-
PDF
- Split View
-
Views
-
Cite
Cite
W. Christopher Long, Katherine M. Swiney, Robert J. Foy, Effects of high pCO2 on Tanner crab reproduction and early life history, Part II: carryover effects on larvae from oogenesis and embryogenesis are stronger than direct effects, ICES Journal of Marine Science, Volume 73, Issue 3, February/March 2016, Pages 836–848, https://doi.org/10.1093/icesjms/fsv251
- Share Icon Share
Abstract
Anthropogenic CO2 release is increasing the pCO2 in the atmosphere and oceans and causing a decrease in the pH of the oceans. This decrease in pH, known as ocean acidification, can have substantial negative effects on marine life. In this study, we use wild-brooded larvae and larvae from females held in treatment pH for two brooding cycles over 2 years to detect carryover effects from oogenesis and embryogenesis. Ovigerous females were held at three pHs: ∼8.1 (Ambient), 7.8, and 7.5. Exposure to acidified conditions at the larval stage alone had minimal effects on the larvae, possibly because larvae may be adapted to living in an environment with large pH swings. Exposure of Tanner crab larvae to low pH during the embryo phase had a more substantial effect on morphology, size, Ca/Mg content, and metabolic rate than exposure during the larval phase, and maternal exposure during the oogenesis phase increased the carryover effect. Although the larval phase itself is resilient to low pH, carryover effects are likely to have a negative effect on larvae in the wild. These results, combined with negative effects of high pCO2 at other life history stages, indicate that high pCO2 may have a negative effect on the Tanner crab populations and fisheries soon.
Introduction
Anthropogenic activities are increasing the concentration of CO2 in the atmosphere and ocean and are predicted to reduce ocean pH of ∼0.3 units by 2100 and 0.5 units by 2200 (Caldeira and Wickett, 2003). High-latitude areas are expected to be affected sooner than other areas. For example, in North Pacific waters, a number of factors interact to affect the severity of ocean acidification. CO2 is more soluble in the colder water that exists at high-latitude waters, and thus uptake from the atmosphere is more rapid at the surface, while upwelling (particularly in the Gulf of Alaska) brings up CO2-rich waters from the deep (Fabry et al., 2009). Ice melt from sea ice and glaciers, exacerbated by increased temperatures, can reduce the saturation state of calcium carbonate minerals, particularly in surface waters (Evans et al., 2014; Mathis et al., 2014). These factors, combined with stratified waters and seasonal, high rates of carbon export from the surface layers during periods of high productivity, can lead to pCO2 in bottom waters exceeding 1600 ppm in the late summer and early fall (Mathis et al., 2014). Surface waters are predicted to become, on average, undersaturated with respect to aragonite by 2070 in Bering Sea (Mathis et al., 2015).
Ocean acidification is expected to have a physiological effect on many marine animals, particularly on those with calcium carbonate shells or exoskeletons (Fabry et al., 2008). Although crustaceans are among the many calcifying marine organisms, their response to ocean acidification is mixed (Wittmann and Pörtner, 2013). Even at early life history stages, such as the embryo and larval stages, a wide response is seen, although these developmental stages are thought to be more sensitive to stressors. The larvae of some crustacean species have reduced survival (Walther et al., 2010; Long et al., 2013b) and growth (Keppel et al., 2012; Schiffer et al., 2014) or exhibit deformities (Agnalt et al., 2013) under acidified conditions, although other species are resilient to such changes (Styf et al., 2013; Small et al., 2015).
Because the larval stage is typically only a small fraction of the life history of most crustaceans and other marine invertebrates, exposure to ocean acidification during this stage is relatively brief. However, carryover effects from previous stages may be important. Carryover effects occur when exposure to a stressor at one life history stage affects performance in a subsequent stage. For example, juvenile Olympia oysters, Ostrea lurida, suffer reduced growth after settlement if exposed to low pH during the larval phase regardless of the pH juveniles are exposed to (Hettinger et al., 2012, 2013). The larval phase of both the spider crab, Hyas araneus, and red king crab, Paralithodes camtschaticus, suffers greater mortality following exposure to acidified water as embryos (Long et al., 2013a; Schiffer et al., 2014). Thus, understanding carryover effects is critical for our understanding of how ocean acidification will affect a particular species.
The Tanner crab, Chionoecetes bairdi, is a commercially important crab species in Alaskan waters (NPFMC, 2011). Primiparous (first egg clutch) Tanner crab females molt to maturity, mate, and extrude a batch of eggs in the late winter (Paul and Adams, 1984), whereas multiparous, brooding a second or subsequent clutch, release their larvae typically in April to May and then mate, and extrude new eggs right thereafter (Munk et al., 1996). Eggs are brooded for about a year before larval hatching (Swiney, 2008); during that year, oogenesis in the female ovaries is preparing oocytes for the next year's clutch. Larvae spend ∼3 months in the plankton as they pass through a prezoeal stage which lasts only a few hours (Haynes, 1973), followed by two zoeal and a megalops stage which each last about a month, before settling into the benthic environment, and molting to the first crab stage (Incze et al., 1982).
The waters at the high latitudes where Tanner crabs live are expected to acidify more rapidly than elsewhere (Fabry et al., 2009). Seasonal variability in the pH of bottom water in Bering Sea results in pH levels that are physiologically stressful (pH < 7.8) to juvenile Tanner crabs for at least part of the year (Cross et al., 2013). Juvenile Tanner crabs exhibit decreased growth, survival, and calcification under near-future levels of CO2 (Long et al., 2013b); adults suffer increased haemocyte mortality and a decreased intracellular pH in semi-granular and granular haemocytes (Meseck et al., 2015). Given the sensitivity of this species to high pCO2, it is imperative to understand its effects at all life history stages; different life history stages may differ in their response (e.g. Hettinger et al., 2012). In addition, because of the year-long duration of both oogenesis and embryogenesis, it is critical to understand potential carryover effects because direct effects alone may substantially underestimate the cumulative effects of high pCO2 exposure (Hettinger et al., 2012, 2013; Schiffer et al., 2014).
To determine how high pCO2 is likely to affect the Tanner crab stocks, we performed a series of experiments examining a wide range of life history stages and responses. In an initial study, we determined how high pCO2 affected juvenile Tanner crab growth, survival, calcification, and condition (Long et al., 2013b). This paper is the second in a two-part series that examines the effects of high pCO2 on reproduction and early life history stages. In the first paper, we determine the effects of high pCO2 on embryogenesis, larval hatching success, and ovigerous Tanner crabs (Swiney et al., 2016). In this paper, we examine the effects of high pCO2 on the starvation-survival, carbon and nitrogen content, and calcium/magnesium content of larval Tanner crabs. By using larvae from embryos reared in the first experiment, we examine carryover effects to the larval stage from exposure during oogenesis and embryogenesis. Understanding how both the direct and carryover effects of high pCO2 affect larval fitness is critical for understanding how these stocks may respond to ocean acidification.
Methods
Overview
To determine the effects of high pCO2 on larval Tanner crabs, we performed three sets of experiments over 3 years, 2011–2013 (overall experimental design is depicted in Supplementary material S1). In 2011, we used wild-brooded larvae to determine the effects on the larval stage only. We then held females with newly extruded embryos in the laboratory at three pH treatments, ambient and two experimental pH levels (pH 7.8 and pH 7.5), until the larvae hatched and, in 2012, we used these larvae to determine carryover effects of exposure at the embryo stage on larvae using a fully crossed design between embryo and larval treatments. Finally, we held the females for another year in their pH treatments. So, in 2013, the larvae that hatched out came from oocytes that developed in the females while they were in treatment water and embryos that had developed in treatment water. These larvae allowed us to determine carryover effects of exposure during both oogenesis and embryogenesis on larvae, again via a fully crossed experimental design. All larval experiments were performed on the first zoeal stage and no molting was observed during any of the experiments.
Water acidification
Water was acidified using CO2 as described in Long et al. (2013a) and Swiney et al. (2016) to produce water with pHs of 7.8 and 7.5 (expected pHs in ∼2100 and 2200). pH and temperature in experimental units were measured daily using a Durafet II or III pH probe during all experiments. Water samples to monitor experimental conditions were taken once a week, from the head tanks (2011), or from the larval experimental tanks (2012 and 2013; see details on set-up below) poisoned with mercuric chloride, and sent to the NOAA's Auke Bay Laboratories (2011 and 2012) or to the University of Alaska Fairbanks (2013) for analysis of salinity, dissolved inorganic carbon (DIC), and total alkalinity (TA) as described in Swiney et al. (2016). Samples sizes (total) were 6 in 2011, 16 in 2012, and 13 in 2013. The seacarb package in R (V2.14.0, Vienna, Austria) was used to calculate the pCO2, carbonate, and bicarbonate concentrations, and the saturation state of calcite and aragonite (Lavigne and Gattuse, 2012).
