-
PDF
- Split View
-
Views
-
Cite
Cite
Yi Zhang, Ling-Ling Ruan, Ming-Rui Li, Lu Yao, Fang-Fang Li, You-Long Xie, Jing Tang, Qian Feng, Xiao-Yan Chen, Yu-Bin Ding, Li-Juan Fu, Palmitic acid impairs human and mouse placental function by inhibiting trophoblast autophagy through induction of acyl-coenzyme A-binding protein (ACBP) upregulation, Human Reproduction, Volume 39, Issue 7, July 2024, Pages 1423–1431, https://doi.org/10.1093/humrep/deae091
- Share Icon Share
Abstract
Can exposure to palmitic acid (PA), a common saturated fatty acid, modulate autophagy in both human and mouse trophoblast cells through the regulation of acyl-coenzyme A-binding protein (ACBP)?
PA exposure before and during pregnancy impairs placental development through mechanisms involving placental autophagy and ACBP expression.
High-fat diets, including PA, have been implicated in adverse effects on human placental and fetal development. Despite this recognition, the precise molecular mechanisms underlying these effects are not fully understood.
Extravillous trophoblast (EVT) cell line HTR-8/SVneo and human trophoblast stem cell (hTSC)-derived EVT (hTSCs-EVT) were exposed to PA or vehicle control for 24 h. Female wild-type C57BL/6 mice were divided into PA and control groups (n = 10 per group) and subjected to a 12-week dietary intervention. Afterward, they were mated with male wild-type C57BL/6 mice and euthanized on Day 14 of gestation. Female ACBPflox/flox mice were also randomly assigned to control and PA-exposed groups (each with 10 mice), undergoing the same dietary intervention and mating with ACBPflox/floxELF5-Cre male mice, followed by euthanasia on Day 14 of gestation. The study assessed the effects of PA on mouse embryonic development and placental autophagy. Additionally, the role of ACBP in the pathogenesis of PA-induced placental toxicity was investigated.
The findings were validated using real-time PCR, Western blot, immunofluorescence, transmission electron microscopy, and shRNA knockdown approaches.
Exposure to PA-upregulated ACBP expression in both human HTR-8/SVneo cells and hTSCs-EVT, as well as in mouse placenta. PA exposure also induced autophagic dysfunction in HTR-8/SVneo cells, hTSCs-EVT, and mouse placenta. Through studies on ACBP placental conditional knockout mice and ACBP knockdown human trophoblast cells, it was revealed that reduced ACBP expression led to trophoblast malfunction and affected the expression of autophagy-related proteins LC3B-II and P62, thereby impacting embryonic development. Conversely, ACBP knockdown partially mitigated PA-induced impairment of placental trophoblast autophagy, observed both in vitro in human trophoblast cells and in vivo in mice.
N/A.
Primary EVT cells from early pregnancy are fragile, limiting research use. Maintaining their viability is tough, affecting data reliability. The study lacks depth to explore PA diet cessation effects after 12 weeks. Without follow-up, understanding postdiet impacts on pregnancy stages is incomplete. Placental abnormalities linked to elevated PA diet in embryos lack confirmation due to absence of control groups. Clarifying if issues stem solely from PA exposure is difficult without proper controls.
Consuming a high-fat diet before and during pregnancy may result in complications or challenges in successfully carrying the pregnancy to term. It suggests that such dietary habits can have detrimental effects on the health of both the mother and the developing fetus.
This work was supported in part by the National Natural Science Foundation of China (82171664, 82301909) and the Natural Science Foundation of Chongqing Municipality of China (CSTB2022NS·CQ-LZX0062, cstc2019jcyj-msxmX0749, and cstc2021jcyj-msxmX0236). The authors declare that they have no conflict of interest.
N/A.
