and density of crabs, and this could even be deployed on some traps or associated sensor equipment (unpublished data; Figure 6). Although increasing catches or catch rates has its own ramifications, it could improve the efficiency and profitability of fishing, thus requiring less effort, and subsequently fewer environmental impacts.
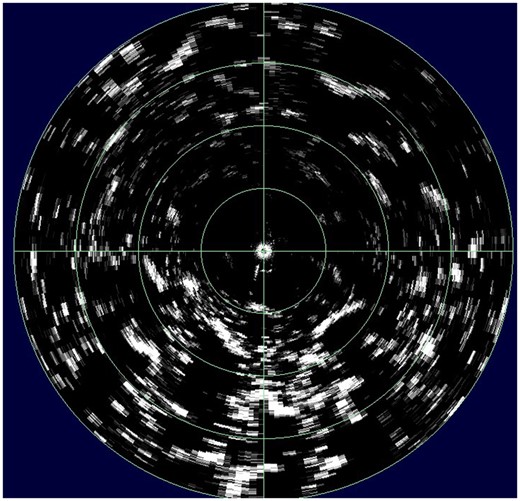
Sector scanning sonar image of Tanner crabs Chionoecetes bairdi in Chiniak Bay, Alaska, taken from a remotely operated vehicle, with transducer located 0.5 m off bottom. Radius is 10 m, with 2.5 m between concentric circles. Small white spots are individual male crabs; larger “blotches” are aggregations of females.
Acoustic modems used for on-demand recovery could also be adapted to provide information such as the owner’s name, trap ID number, and deployment position that would be available to other fishers or enforcement agencies, enabling proper enforcement of fishing regulations (Baumgartner et al., 2019). Additional sensors could capture information such as depth, temperature, or trap occupancy and encrypt it so that it would only be accessible by the owner. Trap modems could be surveyed by enforcement vessels, robotic gliders, or passing vessels, so that location and movements of the traps could be monitored, and any change in position could be reported to the owner. The modem could double as a release mechanism, triggered by an acoustic signal from the owner’s vessel, and encoded to a specific user. Recovery methods could include bottom stowed rope, variable buoyancy traps, air-inflated lift bags, and/or trap docking systems. Benefits of such technology include reductions in lost gear and lost catch due to trap movement or broken lines; reduced impacts on emergent epifauna due to dragging or relocation of traps; reduction in ghost fishing; elimination of ropes and entanglement of mammals; reduced conflict between gear; and improved safety due to elimination of the requirement to haul ropes under tension and associated equipment.
Conclusions, recommendations, and future innovations
In addition to impacts on fished populations, stationary gear (traps and pots) has direct impacts on habitat and other organisms in the immediate environment. Such impacts occur while deployed, during recovery, and after loss of contact with the gear. Soft corals, particularly gorgonians, are most often cited as being impacted, though impacts to stony corals, sponges, and SAV may be greater. The impact of trap contact with the bottom is generally confined to a fraction of the trap footprint, but dragging of the trap during retrieval, by ice, or storms can expand the footprint by an order of magnitude. Ongoing development of strategies and modifications to reduce entanglement of marine mammals in floating line is currently driving innovation in trap design, and some variation in buoyless or ropeless systems will probably be required and implemented in the near future in US waters.
Habitat damage caused during the retrieval of traps is poorly explored or understood but could be doing long-term harm to habitats that support the targeted populations. Methods for retrieving traps quickly without dragging have not yet been developed but would be extremely useful for fisheries such as lobster and black sea bass, where traps are set in habitat characterized by abundant live organisms. Such solutions might involve modifying the length of “snood” lines connecting traps to the groundline, or increasing the distance between the traps as depth increases. Future innovations could use sensors to adjust the buoyancy of the traps, via inflatable bladders, or use water current indicators to automatically reposition traps. In the future, “Smart traps” could perhaps even adjust their own position, using water currents to follow environmental gradients or crab aggregations to improve their catches.
The need to reduce environmental impacts will drive the evolution of trap and buoy design in the near future. Although habitat impacts are hidden from both fishers and the public, the impacts to marine mammals are well known and pose an ethical threat to sustainability of trap fisheries, and thus deserve serious consideration. The designation of “Whale-safe” will become especially important if fishers want to avoid consumer boycotts of their fisheries, akin to the “dolphin-tuna” controversy that led to passage of the Dolphin Protection Consumer Information Act (16 U.S.C. 1385) in 1990 (US Congress, 1990). It is also necessary for fisheries to maintain seafood certification by groups such as the Marine Stewardship Council (Moore and van der Hoop, 2012).
The future of trap fishing
In a hypothetical future scenario, a fishing boat might deploy a fleet of independent “smart” traps, along with additional “commander” modules, containing a suite of environmental sensors, including sonar. After landing on the bottom, instruments on the commander module would measure the water temperature, current speed, and direction and determine tidal timing and velocity. Sensors on each trap would relay their catch information to the commander. Marine mammals swimming above or around the traps would be completely immune to entanglement. After several hours of fishing, the commander modules would determine that catch rates had levelled off, but sonar data may indicate that more crabs and better fishing conditions were present 50 m to the west of the fleet. Using measured current patterns, the commander would signal the entire fleet of traps to increase buoyancy bladders just enough to lift them slightly off the bottom and enable them to drift with the tide to the new location, passing over epibenthic organisms without disturbing them, before settling to the bottom. When sensors indicate that catch levels were optimal, the commander would signal the ship to come retrieve them and then trigger all traps to become buoyant in sequence, arriving at the surface exactly in time to be recovered. Such a scenario would result in greatly improved economic and fuel efficiency, reduced contact with the bottom or benthic epifauna, minimal or no dragging of traps, and virtually no impact to marine mammals. In other words, a great improvement over current fishing methods.
Acknowledgements
Salary for B. Stevens was partially supported by NOAA grant NA16SEC4810007 to the Living Marine Resources Cooperative Science Center at University of Maryland Eastern Shore. Reference to trade names or commercial firms does not imply endorsement by NOAA. The scientific results and conclusions, as well as any views or opinions expressed herein, are those of the author(s) and do not necessarily reflect those of NOAA or the Department of Commerce.
References
ALWTRT.
CDFW.
US Congress.