The pH in the larval experiments stayed well within the nominal range in all 3 years (Table 1). Temperatures were slightly (∼0.4°C) lower in 2012 than in 2013, and ∼2°C lower in 2011 than 2012 reflecting differences in the ambient incoming seawater. pCO2 increased with decreasing pH. Ambient water pH and pCO2 differed slightly among the years and was always above saturation for both aragonite and calcite, pH 7.8 water was undersaturated with respect to aragonite, and pH 7.5 water was undersaturated with respect to both aragonite and calcite. Full water chemistry parameters in the female/embryo treatments, presented elsewhere (Swiney et al., 2016), were similar to the conditions in these larval experiments with pHs ± 1 standard deviation (s.d.) of 8.09 ± 0.07, 7.80 ± 0.03, and 7.50 ± 0.03 in the Ambient, pH 7.8, and pH 7.5 treatments.
Average water chemistry parameters in experimental units (±1 standard deviation) during experiments on larval Tanner crabs for each of the 3 years.
Treatment . | Temperature (°C) . | pHF . | pCO2 (µatm) . | HCO3− (mmol kg−1) . | (mmol kg−1) . | DIC (mmol kg−1) . | Alkalinity (mmol kg−1) . | ΩAragonite . | ΩCalcite . |
---|---|---|---|---|---|---|---|---|---|
2011 | |||||||||
Ambient | 4.66 ± 0.19 | 8.21 ± 0.04 | 269.42 ± 20.45 | 1.81 ± 0.01 | 0.12 ± 0.01 | 1.95 ± 0.00 | 2.13 ± 0.01 | 1.88 ± 0.09 | 3.00 ± 0.14 |
pH 7.8 | 4.61 ± 0.13 | 7.79 ± 0.02 | 810.33 ± 22.89 | 1.97 ± 0.00 | 0.05 ± 0.00 | 2.06 ± 0.00 | 2.10 ± 0.01 | 0.76 ± 0.03 | 1.21 ± 0.05 |
pH 7.5 | 4.63 ± 0.17 | 7.49 ± 0.02 | 1665.48 ± 161.70 | 2.02 ± 0.02 | 0.03 ± 0.00 | 2.14 ± 0.01 | 2.09 ± 0.02 | 0.39 ± 0.03 | 0.62 ± 0.05 |
2012 | |||||||||
Ambient | 6.85 ± 0.66 | 8.16 ± 0.04 | 345.66 ± 34.66 | 1.87 ± 0.01 | 0.11 ± 0.01 | 2.00 ± 0.01 | 2.16 ± 0.06 | 1.70 ± 0.13 | 2.71 ± 0.21 |
pH 7.8 | 6.58 ± 0.43 | 7.81 ± 0.02 | 787.30 ± 21.50 | 2.02 ± 0.02 | 0.06 ± 0.00 | 2.11 ± 0.02 | 2.17 ± 0.05 | 0.85 ± 0.03 | 1.36 ± 0.04 |
pH 7.5 | 6.69 ± 0.50 | 7.50 ± 0.02 | 1643.71 ± 91.51 | 2.03 ± 0.04 | 0.03 ± 0.00 | 2.14 ± 0.05 | 2.23 ± 0.14 | 0.42 ± 0.02 | 0.66 ± 0.04 |
2013 | |||||||||
Ambient | 7.01 ± 0.55 | 8.16 ± 0.03 | 326.53 ± 33.75 | 1.85 ± 0.01 | 0.12 ± 0.01 | 1.98 ± 0.01 | 2.12 ± 0.01 | 1.76 ± 0.16 | 2.81 ± 0.25 |
pH 7.8 | 7.12 ± 0.59 | 7.79 ± 0.01 | 811.36 ± 38.31 | 1.99 ± 0.02 | 0.05 ± 0.00 | 2.09 ± 0.02 | 2.09 ± 0.02 | 0.81 ± 0.04 | 1.29 ± 0.07 |
pH 7.5 | 7.06 ± 0.54 | 7.50 ± 0.02 | 1619.80 ± 59.85 | 2.05 ± 0.02 | 0.03 ± 0.00 | 2.15 ± 0.02 | 2.13 ± 0.01 | 0.43 ± 0.02 | 0.69 ± 0.03 |
Treatment . | Temperature (°C) . | pHF . | pCO2 (µatm) . | HCO3− (mmol kg−1) . | (mmol kg−1) . | DIC (mmol kg−1) . | Alkalinity (mmol kg−1) . | ΩAragonite . | ΩCalcite . |
---|---|---|---|---|---|---|---|---|---|
2011 | |||||||||
Ambient | 4.66 ± 0.19 | 8.21 ± 0.04 | 269.42 ± 20.45 | 1.81 ± 0.01 | 0.12 ± 0.01 | 1.95 ± 0.00 | 2.13 ± 0.01 | 1.88 ± 0.09 | 3.00 ± 0.14 |
pH 7.8 | 4.61 ± 0.13 | 7.79 ± 0.02 | 810.33 ± 22.89 | 1.97 ± 0.00 | 0.05 ± 0.00 | 2.06 ± 0.00 | 2.10 ± 0.01 | 0.76 ± 0.03 | 1.21 ± 0.05 |
pH 7.5 | 4.63 ± 0.17 | 7.49 ± 0.02 | 1665.48 ± 161.70 | 2.02 ± 0.02 | 0.03 ± 0.00 | 2.14 ± 0.01 | 2.09 ± 0.02 | 0.39 ± 0.03 | 0.62 ± 0.05 |
2012 | |||||||||
Ambient | 6.85 ± 0.66 | 8.16 ± 0.04 | 345.66 ± 34.66 | 1.87 ± 0.01 | 0.11 ± 0.01 | 2.00 ± 0.01 | 2.16 ± 0.06 | 1.70 ± 0.13 | 2.71 ± 0.21 |
pH 7.8 | 6.58 ± 0.43 | 7.81 ± 0.02 | 787.30 ± 21.50 | 2.02 ± 0.02 | 0.06 ± 0.00 | 2.11 ± 0.02 | 2.17 ± 0.05 | 0.85 ± 0.03 | 1.36 ± 0.04 |
pH 7.5 | 6.69 ± 0.50 | 7.50 ± 0.02 | 1643.71 ± 91.51 | 2.03 ± 0.04 | 0.03 ± 0.00 | 2.14 ± 0.05 | 2.23 ± 0.14 | 0.42 ± 0.02 | 0.66 ± 0.04 |
2013 | |||||||||
Ambient | 7.01 ± 0.55 | 8.16 ± 0.03 | 326.53 ± 33.75 | 1.85 ± 0.01 | 0.12 ± 0.01 | 1.98 ± 0.01 | 2.12 ± 0.01 | 1.76 ± 0.16 | 2.81 ± 0.25 |
pH 7.8 | 7.12 ± 0.59 | 7.79 ± 0.01 | 811.36 ± 38.31 | 1.99 ± 0.02 | 0.05 ± 0.00 | 2.09 ± 0.02 | 2.09 ± 0.02 | 0.81 ± 0.04 | 1.29 ± 0.07 |
pH 7.5 | 7.06 ± 0.54 | 7.50 ± 0.02 | 1619.80 ± 59.85 | 2.05 ± 0.02 | 0.03 ± 0.00 | 2.15 ± 0.02 | 2.13 ± 0.01 | 0.43 ± 0.02 | 0.69 ± 0.03 |
Temperature and pH (free scale) were measured daily, DIC (all years) and alkalinity (2012 and 2013 only) were measured weekly, and all other parameters were calculated from pH and DIC.
Average water chemistry parameters in experimental units (±1 standard deviation) during experiments on larval Tanner crabs for each of the 3 years.