Introduction
Palmitic acid (PA), a 16-carbon long-chain saturated fatty acid (C16H32O2), is abundantly present in the human diet and comprises approximately 65% of the body’s saturated fatty acids and contributes 28–32% of the total fatty acids in serum (Fatima et al., 2019). Elevated intake of saturated fatty acids, particularly PA, leads to an increased circulation of these fatty acids in the bloodstream (Blüher, 2019). Substantial research has meticulously elucidated the role of PA as a signaling molecule, exerting influence on the progression of diverse pathological conditions, encompassing metabolic syndrome, cardiovascular diseases, cancer, neurodegenerative diseases, and inflammation at the molecular level (Zong et al., 2016).
PA stands out as a significant contributor to the increased risk of obesity, especially notable in pregnant women with a high body mass index (Desoye and Herrera, 2021; Zhao et al., 2023). Maternal obesity has been increasingly associated with a spectrum of adverse pregnancy outcomes, including gestational diabetes, gestational hypertension, preeclampsia, macrosomia, and stillbirth (Catalano and Shankar, 2017; Creanga et al., 2022). Recent studies have highlighted how obesity can disrupt normal placental development through various mechanisms, including altered angiogenesis, inflammation, hormonal imbalances, and epigenetic modifications (Kim et al., 2014; Hjort et al., 2022; Napso et al., 2022). These disruptions in placental development can have profound consequences on fetal health and contribute to pregnancy complications (Howell and Powell, 2017). Moreover, emerging research has shown that poor dietary habits can induce maternal obesity, leading to structural and functional changes in the placenta (Stuart et al., 2018). Maternal obesity often results in the ectopic deposition of lipids within placental tissue, triggering cytotoxicity and chronic inflammatory responses (Pantaleao et al., 2022). It has been shown that a significantly increased PA concentration is present in serum samples of pregnant women bearing children with congenital heart disease (CHD). Feeding pregnant mice with PA increased CHD risk in offspring (Zhao et al., 2023). Despite this growing body of knowledge, the precise mechanisms by which obesity affects placental development and pregnancy outcomes remain unclear.
Studies have shown that elevated levels of acyl-CoA-binding protein (ACBP), a molecule with a well-conserved structure and function, have been observed in obese individuals compared to those with a leaner physique (Charmpilas et al., 2020). ACBP is known to stimulate appetite and lipid metabolism (Sica et al., 2021). Moreover, studies have reported increased ACBP mRNA expression in white adipose tissue and higher plasma ACBP levels in individuals with obesity (Li et al., 2021). Research findings suggest that genetic manipulation or antibody-mediated neutralization of ACBP can lead to reduced appetite and induce lipocatabolic reactions in mice, further emphasizing its role in metabolism regulation (Bravo-San Pedro et al., 2019b).
Furthermore, ACBP is secreted via an autophagy-dependent mechanism, indicating a potential mechanism for controlling lipid metabolism through intracellular regulation of this protein’s levels (Bravo-San Pedro et al., 2019a; Montégut et al., 2023). In mice, the systemic administration of ACBP protein inhibits autophagy, promotes lipogenesis, reduces glycemia, and stimulates appetite and weight gain (Joseph et al., 2021). Autophagy, on the other hand, is a cellular process essential for energy catabolism, removing unnecessary or damaged organelles and maintaining cellular homeostasis (Glick et al., 2010). Dysregulated autophagy, either excessively high or low, can lead to cell death. Proper autophagy regulation is crucial for placental stability (Redman et al., 2022), and disturbances in autophagy have been linked to pregnancy complications, such as preterm birth, preeclampsia, and restricted intrauterine growth (Bildirici et al., 2012).
In this study, we utilized human trophoblasts alongside a placenta-specific ACBP conditional knockout mouse model exposed to PA. Our aim was to explore the involvement of ACBP in the development of PA-induced placental toxicity.
Materials and methods
Ethical approval
This study received ethical approval from the Ethics Committee (reference: 2022122) of Chongqing Medical University, and written informed consent was obtained from all patients before sample collection. All animal experiments conducted in this study were approved by the Animal Care and Use Committee of Chongqing Medical University.