Treatment . | Temperature (°C) . | pHF . | pCO2 (µatm) . | HCO3− (mmol kg−1) . | (mmol kg−1) . | DIC (mmol kg−1) . | Alkalinity (mmol kg−1) . | ΩAragonite . | ΩCalcite . |
---|---|---|---|---|---|---|---|---|---|
2011 | |||||||||
Ambient | 4.66 ± 0.19 | 8.21 ± 0.04 | 269.42 ± 20.45 | 1.81 ± 0.01 | 0.12 ± 0.01 | 1.95 ± 0.00 | 2.13 ± 0.01 | 1.88 ± 0.09 | 3.00 ± 0.14 |
pH 7.8 | 4.61 ± 0.13 | 7.79 ± 0.02 | 810.33 ± 22.89 | 1.97 ± 0.00 | 0.05 ± 0.00 | 2.06 ± 0.00 | 2.10 ± 0.01 | 0.76 ± 0.03 | 1.21 ± 0.05 |
pH 7.5 | 4.63 ± 0.17 | 7.49 ± 0.02 | 1665.48 ± 161.70 | 2.02 ± 0.02 | 0.03 ± 0.00 | 2.14 ± 0.01 | 2.09 ± 0.02 | 0.39 ± 0.03 | 0.62 ± 0.05 |
2012 | |||||||||
Ambient | 6.85 ± 0.66 | 8.16 ± 0.04 | 345.66 ± 34.66 | 1.87 ± 0.01 | 0.11 ± 0.01 | 2.00 ± 0.01 | 2.16 ± 0.06 | 1.70 ± 0.13 | 2.71 ± 0.21 |
pH 7.8 | 6.58 ± 0.43 | 7.81 ± 0.02 | 787.30 ± 21.50 | 2.02 ± 0.02 | 0.06 ± 0.00 | 2.11 ± 0.02 | 2.17 ± 0.05 | 0.85 ± 0.03 | 1.36 ± 0.04 |
pH 7.5 | 6.69 ± 0.50 | 7.50 ± 0.02 | 1643.71 ± 91.51 | 2.03 ± 0.04 | 0.03 ± 0.00 | 2.14 ± 0.05 | 2.23 ± 0.14 | 0.42 ± 0.02 | 0.66 ± 0.04 |
2013 | |||||||||
Ambient | 7.01 ± 0.55 | 8.16 ± 0.03 | 326.53 ± 33.75 | 1.85 ± 0.01 | 0.12 ± 0.01 | 1.98 ± 0.01 | 2.12 ± 0.01 | 1.76 ± 0.16 | 2.81 ± 0.25 |
pH 7.8 | 7.12 ± 0.59 | 7.79 ± 0.01 | 811.36 ± 38.31 | 1.99 ± 0.02 | 0.05 ± 0.00 | 2.09 ± 0.02 | 2.09 ± 0.02 | 0.81 ± 0.04 | 1.29 ± 0.07 |
pH 7.5 | 7.06 ± 0.54 | 7.50 ± 0.02 | 1619.80 ± 59.85 | 2.05 ± 0.02 | 0.03 ± 0.00 | 2.15 ± 0.02 | 2.13 ± 0.01 | 0.43 ± 0.02 | 0.69 ± 0.03 |
Treatment . | Temperature (°C) . | pHF . | pCO2 (µatm) . | HCO3− (mmol kg−1) . | (mmol kg−1) . | DIC (mmol kg−1) . | Alkalinity (mmol kg−1) . | ΩAragonite . | ΩCalcite . |
---|---|---|---|---|---|---|---|---|---|
2011 | |||||||||
Ambient | 4.66 ± 0.19 | 8.21 ± 0.04 | 269.42 ± 20.45 | 1.81 ± 0.01 | 0.12 ± 0.01 | 1.95 ± 0.00 | 2.13 ± 0.01 | 1.88 ± 0.09 | 3.00 ± 0.14 |
pH 7.8 | 4.61 ± 0.13 | 7.79 ± 0.02 | 810.33 ± 22.89 | 1.97 ± 0.00 | 0.05 ± 0.00 | 2.06 ± 0.00 | 2.10 ± 0.01 | 0.76 ± 0.03 | 1.21 ± 0.05 |
pH 7.5 | 4.63 ± 0.17 | 7.49 ± 0.02 | 1665.48 ± 161.70 | 2.02 ± 0.02 | 0.03 ± 0.00 | 2.14 ± 0.01 | 2.09 ± 0.02 | 0.39 ± 0.03 | 0.62 ± 0.05 |
2012 | |||||||||
Ambient | 6.85 ± 0.66 | 8.16 ± 0.04 | 345.66 ± 34.66 | 1.87 ± 0.01 | 0.11 ± 0.01 | 2.00 ± 0.01 | 2.16 ± 0.06 | 1.70 ± 0.13 | 2.71 ± 0.21 |
pH 7.8 | 6.58 ± 0.43 | 7.81 ± 0.02 | 787.30 ± 21.50 | 2.02 ± 0.02 | 0.06 ± 0.00 | 2.11 ± 0.02 | 2.17 ± 0.05 | 0.85 ± 0.03 | 1.36 ± 0.04 |
pH 7.5 | 6.69 ± 0.50 | 7.50 ± 0.02 | 1643.71 ± 91.51 | 2.03 ± 0.04 | 0.03 ± 0.00 | 2.14 ± 0.05 | 2.23 ± 0.14 | 0.42 ± 0.02 | 0.66 ± 0.04 |
2013 | |||||||||
Ambient | 7.01 ± 0.55 | 8.16 ± 0.03 | 326.53 ± 33.75 | 1.85 ± 0.01 | 0.12 ± 0.01 | 1.98 ± 0.01 | 2.12 ± 0.01 | 1.76 ± 0.16 | 2.81 ± 0.25 |
pH 7.8 | 7.12 ± 0.59 | 7.79 ± 0.01 | 811.36 ± 38.31 | 1.99 ± 0.02 | 0.05 ± 0.00 | 2.09 ± 0.02 | 2.09 ± 0.02 | 0.81 ± 0.04 | 1.29 ± 0.07 |
pH 7.5 | 7.06 ± 0.54 | 7.50 ± 0.02 | 1619.80 ± 59.85 | 2.05 ± 0.02 | 0.03 ± 0.00 | 2.15 ± 0.02 | 2.13 ± 0.01 | 0.43 ± 0.02 | 0.69 ± 0.03 |
Temperature and pH (free scale) were measured daily, DIC (all years) and alkalinity (2012 and 2013 only) were measured weekly, and all other parameters were calculated from pH and DIC.
2011 wild-brooded larvae
Ethical approval for this research was not required by any federal, state, or international laws because the study was conducted on invertebrates not covered under these laws. Healthy, multiparous, ovigerous female Tanner crabs were captured in Chiniak Bay, Kodiak, Alaska, in the spring of 2011 in crab pots and a 3 m beam trawl. Four healthy females with clutches of eyed eggs, average carapace width (CW) ± s.d. of 93.3 ± 3.1 mm were held in individual 68 l tubs with flow-through ambient water without food for 5 d, after which larvae for the experiments were collected in a single day in water baths and pooled. The larvae were held at ambient pH and temperature for 24 h before beginning the experiments, and only healthy, actively swimming larvae were used. Experiments were performed in PVC inserts with mesh bottoms placed inside beakers, with the tops of beakers covered with a piece of plastic bubble wrap to reduce gas exchange with the atmosphere as described in Long et al. (2013a). The same three pH treatments as above were used (Ambient, pH 7.8, and pH 7.5). The beakers were kept in a cold room at 5°C, which was ambient temperature of incoming seawater at the time, and water was changed once a day by moving each insert from its old beaker to a beaker of new treatment water. The pH and temperature were measured in each insert each day. Two experiments were performed to determine the effects of high pCO2 on larval (i) morphology and (ii) survival under starvation conditions. For each experiment, there were five replicates of each treatment.
Larval morphology experiments were performed in 2 l PVC inserts/beakers. Approximately 200 larvae were placed in each beaker. Seven larvae were measured initially, before the experiment. Larvae were held for 10 d when three larvae from each beaker were measured (15 per treatment). As the larvae did not molt during the experiment and larvae morphology does not change within a molt stage under normal conditions, we were looking to see if any degradation of the exoskeleton was occurring such as observed by Kurihara et al. (2008). Larvae were photographed under a stereomicroscope and Image-Pro Plus v. 6.00.260 (Media Cybernetics, Inc., Bethesda, MD, USA) was used to measure the carapace width (including spines), lateral spine length, dorsal spine length, rostro-dorsal length, rostral spine length, and protopodite length (Supplementary material S2) as per Webb et al. (2006). Larval morphometrics were analysed using an analysis of similarity (ANOSIM) performed on a Bray–Curtis similarity matrix with pH treatment as the factor in Primer 6.1.13 (Primer, UK).
Larval starvation-survival experiments were performed in 1 l PVC inserts/beakers. Each beaker was stocked with ∼20 larvae. Larvae were checked daily and any larvae that failed to move within a 15 s observation period (based on previous experience with larvae (Long et al., 2013a) and initial observations) were considered dead and discarded. The experiment continued until all larvae were dead. The survival data were fit to a logistic regression model assuming a binomial distribution of data: Pm=1/[1+(t/t50)s], where Pm is the probability of mortality, t the time in days, t50 the time of 50% mortality (sometimes called the LT50) also in days, and s a slope parameter. The t50 parameter indicates how long on average a larvae survives. The slope parameter is proportional to the slope of the curve at t50 (Long, 2016), larger absolute values of s indicate that most of the individual mortality events occurred close to t50, smaller absolute values indicate a greater spread in individual survival times around t50. In this paper, the t50 parameter is the parameter of interest as we want to know if and how the pH treatment affects the survival time; s is necessary for the model but is not interpreted directly. We constructed a series of a priori models in which t50 or s was allowed to vary among the pH treatments and fit them to the data in R 2.14.0 (Vienna, Austria). Post hoc, we noticed that pH 7.8 and Ambient had similar t50 values, so we included models in which those two treatments differed from the pH 7.5 treatment. We selected the best model using the Akaike's information criterion corrected for small sample size (AICc). Models whose AICc differed by <2 were considered to explain the data equally well (Burnham and Anderson, 2002).
2012 and 2013 laboratory-reared larvae
Healthy, multiparous, ovigerous female Tanner crabs, CW 98.7 ± 4.8 mm (s.d.), were captured as described above. Their embryos were either newly extruded (uneyed) or about to hatch (eyed); females which hatched larvae in the lab were allowed to mate and extrude a clutch of eggs in ambient seawater before being placed in the experiment (this occurred within 13 d of capture). Each female was held in an individual container with flow-through seawater. A total of 48 females were used and each was randomly assigned to one of the three pH treatments, for a total of 16 replicates per treatment. Details of the female holding conditions and the effects of high pCO2 on embryo development and Tanner crab fecundity and hatching success are published separately (Swiney et al., 2016). In short, the females were placed in treatment water on 21 June 2011, and held during brooding for the first year. At hatching in May 2012, larvae were collected and used for the 2012 larval experiments. Because the females reach peak hatching at different times, larvae from 2 (Ambient) to 3 (pH 7.8 and 7.5) females within each treatment that were at peak hatching were collected on the same day and used for the experiments. The larvae were pooled and a similar number of larvae were used from each female within each treatment. After hatching, a male crab was placed in with each of the females to allow for mating, and all female extruded a new batch of eggs. Mortality during the first year resulted in 12 females in the Ambient and pH 7.5 treatments and 11 in the pH 7.8 treatment at the beginning of the second brooding year. Females were held in their treatment water until the embryos hatched out in April–June 2013 and were used for the 2013 larval experiments. Larvae from two females from each treatment that were at peak hatching were collected, pooled, and used in the experiments.
In 2012 and 2013, experiments were performed in the same-sized 1 and 2 l PVC inserts as in 2011. These were placed in a tank (one per treatment) with flow-through seawater at the appropriate treatment pH and ambient temperature. The water within the tank was recirculated into each of the inserts. This set-up allowed for better control over the pH, reduced the amount of manipulation the larvae received (no changing of water), and allowed for better water quality (flow-through vs. static conditions). We performed four experiments to determine the effects of high pCO2 on: (i) larval survival, (ii) larval carbon and nitrogen content (CN), (iii) larval calcium and magnesium content, and (iv) larval mass all under starvation conditions to allow interpretation of the survival data in the light of the changing organic and mineral content of the larvae. In 2011, we had planned to run all of these experiments, but the total mass of larvae in each replicate beaker at the end of the experiment was insufficient for the measurement of some of the parameters, so the data could not be collected; this is also why we increased the density of larvae in the experiments and decreased the exposure time from 10 to 7 d. Each of the experiments was fully crossed between embryo treatment and larval treatment, allowing us to examine carryover effects between the stages (Long et al., 2013a), and five replicates were performed for each experiment for each embryo–larval treatment combination. In 2013, however, so few larvae hatched from females in the pH 7.5 treatment (Swiney et al., 2016) that we were only able to perform the starvation survival experiments on these larvae. Before the beginning of the experiments, five samples for CN, calcium/magnesium content, and average larval mass analyses were taken from the starting pool of larvae as described below. In addition, because we did not observe any change in larval morphology after rearing larvae in acidified water in 2011 (see the Results section), we did not examine larval morphology after exposure to acidified waters in 2012 or 2013; instead, we examined if exposure to acidified conditions for a year as embryos affected larval morphology by measuring 15 newly hatched larvae from each treatment as above. Differences in larval morphometrics were analysed using an ANOSIM performed on a Bray–Curtis similarity matrix with embryo treatment as the factor.
Starvation survival experiments were performed in 1 l sized PVC inserts as above until all larvae had died. The data were fit to a series of logistic regressions as above, where the t50 or s parameters were allowed to vary linearly with the embryo and larval treatments. The AICc was calculated for each model and the best model selected. For CN, calcium/magnesium content, and larval mass experiments, ∼300 larvae were stocked in 2 l PVC inserts, held at their treatment pH for 7 d, dried to a constant mass, and then sent to analytical laboratories to be analysed for CN, calcium, and magnesium contents. The CN analysis was performed at the University of California Santa Barbara using the Dumas combustion method and an automated organic elemental analyser (Gnaiger and Bitterlich, 1984). In 2012, calcium/magnesium contents were analysed at the NOAA Auke Bay Laboratory using a Dionex Ion Chromatography system. In 2013, calcium/magnesium contents were analysed at Gel Laboratories via inductively coupled plasma-atomic emission spectrometry. To determine the average mass, subset of 50 larvae was counted, dried, and massed from each replicate PVC insert in both the CN and calcium/magnesium content experiments in 2012 (sample size of 10 per treatment combination), and from each replicate PVC insert in the calcium/magnesium experiments only in 2013 (5 per treatment combination). The average mass of each larva was calculated and the sample was recombined with the remaining larvae from each replicate before CN or calcium/magnesium analysis. The per cent dry mass of carbon, nitrogen, calcium, magnesium, the C:N and Ca:Mg ratios, and the average mass of a larvae were analysed with fully crossed two-way analysis of variance with embryo and larval treatments as factors. Always, the assumption of homogeneity of variance was checked with Levene's test and the data were transformed to meet the assumption, when necessary. When there were significant effects, Tukey's post hoc test was used to detect differences among treatments.
Results
2011 wild-brooded larvae
Larval morphometrics did not vary with treatment (ANOSIM, Global R = 0.009, p = 0.364) and did not change between the beginning and end of the experiment (Figure 1). pH treatment had little to no effect on starvation survival times. Two models fit the survival data equally well: in the first, only the slope parameter varied among the treatments, and in the second, the slope parameter varied among the treatments and the t50 for Ambient and pH 7.8 larvae differed from the pH 7.5 larvae (Table 2). However, the differences among the treatment were slight, with the t50 parameter varying by only 0.18 d, and likely not biologically relevant (Table 2, Figure 2).
Ranking of models of Tanner crab larvae mortality in 2011 using AICc and the parameter estimates ± standard error for the best fit models.