Culture of human extravillous trophoblast cell line HTR-8/SVneo
HTR-8/SVneo cell line, graciously provided by Professor Charles H. Graham from Queen’s University in Canada, comprises immortalized human placental extravillous trophoblast (EVT) cells derived from first-trimester human trophoblasts (Graham et al., 1993; Abbas et al., 2020). These cells express various markers characteristic of trophoblasts and are extensively utilized for functional studies of trophoblast and placenta. For details of experimental procedures, see Supplementary materials and methods.
Culture of human trophoblast stem cells and induction of human trophoblast stem cell-EVT
Human trophoblast stem cells (hTSCs) were cultured in DMEM/F12 medium following the protocol by Okae et al. (2018). hTSC-EVT cells were obtained using the same protocol. Trophoblast lineages were identified using relevant markers (Supplementary Fig. S1A–F). Experimental procedures are detailed in Supplementary materials and methods.
Drug treatments for human EVT cell line and hTSC-EVT
PA, obtained from Sigma-Aldrich, was prepared by dissolving it in 0.1 M NaOH at 70°C to create a 100-mM stock solution. Subsequently, it was mixed with 15% fatty-acid-free BSA (Sigma-Aldrich) at 37°C for 2 h, resulting in a 5-mM PA–BSA conjugate solution. In brief, 0.15 g of BSA was dissolved in 1 ml of deionized water with stirring at room temperature. The final stock solution was filtered through a 0.45µm filter, aliquoted, and stored at −20°C. HTR-8/SVneo cells were treated with various concentrations of PA (0, 0.25, 0.5 mM) for 24 h, with BSA solution used as a control. While hTSC-EVT was treated with 0.5 mM PA for 24 h. To investigate the impact of PA on autophagy in HTR-8/SVneo cells, a combination of 5 μM Rapamycin (Rap) (Sigma-Aldrich) or 10 μM chloroquine (CQ) (Sigma-Aldrich) was added to the HTR-8/SVneo cells with or without PA for an additional 24 h.
Animals and treatments
The experimental procedures were approved by the Animal Protection and Use Committee of Chongqing Medical University and followed the principles outlined in the Guide for the Protection and Use of Laboratory Animals. Wild-type C57BL/6 female and male mice were procured from Beijing Vital River Laboratory Animal Technology Co., Ltd (Beijing, China). Female control mice (n = 10) were provided with a standard diet, whereas those in the PA diet-induced group (n = 10) were fed normal mouse chow supplemented with 5% PA (Jiangsu Xietong Pharmaceutical Bio-Engineering). Following 12 weeks of dietary intervention, both groups of mice were mated with wild-type C57BL/6 male mice. Pregnant mice from the two groups were kept with their respective diet until sacrifice on Day 14 of gestation. Day 1 of pregnancy (D1) was defined as the day when a vaginal plug was observed.
To generate placenta-specific ACBP conditional knockout mice, we utilized the Cre-LoxP system in C57BL/6 mice. ACBPflox/flox mice were generously provided by Prof. Susanne Mandrup from the University of Southern Denmark (Ditte et al., 2013), while ELF5-Cre mice were obtained from Prof. Haibin Wang at Xiamen University (Kong et al., 2018). The mice were housed in the Animal Breeding Center of Chongqing Medical University under standard conditions, which included a constant temperature (22 ± 2°C), consistent humidity (50%), a 12-h light/dark cycle, access to regular chow, and ad libitum water intake (five female mice per cage). ACBPflox/flox female mice were randomly divided into control and PA-exposed groups, each consisting of 10 mice. After 12 weeks of dietary intervention, both groups of mice were mated with ACBPflox/flox/ELF5-Cre males and sampled on Day 14 of gestation. Fetuses with the ACBPflox/flox genotype were assigned to the control group, whereas those with the ACBPflox/flox/ELF5-Cre (CKO) genotype constituted the experimental group (Supplementary Fig. S1H–J). This division aimed to investigate the effects of ACBP knockout on pregnancy outcomes induced by PA. The placenta and embryos were collected on gestational Day 14, and their images were captured and weights calculated. The embryo resorption rate was calculated as follows: Resorption rate (%) = [number of resorbed embryos/number of total (resorbed + viable) embryos] × 100 (Yin et al., 2019). These tissue sections were either fixed in a 4.0% paraformaldehyde (PFA) solution or frozen in liquid nitrogen until further use.