Model . | K . | AICc . | ΔAICc . | Likelihood . | AICc weight . |
---|---|---|---|---|---|
t50, s | 2 | 923.39 | 4.01 | 0.13 | 0.05 |
t50(T), s | 4 | 924.39 | 5.01 | 0.08 | 0.03 |
t50(T2), s | 3 | 922.85 | 3.47 | 0.18 | 0.07 |
t50, s(T) | 4 | 919.38 | 0.00 | 1.00 | 0.40 |
t50(T)s(T) | 6 | 921.79 | 2.41 | 0.30 | 0.12 |
t50(T2)s(T) | 5 | 919.84 | 0.46 | 0.80 | 0.32 |
Parameter estimates | |||||
Parameter | t50, s(T) | t50(T),s(T2) | |||
t50 | 9.47 ± 0.07 | – | |||
t50(A, pH 7.8) | – | 9.54 ± 0.09 | |||
t50 (pH 7.5) | – | 9.36 ± 0.11 | |||
s(A) | −6.18 ± 0.32 | −6.22 ± 0.33 | |||
s(pH 7.8) | −7.12 ± 0.37 | −7.16 ± 0.38 | |||
s(pH 7.5) | −7.6 ± 0.41 | −7.53 ± 0.41 |
Model . | K . | AICc . | ΔAICc . | Likelihood . | AICc weight . |
---|---|---|---|---|---|
t50, s | 2 | 923.39 | 4.01 | 0.13 | 0.05 |
t50(T), s | 4 | 924.39 | 5.01 | 0.08 | 0.03 |
t50(T2), s | 3 | 922.85 | 3.47 | 0.18 | 0.07 |
t50, s(T) | 4 | 919.38 | 0.00 | 1.00 | 0.40 |
t50(T)s(T) | 6 | 921.79 | 2.41 | 0.30 | 0.12 |
t50(T2)s(T) | 5 | 919.84 | 0.46 | 0.80 | 0.32 |
Parameter estimates | |||||
Parameter | t50, s(T) | t50(T),s(T2) | |||
t50 | 9.47 ± 0.07 | – | |||
t50(A, pH 7.8) | – | 9.54 ± 0.09 | |||
t50 (pH 7.5) | – | 9.36 ± 0.11 | |||
s(A) | −6.18 ± 0.32 | −6.22 ± 0.33 | |||
s(pH 7.8) | −7.12 ± 0.37 | −7.16 ± 0.38 | |||
s(pH 7.5) | −7.6 ± 0.41 | −7.53 ± 0.41 |
Model indicates the parameters used and how they were modelled (see text for details). Where factors are included parenthetically, the parameter is modelled as a linear function of those parameters. T indicates that the parameter varies among all treatments and T2 indicates the post hoc models in which the parameter was the same for the Ambient (A) and pH 7.8 treatments which differed from the pH 7.5 treatment. K is the number of parameters for each model. To show the greatest difference among the treatments supported by the data, the more complex t50(T2)s(T) model is presented in Figure 2. The t50 parameters have units of days and the s parameters are unitless.
Ranking of models of Tanner crab larvae mortality in 2011 using AICc and the parameter estimates ± standard error for the best fit models.
Model . | K . | AICc . | ΔAICc . | Likelihood . | AICc weight . |
---|---|---|---|---|---|
t50, s | 2 | 923.39 | 4.01 | 0.13 | 0.05 |
t50(T), s | 4 | 924.39 | 5.01 | 0.08 | 0.03 |
t50(T2), s | 3 | 922.85 | 3.47 | 0.18 | 0.07 |
t50, s(T) | 4 | 919.38 | 0.00 | 1.00 | 0.40 |
t50(T)s(T) | 6 | 921.79 | 2.41 | 0.30 | 0.12 |
t50(T2)s(T) | 5 | 919.84 | 0.46 | 0.80 | 0.32 |
Parameter estimates | |||||
Parameter | t50, s(T) | t50(T),s(T2) | |||
t50 | 9.47 ± 0.07 | – | |||
t50(A, pH 7.8) | – | 9.54 ± 0.09 | |||
t50 (pH 7.5) | – | 9.36 ± 0.11 | |||
s(A) | −6.18 ± 0.32 | −6.22 ± 0.33 | |||
s(pH 7.8) | −7.12 ± 0.37 | −7.16 ± 0.38 | |||
s(pH 7.5) | −7.6 ± 0.41 | −7.53 ± 0.41 |
Model . | K . | AICc . | ΔAICc . | Likelihood . | AICc weight . |
---|---|---|---|---|---|
t50, s | 2 | 923.39 | 4.01 | 0.13 | 0.05 |
t50(T), s | 4 | 924.39 | 5.01 | 0.08 | 0.03 |
t50(T2), s | 3 | 922.85 | 3.47 | 0.18 | 0.07 |
t50, s(T) | 4 | 919.38 | 0.00 | 1.00 | 0.40 |
t50(T)s(T) | 6 | 921.79 | 2.41 | 0.30 | 0.12 |
t50(T2)s(T) | 5 | 919.84 | 0.46 | 0.80 | 0.32 |
Parameter estimates | |||||
Parameter | t50, s(T) | t50(T),s(T2) | |||
t50 | 9.47 ± 0.07 | – | |||
t50(A, pH 7.8) | – | 9.54 ± 0.09 | |||
t50 (pH 7.5) | – | 9.36 ± 0.11 | |||
s(A) | −6.18 ± 0.32 | −6.22 ± 0.33 | |||
s(pH 7.8) | −7.12 ± 0.37 | −7.16 ± 0.38 | |||
s(pH 7.5) | −7.6 ± 0.41 | −7.53 ± 0.41 |
Model indicates the parameters used and how they were modelled (see text for details). Where factors are included parenthetically, the parameter is modelled as a linear function of those parameters. T indicates that the parameter varies among all treatments and T2 indicates the post hoc models in which the parameter was the same for the Ambient (A) and pH 7.8 treatments which differed from the pH 7.5 treatment. K is the number of parameters for each model. To show the greatest difference among the treatments supported by the data, the more complex t50(T2)s(T) model is presented in Figure 2. The t50 parameters have units of days and the s parameters are unitless.
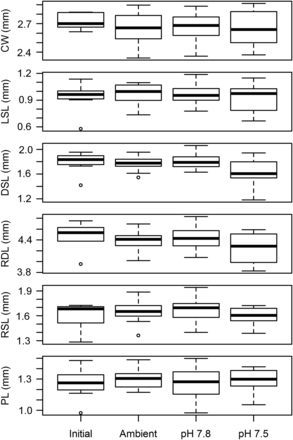
Box-plots of measurements made on larvae in the 2011 experiment. Initial represents larvae measured the day after hatching. Ambient, pH 7.8, and pH 7.5 represent larvae that had been held in ambient and acidified water for 10 d. Measurements included CW—carapace width, LSL—lateral spine length, DSL—dorsal spine length, RDL—rostro-dorsal length, RSL—rostral spine length, and PL—protopodite length.
![Mortality of Tanner crab larvae during starvation in 2011 at three pH treatments over time. Points are the average per cent mortality ± 1 standard deviation at each treatment and lines are one of the best fit logistic regression model for each [the t50(T2)s(T) model is presented, see Table 2 for parameter estimates]. The points for pH 7.8 and pH 7.5 are offset by 0.2 and −0.2 d, respectively, to avoid overlapping error bars.](https://oup.silverchair-cdn.com/oup/backfile/Content_public/Journal/icesjms/73/3/10.1093_icesjms_fsv251/2/m_fsv25102.gif?Expires=1750224482&Signature=NjCCH0VYTyU0vNtT9uh6aOsS3ZP68uHyN7z3PFehmUfySHxCy-k~i1YEnadzhl1pdeMxL1yUyzFuhzxuHmuE7sJ-7nfVx0rNIBjksYcN2VXgpKU0LcEe-CJ3oCZYq8LTArL634NjFtiqyyqbVGVD9uvTWr8-3dAwOr4zwLuvT-hUAbtVLUg5EM-lDOIrvnKDpRaoXJ2uym9x35uHpICxa6QH77NkJwY745XQOyB2t32jEzBIhbX6BbiGelOnNL0APTNIdyuw5YAMf5RA6PEebpdgmVM-4EtqLQS6YbVF4G0Z9KQvW6YGM2hlBMsOECtnjqpy-H75DlNXJDADwD6JBA__&Key-Pair-Id=APKAIE5G5CRDK6RD3PGA)
Mortality of Tanner crab larvae during starvation in 2011 at three pH treatments over time. Points are the average per cent mortality ± 1 standard deviation at each treatment and lines are one of the best fit logistic regression model for each [the t50(T2)s(T) model is presented, see Table 2 for parameter estimates]. The points for pH 7.8 and pH 7.5 are offset by 0.2 and −0.2 d, respectively, to avoid overlapping error bars.
2012 and 2013 laboratory-reared larvae
In 2012, there was a statistically significant effect of embryo treatment on larval morphometrics (Global R = 0.074, p = 0.02). Pairwise comparisons showed that the only difference was between pH 7.8 and pH 7.5, and ordination showed that, on average, pH 7.5 embryos were slightly larger than pH 7.8; however, there was significant overlap and the effect size was small; a 2% difference in the rostro-dorsal length, for example (Figure 3), is unlikely to have any discernible effect on larval survival or performance. Additionally, the low Global R value also indicates that the differences were not biologically relevant (Clarke and Warwick, 2001). In 2013, larval morphometrics differed among embryo pH treatments (Global R = 0.383, p < 0.0001), with pH 7.8 and Ambient larvae being larger than pH 7.5 larvae (Figure 3); the effect sizes were larger than in 2012, with pH 7.5 larvae having 10% smaller carapace width than Ambient and pH 7.8 larvae, for example (Figure 3).
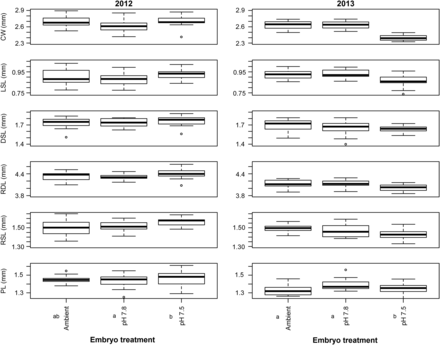
Box-plots of measurements made on larvae in the 2012 and 2013 experiment. Ambient, pH 7.8, and pH 7.5 represent the treatment embryos were reared at. Measurements included CW—carapace width, LSL—lateral spine length, DSL—dorsal spine length, RDL—rostro-dorsal length, RSL—rostral spine length, and PL—protopodite length. Statistical differences within each year are indicated with different letters next to the treatments.
In 2012 and 2013, the t50 and s parameters varied among treatments at both the embryo and larval stages as well as in their interaction in the best fit models of larval survival (Supplementary material S3). In 2012, the full model contained a number of parameters whose 95% confidence intervals, as indicated by the standard error of the parameter estimates, substantially overlapped 0. We thus created a post hoc model in which these parameters were eliminated, thus increasing the parsimony of the best model substantially (Supplementary material S3). In both years, the effect of treatment at the embryo stage was larger than treatment at the larval stage; larvae from embryos that developed in pH 7.5 water died 3.01 d later in 2012 and 3.32 d later in 2013 (when averaged across the larval treatments) than those that developed in Ambient water (Figure 4, Table 3). In 2012, larvae from embryos that had developed in pH 7.8 water were similar to Ambient larvae, whereas in 2013, they were intermediate between the Ambient and pH 7.5 larvae. The effect of treatment at the larval stage and the interactive effects were much smaller and no clear pattern in the data is apparent (Figure 4, Table 3).
Parameter estimates for logistic regressions on larval survival experiments in 2012 and 2013 graphed in Figure 4.
Embryo | A | A | A | 7.8 | 7.8 | 7.8 | 7.5 | 7.5 | 7.5 |
Larvae | A | 7.8 | 7.5 | A | 7.8 | 7.5 | A | 7.8 | 7.5 |
2012 | |||||||||
t50 | 9.52 | 9.09 | 9.80 | 9.24 | 8.81 | 9.52 | 12.80 | 13.14 | 11.50 |
s | −3.13 | −3.13 | −3.13 | −2.86 | −2.86 | −3.18 | −3.93 | −3.93 | −3.54 |
2013 | |||||||||
t50 | 12.42 | 11.36 | 10.44 | 14.54 | 14.05 | 12.06 | 15.58 | 13.28 | 15.31 |
s | −3.81 | −4.01 | −3.44 | −4.86 | −5.31 | −3.39 | −5.10 | −4.25 | −7.25 |
Embryo | A | A | A | 7.8 | 7.8 | 7.8 | 7.5 | 7.5 | 7.5 |
Larvae | A | 7.8 | 7.5 | A | 7.8 | 7.5 | A | 7.8 | 7.5 |
2012 | |||||||||
t50 | 9.52 | 9.09 | 9.80 | 9.24 | 8.81 | 9.52 | 12.80 | 13.14 | 11.50 |
s | −3.13 | −3.13 | −3.13 | −2.86 | −2.86 | −3.18 | −3.93 | −3.93 | −3.54 |
2013 | |||||||||
t50 | 12.42 | 11.36 | 10.44 | 14.54 | 14.05 | 12.06 | 15.58 | 13.28 | 15.31 |
s | −3.81 | −4.01 | −3.44 | −4.86 | −5.31 | −3.39 | −5.10 | −4.25 | −7.25 |
Embryo and Larvae indicates the treatments at the embryo and larval stages and include A—Ambient, 7.8—pH 7.8, and 7.5—pH 7.5. The t50 parameters have units of days and the s parameters are unitless.
Parameter estimates for logistic regressions on larval survival experiments in 2012 and 2013 graphed in Figure 4.
Embryo | A | A | A | 7.8 | 7.8 | 7.8 | 7.5 | 7.5 | 7.5 |
Larvae | A | 7.8 | 7.5 | A | 7.8 | 7.5 | A | 7.8 | 7.5 |
2012 | |||||||||
t50 | 9.52 | 9.09 | 9.80 | 9.24 | 8.81 | 9.52 | 12.80 | 13.14 | 11.50 |
s | −3.13 | −3.13 | −3.13 | −2.86 | −2.86 | −3.18 | −3.93 | −3.93 | −3.54 |
2013 | |||||||||
t50 | 12.42 | 11.36 | 10.44 | 14.54 | 14.05 | 12.06 | 15.58 | 13.28 | 15.31 |
s | −3.81 | −4.01 | −3.44 | −4.86 | −5.31 | −3.39 | −5.10 | −4.25 | −7.25 |
Embryo | A | A | A | 7.8 | 7.8 | 7.8 | 7.5 | 7.5 | 7.5 |
Larvae | A | 7.8 | 7.5 | A | 7.8 | 7.5 | A | 7.8 | 7.5 |
2012 | |||||||||
t50 | 9.52 | 9.09 | 9.80 | 9.24 | 8.81 | 9.52 | 12.80 | 13.14 | 11.50 |
s | −3.13 | −3.13 | −3.13 | −2.86 | −2.86 | −3.18 | −3.93 | −3.93 | −3.54 |
2013 | |||||||||
t50 | 12.42 | 11.36 | 10.44 | 14.54 | 14.05 | 12.06 | 15.58 | 13.28 | 15.31 |
s | −3.81 | −4.01 | −3.44 | −4.86 | −5.31 | −3.39 | −5.10 | −4.25 | −7.25 |
Embryo and Larvae indicates the treatments at the embryo and larval stages and include A—Ambient, 7.8—pH 7.8, and 7.5—pH 7.5. The t50 parameters have units of days and the s parameters are unitless.

Mortality of Tanner crab larvae during starvation at three pH treatments first as embryos and then as larvae in (a) 2012 and (b) 2013. Points are the average per cent mortality and lines are the best fit logistic regression model for each (see Table 3 for parameter estimates). The treatments are A—Ambient, 7.8—pH 7.8, and 7.5—pH 7.5. For example, A–7.8 denotes the mortality of larval crab in pH 7.8 water who spent their embryonic stages in Ambient pH water.
The C and N content of larvae varied with the larval and embryo treatments in both years (Figure 5, Supplementary material S4). In general, exposure to acidified water at the embryo and larval stages increased the C and N content of the larvae, with the effect of the embryo treatment being larger (Figure 5). Larvae exposed to pH 7.8 as embryos did not differ from the Ambient larvae in 2012, but did in 2013. In addition, there was always a drop in the C and N content between the initial measurement made right after hatching and after 7 d of larval starvation. Although there was a significant effect of treatment on the C:N ratio in 2013 (though not in 2012), the effect was small and no pattern is discernible (Figure 5, Supplementary material S4).
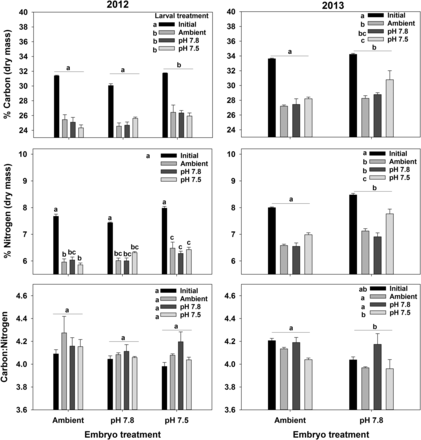
Effects of exposure to acidified water at the embryo and larval stages on carbon (C) and nitrogen (N) content and the C:N ratio in Tanner crab larvae. Bars are the mean + 1 standard error. For the larval treatments, Initial represents C, N, or C:N of the larvae immediately after hatching and Ambient, pH 7.8, and pH 7.5 represent the treatment larvae we held in for 7 d. Statistically significant differences (Tukey's test) among embryo treatments are indicated with letters over groups of bars; differences among Larval treatments are indicated with letters next to the legend. Where there was an interactive effect, difference is indicated with letters over each bar. Results for the 2012 experiments are in the left-side plots and those for the 2013 experiments are on the right-side plots.