Lentivirus-mediated knockdown of ACBP expression
To knock down ACBP expression, shRNAs were designed targeting two interference targets (Human-ACBP-1 and Human-ACBP-3). The RNA interference target sequences included a scrambled sequence as a negative control shCTR (5′-GACTCCAGTGGTAATCTAC-3′), shACBP-1 (5′-ACCAGTTAAACCAGCTACTCA-3′), and shACBP-3 (5′-GCTCACCATACGGCTCTAACA-3′). The designed DNA was sent to BGI (Shenzhen, China) for sequencing, and the shRNAs were cloned into linearized vectors at AgeI/EcoRI sites with sequences encoding green fluorescent protein (GFP) to construct recombinant lentivirus vectors. HEK293T cells expressing the SV40T antigen, provided by Dr Jun Zhang from Chongqing Medical University, were used for packaging lentiviruse (Pear et al., 1993). Culturing was performed in DMEM-high glucose medium (Sigma-Aldrich, MO, USA) supplemented with 10% FBS. When HEK293T cells reached about 80% confluence, they were passaged at a ratio of 1:3 and inoculated in a 60-mm dish to prepare for viral packaging. The plasmid was added to opti-mem (Beyotime) in proportion, and lipo8000TM (Beyotime) was added into the HEK293T petri dish. The mixture was gently shaken and mixed well to initiate the viral packaging process.
Autophagic flux assay using tandem fluorescent-tagged LC3
The autophagic flux of HTR-8/SVneo cells upon PA treatment was monitored using the RFP/GFP-LC3 and pEGFP-LC3 plasmids (kindly provided by Dr Jun Zhang from Chongqing Medical University) (Zhang et al., 2018). We constructed the specific HTR-8/SVneo cells, which stably expressing the mRFP-GFP-LC3 (tandem fluorescent-tagged LC3, tfLC3) fusion protein. HTR-8/SVneo-tfLC3 cells were isolated by inoculation of mRFP-GFP-LC3 lentivirus followed by selection with puromycin. For transfection with pEGFP-LC3 plasmid, cells were seeded in 12-well plates overnight and then transfected with plasmid using Lipofectamine 2000 (Invitrogen, CA, USA) for 24 h according to the manufacturer’s instructions. Autophagosome maturation was estimated by yellow (accumulation of autophagosomes) and red (autolysosomes) fluorescence structures in cells.
Transmission electron microscopy
Fresh placental tissues were immediately cut into tissue blocks of 1 mm3 and stored in glutaraldehyde (Sigma-Aldrich) fixation solution at 4°C within 1 min. The HTR-8/SVneo cells were treated with PA (0, 0.25, and 0.5 mM) for 24 h and washed 3 times by phosphate-buffered saline (Boster). Then, PA-treated cells were collected into a 2-ml microcentrifuge tube following 0.5% pancreatin (Beyotime) digestion and fixed in glutaraldehyde (Sigma-Aldrich). transmission electron microscopy (TEM) was performed by the College of Life Sciences of Chongqing Medical University.