Larval Ca and Mg content varied with larval and embryo treatments in both years (Figure 6, Supplementary material S4). Exposure to acidified water at the embryo stage reduced the Ca content in both years and the Mg content in 2012 (the lack of a difference in 2013 is probably because we were unable to run this experiment with pH 7.5 larvae). Ca content was highest in larval reared at pH 7.8 and was lowest in those reared at pH 7.5, with those reared at the Ambient in between. Larvae also generally increased their Ca and Mg contents during their first 7 d as larvae (Figure 6). In 2012, the Mg content of larvae exposed to pH 7.5 water at the embryo stage was lower than the other two treatments. The Ca:Mg ratio also decreased in acidified water at the embryo stage, and was highest in larvae reared at pH 7.8 during the larval phase (Figure 6). To meet the assumption of homogeneity of variance, the Ca:Mg ratio in 2012 was square-root-transformed, and the 2013 Mg content was log-transformed before analysis.
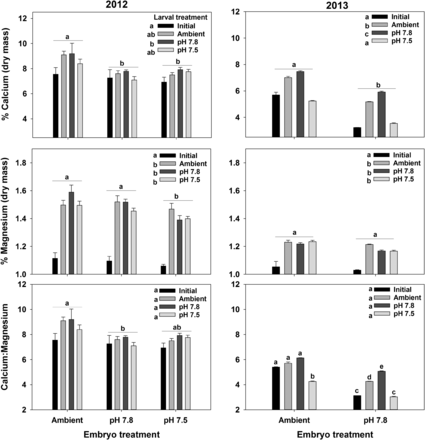
Effects of exposure to acidified water at the embryo and larval stages on calcium and magnesium content and the Ca:Mg ratio in Tanner crab larvae. Bars are the mean + 1 standard error. For the larval treatments, Initial represents the larvae immediately after hatching and Ambient, pH 7.8, and pH 7.5 represent the treatment larvae we held in for 7 d. Statistically significant differences (Tukey's test) among embryo treatments are indicated with letters over groups of bars; differences among Larval treatments are indicated with letters next to the legend. Where there was an interactive effect, difference is indicated with letters over each bar. Results for the 2012 experiments are in the left-side plots and those for the 2013 experiments are on the right-side plots.
In 2012, the average larval mass varied among the larval treatments, with those reared in pH 7.8 being highest, pH 7.5 being intermediate, and Ambient being lowest (Figure 7, Supplementary material S4). In 2013, exposure to ambient water at both the embryo and larval stages was associated with higher larval mass (Figure 7).
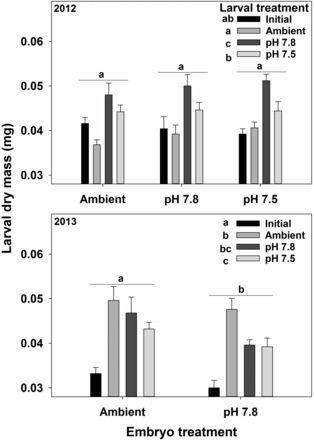
Effects of exposure to acidified water at the embryo and larval stages on the mass of Tanner crab larvae in 2012 and 2013 experiments. Bars are the mean + 1 standard error. For the larval treatments, Initial represents the larvae immediately after hatching and Ambient, pH 7.8, and pH 7.5 represent the treatment larvae we held in for 7 d. Statistically significant differences (Tukey's test) among embryo treatments are indicated with letters over groups of bars; differences among Larval treatments are indicated with letters next to the legend.
All data and metadata associated with this project have been archived at the NPRB website (http://project.nprb.org/view.jsp?id=ab9b22fc-3409-4bf9-8d5e-454e28371d7b). Metadata is currently available and data will be publically available after a 2-year embargo period or upon request.
Discussion
Exposure to acidified water had a significant effect on the Tanner crab larvae, and there were substantial carryover effects from exposure of the females during oogenesis and embryogenesis. Starvation-survival time and morphology of wild-brooded larvae were not affected by low pH water, indicating that they were generally tolerant of the range of pHs in this experiment. However, exposure to low pH water during embryogenesis increased survival time (indicative of decreased metabolism), decreased the calcium content and mass, and changed the morphology of the larvae. Effects were mostly apparent at pH 7.5, indicating that Tanner crab larvae are moderately sensitive to high pCO2. However, given the presence of carryover effects and the effects high pCO2 have on other life history stages (Long et al., 2013b; Swiney et al., 2016), high pCO2 may have a substantial effect on Tanner crab populations, and fisheries, within the next 80 years (Punt et al., 2016).
Exposure to acidified water at the larval phase had little effect on larvae. We saw no biologically significant differences among larval treatments for the wild-brooded larvae, and only slight (if any) differences in carbon and nitrogen content, calcium or magnesium content, or starvation survival in the lab-brooded larvae. Effect sizes were either small or zero in 2012 and were greater in 2013. The biggest difference was lower calcium content followed by higher per cent carbon and nitrogen contents of pH 7.5 larvae. The higher C and N content may be because in 2013, the pH 7.5 larvae also had a smaller average mass than the other two treatments, indicating that total carbon and nitrogen content per larvae were similar among treatments. Similarly, the mass of embryo porcelain crab, Petrolisthes cinctipes, reared under low pH was lower; the per cent carbon was higher leading to almost identical carbon content per embryo (Carter et al., 2013). Of the Tanner crab life history stages tested, including the embryos (Swiney et al., 2016) and juveniles (Long et al., 2013b) both of which exhibited increases in the mortality rate, the larval phase seems the least affected by direct exposure to high pCO2. Stage-specific sensitivities to ocean acidification are common in crustacean. In Pe. cinctipes, acidified water does not affect larval survival, but does decrease juvenile survival (Ceballos-Osuna et al., 2013). The barnacle, Semibalanus balanoides, does not show increased mortality at the embryo (Findlay et al., 2009) or the post-larval (Findlay et al., 2010b) stages under acidified conditions, but does at the larval (Findlay et al., 2010a) and adult stages (Findlay et al., 2009). Even different larval phases can differ in sensitivity; H. araneus and Homarus gammarus larvae are only sensitive at the megalopa stage (Walther et al., 2010; Small et al., 2015). Therefore, insensitivity to acidification at one stage does not imply a general insensitivity throughout an organism's ontogeny. Later larval stages of larval development in Tanner crabs may be more sensitive than the first stage tested here.
Tanner crab larvae may be better adapted to changes in pH than other life history stages because of their natural environment; many species of crustaceans have larvae that are tolerant of low pH (Arnold et al., 2009; Arnberg et al., 2013; Schiffer et al., 2013). The larval phase occurs in the water column during the spring bloom when diel fluctuations in the water pH often high; short-term pH variability is higher during periods of high biological activity (Hofmann et al., 2011, 2015), whereas deeper benthic habitat has less short-term variability (Matson et al., 2014). Many crustacean larvae, including Tanner crab larvae (Wolotira et al., 1990), exhibit diel vertical migration, and there is a large difference in the carbonate chemistry between surface waters and those below the mixed layer in the Bering Sea when Tanner larvae are present (Cross et al., 2013). Larval Tanner crabs may therefore be exposed to much higher short-term variability in pH than later benthic phases, and may therefore be better adapted to such pH variability than other stages. Similarly, Styf et al. (2013) argued that the insensitivity of Nephrops norvegicus embryos to low pH water was an adaptation to the naturally low pH environment in burrows in which the embryos are incubated in the wild.
Carryover effects (sometimes called transgenerational effects) from exposure to low pH during the embryo phase had larger effect sizes on Tanner crab larvae than effects from direct exposure, and carryover effects from maternal exposure during the oogenesis phase increased the effect sizes. Larval morphometry did not differ among larval treatments for wild-brooded larvae; differed statistically, but not biologically, among embryo treatment in 2012; and differed both statistically and biologically among embryo treatment in 2013. The direct effects of acidification on Tanner crab embryos were similar (Swiney et al., 2016); differences among pH treatments on embryo development were not biologically significant until 2013. Similarly, the reduction in calcium and magnesium content and the increase in starvation survival time between the Ambient and pH 7.8 embryo treatments were greater in 2013 than in 2012. Because of high mortality at the embryo and pre-zoea stages for the pH 7.5 embryo treatment (Swiney et al., 2016), we cannot explicitly compare these parameters between the years for the pH 7.5 embryo treatment; however, the greater mortality rate of the first larval stage is a clear indication of substantial carryover effects, despite the lack of data on non-lethal effects. Although the larval exposure experiments were of a short duration and a longer exposure time might change the relative importance of direct vs. carryover effect, this does not lessen the import of carryover effects.