Western blot
Tissue samples were melted on ice and weighed (about 30–50 mg) into a homogenizer and ground until no tissue was visible to the naked eye. Cell and tissue samples were lysed in RIPA lysis buffer (Beyotime) for 30 min on ice and protein concentrations were assessed with a BCA Protein Assay kit (Beyotime). An equal amount of protein was electrophoresed on SDS-PAGE and then transferred to a polyvinylidene fluoride membrane (PVDF) (Merck Millipore, USA). The PVDF membrane was treated with PBST (0.1% Tween 20 in PBS) containing 5% skimmed milk (Boster Biological Technology, Wuhan, China) for 90 min at 37°C. Primary antibodies were incubated at 4°C overnight. The PVDF membrane was incubated with secondary antibodies for 90 min at 37°C after being washed with PBST. Using ECL (NCM Biotech, Suzhou, China) to detect the protein signals. The following primary antibodies and secondary antibodies were used in this study: SQSTM1/p62 (PTM BIOLAB, 1:1000, Hangzhou, China), Beta-actin (PTM BIOLAB, 1:2000), LAMP1 (PTM BIOLAB, 1:1000), LAMP2 (PTM BIOLAB, 1:1000), LC3B (Cell Signaling Technology, 1:1000, Boston, USA), ACBP (Proteintech, 1:500, Wuhan, China), HRP antirabbit IgG (H + L) (Invitrogen, 1:10 000, Carlsbad, CA, USA), and HRP antimouse IgG (H + L) (Invitrogen, 1:10 000).
RT–qPCR
Total RNA extraction and reverse transcription were performed using TRIzol reagent and the cDNA synthesis kit (TaKaRa, Dalian, China) according to the manufacturer’s protocol. Measuring the optical density at 260 and 280 nm to assess the purity of RNA. Reverse transcription reaction using 1 μg RNA was carried out at 37°C for 15 min, followed by 5 s at 85°C. RT–qPCR was done using the SYBR Premix Ex Taq™ kit (TaKaRa) with the following conditions: 95°C for 2 min, followed by 40 cycles of 95°C for 30 s and 60°C for 30 s. The relative expression of mRNA was calculated by using the 2−ΔΔCt method. Actb and GAPDH played as the internal control. The following primers were purchased from BGI: GAPDH forward: GGAGCGAGATCCCTCCAAAAT and reverse: GGCTGTTGTCATACTTCTCATGG, Actb forward: CTACCTCAGAAGATCCTGACC and reverse: CACAGCTTCTCTTTGATGTCAC, ACBP forward: GAATTTGACAAAGCCGCTGAG and reverse: CCCACAGTAGCTTGTTTGAAGTG.
Immunofluorescent staining
The HTR-8/SVneo cells were seeded onto coverslips and cultured in 24-well culture plates. Cells were permeabilized at room temperature with 0.3% Triton X-100 for 10 min after being fixed with 4% PFA for 20 min at room temperature. Subsequently, HTR-8/SVneo cells were treated with 10% goat serum (Boster) and 3% bovine serum albumin (BSA, BioFroxx, Germany) at 37°C for 1 h after being washed with PBS and incubated primary antibodies at 4°C overnight. The cells were incubated with secondary antibodies for 90 min at 37°C after being washed with PBST. Then 0.1% DAPI was used to stain their nuclei. Fluorescence images were examined using the confocal microscope (Nikon). The following primary antibodies and secondary antibodies were used in this study: LC3B (Cell Signaling Technology, 1:1000), Goat anti-Rabbit IgG, Alexa Fluor Plus 488 (Invitrogen, 1:1000), Goat anti-mouse IgG, Alexa Fluor Plus 488 (Invitrogen, 1:1000), and DAPI (Invitrogen, 1:10 000).
Statistical analysis
All of the data are expressed as mean±SD. Student’s t-test was applied to analyze the data in the corresponding experiments. One-way ANOVA was used to compare group differences. Using GraphPad Prism 9.0 for the statistical analyses. The significant difference was defined as P < 0.05.