These results highlight the cumulative importance of carryover effects throughout the entire life history in determining the overall effects of high pCO2. In Tanner crabs, larvae experienced negative effects due to exposure at earlier stages. Similarly, Olympic oysters (O. lurida) juveniles experience decreased growth as juveniles if exposed to low pH water as larvae (Hettinger et al., 2012) and H. araneus larvae had reduced survival following exposure at the embryo stage (Schiffer et al., 2014). In these cases, energetic costs incurred at earlier stages may have reduced later performance. In contrast, in other species, carryover effects ameliorate the effects of ocean acidification. Sydney rock oyster, Saccostrea glomerata, larvae have lower survival and growth in acidified water unless their parents are exposed before spawning (Parker et al., 2012); these positive effects extend into adulthood (Parker et al., 2015). Effects may also be mixed and depend on life history stage; maternal exposure to high pCO2 eliminates negative effects of larval exposure in Strongylocentrotus droedachiensis, but larval exposure reduces juvenile survival (Dupont et al., 2013). Ameliorative effects may be a product of physiological plasticity (Dupont et al., 2013; Thor and Dupont, 2015) or increased maternal investment in each offspring (Parker et al., 2012). There is no evidence of positive carryover effects on Tanner crabs in this study though. Many studies fail to account for carryover effects and thus likely underestimate (or overestimate) the cumulative effects of ocean acidification (Hettinger et al., 2013).
Reduced calcium and magnesium content and Ca:Mg ratio at pH 7.5 could affect larval behaviour and survival and is consistent with effects on mature females (Swiney et al., 2016), and juveniles (Long et al., 2013b), and with effects on larvae of other species, such as the European lobster, Ho. gammarus (Arnold et al., 2009; Small et al., 2015) and spider crab, H. araneus (Walther et al., 2011). Where effect size differs among larval stages, the megalopa stage, which is typically more calcified than earlier stages (Anger, 2001), is the most likely to show effects (Arnold et al., 2009; Walther et al., 2011; Small et al., 2015). Tanner crab larvae from embryos reared at pH 7.8 and 7.5 hatched with lower calcium contents than those reared at Ambient pH and larvae held at pH 7.5 did not accumulate any additional calcium over the first 7 d, whereas larvae at pH 7.8 and Ambient pH did accumulate calcium. Lower calcium content in larvae likely decreases the hardness of their cuticle. This could lead to increased vulnerability to predators (Amaral et al., 2012); a small change in prey vulnerability (Long et al., 2008) can substantially increase predation (Long and Seitz, 2008) and decrease population size (Long et al., 2014). Reduced hardness in the cuticle could lead also to a decreased capacity to masticate food, which could reduce larval survival. Although a lower Ca:Mg ratio is associated with stronger calcite (Magdans and Gies, 2004), any increase in hardness from this is unlikely to compensate for the reduction in the overall Ca and Mg content. Also, although later larval stages might be able to “catch-up” to ambient larvae in terms of calcium content (Walther et al., 2011), there would still be a period of increase vulnerability from low calcium levels. The effects at pH 7.8 are interesting. Exposure at the larval stage increased calcium content (trend in 2012, statistically in 2013), while exposure at the embryo stage decreased calcium content. At pH 7.8, red king crab larvae also exhibited increased calcium content (Long et al., 2013a). Although such dome-shaped response, with highest calcification levels at intermediate pHs, occurs in other taxa (Ries et al., 2009), this is the first time, to our knowledge, that it has been observed in a crustacean.
The carbon and nitrogen contents and larval size indicate that ocean acidification has a complex effect on larval development and energetics. At hatching, larvae exposed to low pH as embryos had a smaller size but a higher per cent carbon and nitrogen content. Similarly, just before hatching, embryos held in low pH were smaller, but had larger yolks (Swiney et al., 2016). Embryos might have developed more slowly at low pH, similar to barnacle embryos (Findlay et al., 2009), and this might affect the hatching size and elemental composition of the larvae. A larger hatch size could have a positive effect; larger Chasmagnathus granulate larvae had a shorter developmental time than smaller ones (Giménez et al., 2004). One aspect of carbon and nitrogen metabolism that we did not observe was an increase in protein metabolism under reduced pH. Larval Ho. gammarus (Small et al., 2015), H. araneus (Walther et al., 2010), and Pe. cinctipes (Carter et al., 2013) all increased protein metabolism as evidenced by increased C:N ratios under acidified conditions. However, this is not a universal response; larval red king crab, P. camtschaticus, do not increase protein metabolism at reduced pH (Long et al., 2013a).
The starvation survival time increased with decreasing pH. Larval starvation survival times are primarily a function of the energetic reserves at hatching and the metabolic rate after hatching (Hunter, 1981). At hatching, the total (not per cent) carbon and nitrogen content of the larvae were similar among embryo treatments, indicating similar lipid and protein reserves (Anger and Harms, 1990). After 7 d of starvation, Ambient larvae had a larger decrease in both carbon and nitrogen content than larvae held in low pH water in 2013. Under starvation conditions, crustacean larvae typically use lipid reserves (carbon) for energy first and then protein (nitrogen) (Anger, 2001). This suggests that larvae held under acidified conditions have a lower metabolic rate than those under Ambient conditions, and that after 7 d, Ambient larvae had depleted their lipid reserves and were metabolizing proteins. Lower metabolism in larvae held in acidified conditions would lead to an increase in their survival time. Metabolic depression is a common response to ocean acidification among many taxa, including crustacean, likely because it reduces hemolymph and intracellular acidity by reducing CO2 production from respiration (Small et al., 2010; Carter et al., 2013; Schiffer et al., 2014). Although it does, as in this case, increase survival time under starvation conditions, it can have negative consequences for growth (Carter et al., 2013) and therefore (in mature animals) reproduction (Swiney et al., 2012). This increase in starvation survival time and decrease in metabolism contrasts with the response of other crustaceans. Larval Ho. gammarus (Small et al., 2015) and (during molting) H. araneus (Schiffer et al., 2013) both increase metabolism in acidified water. Larval P. camtschaticus under acidified conditions had a decreased starvation survival time and a faster depletion of carbon reserves, indicating a higher metabolism (Long et al., 2013a).
These results, when combined with those on other life history stages, suggest that the effects of ocean acidification on the Tanner crab population and fishery are likely to be felt within 80 years. Although the direct effects on larvae were relatively slight, effects on embryos, juveniles, and adults are larger (Long et al., 2013b; Swiney et al., 2016). Further, strong carryover effects on the larval stage from earlier stages suggest carryover effects occur at later stages (juvenile and adult) and may be magnified by increased exposure time. Mortality at the juvenile, and to a lesser extent, the embryo stages was significantly higher at pH 7.8, and even higher at pH 7.5, compared with Ambient pH. This is predicted to cause a decrease in the Tanner crab population and fishery before the end of the century (Punt et al., 2016).
A number of questions on the effects of ocean acidification on Tanner crabs remain unanswered. The gradual change in pH over decades may allow evolutionary adaptation. Even at the lowest pH tested, pH 7.5, some larvae, embryos, and juveniles survived and grew, indicating variability in the individual tolerances for low pH (Long et al., 2013a, b; Meseck et al., 2015; Swiney et al., 2016) and the potential for natural selection to allow adaptation to acidified conditions (Reusch, 2014). Indirect effects, such as changes in predation on Tanner crabs or in their ability to feed, may alter the net effect of ocean acidification. Other stressors, such as increasing temperatures, may interact with ocean acidification (Breitburg et al., 2015). Further research is necessary to inform policy-makers of the likely effects that ocean acidification will have on this commercial species and the best management steps to protect this resource.
Acknowledgements
This project was funded by the North Pacific Research Board (NPRB publication no. 537) and the National Marine Fisheries Service (NMFS). We thank NMFS biologists and laboratory technicians for their assistance which made this project possible. Previous versions of this paper were improved by comments from J. Napp, C. Ryer, J. Long, and four anonymous reviewers. The findings and conclusions in the paper are those of the authors and do not necessarily represent the views of the National Marine Fisheries Service, NOAA. Reference to trade names does not imply endorsement by the National Marine Fisheries Service, NOAA.
References
Author notes
Handling editor: C. Brock Woodson