Results
PA exposure resulted in elevated ACBP expression in both human trophoblasts and mouse placentas
Given ACBP’s known role in stimulating lipid metabolism (Knudsen et al., 1993; Joseph et al., 2020) along with the observed increase in ACBP mRNA expression in white adipose tissue and elevated plasma ACBP levels in obese individuals (Li et al., 2021), we conducted an investigation into ACBP mRNA and protein levels in human EVTs and the placentas of pregnant mice exposed to PA. Our aim was to determine whether ACBP is implicated in placental development under conditions of PA exposure. Our findings demonstrated that PA significantly increased ACBP protein and mRNA expression in a time- and dose-dependent manner within HTR-8/SVneo cells (Fig. 1A–F). In 0.5mM PA-treated hTSCs-EVT, both protein and mRNA expressions of ACBP displayed a substantial increase (Fig. 1G–I). Moreover, female wild-type C57BL/6 mice were divided into groups receiving either a PA or control diet for a 12-week feeding period. During this time, female mice on the PA diet exhibited a notable increase in body weight compared to the control group (Supplementary Fig. S1G). Following this dietary regimen, the mice were mated with healthy male counterparts and sacrificed on gestational Day 14 (D14). There was a marked elevation in ACBP protein expression and mRNA levels observed in mouse placentas as a result of PA exposure (Fig. 1J–L). Meanwhile, Comparative analysis revealed a higher incidence of embryo resorption in the PA treatment group compared to the control group (Fig. 1M and N). Additionally, the PA-exposed mice displayed significantly diminished average fetal and placental weights (Fig. 1O and P).
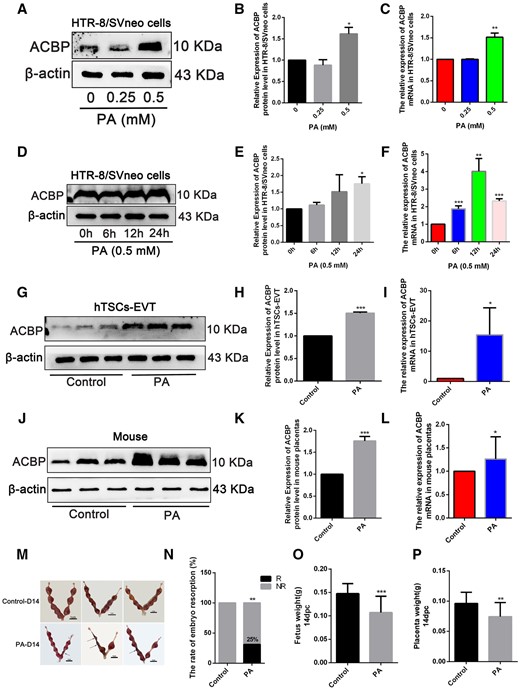
Palmitic acid (PA) exposure upregulated ACBP expression and impaired embryonic development. (A) Western blot of ACBP at 24 h after various concentrations of PA treatment in HTR-8/SVneo cells. (B) Quantified protein levels of ACBP, relative to the protein levels of β-actin. (C) HTR-8/SVneo cells were stimulated with various concentrations of PA, and the mRNA level of ACBP, examined 24 h after the treatment. (D) Western blot of ACBP at 6, 12, and 24h post-treatment with 0.5 mM PA. (E) Quantified protein levels of ACBP at 6, 12, and 24h post-treatment with 0.5 mM PA, relative to the protein levels of β-actin. (F) Quantitative analysis for ACBP mRNA. (G) The protein levels of ACBP detected by Western blot in hTSCs-EVT treated with 0.5 mM PA for 24 h. (H) Quantified protein levels of ACBP, relative to the protein levels of β-actin. (I) Quantitative analysis for ACBP mRNA. (J) Placental ACBP protein levels detected using Western blot. (K) Quantified protein levels of ACBP, relative to the protein levels of β-actin. (L) Quantitative analysis for ACBP mRNA. (M) The uterine appearance on D14 (n=10, Scale bar=1 cm). The black arrow indicates embryo resorption with severe uterine bleeding at D14. (N) Resorption rate of D14 in normal and PA-exposed mice (resorption implantation site per mouse/total implantation site per mouse). (O) Fetus weight (P) Placental weight. *P<0.05, **P<0.01, ***P<0.001. Each result is from three independent experiments.
PA exposure effects on placental autophagy in in vitro human trophoblasts and in vivo mouse placentas
We conducted a comprehensive assessment of how PA exposure affects placental and trophoblast autophagy both in vivo and in vitro. In PA-treated HTR-8/SVneo cells, both protein and mRNA expression of LC3B-II and P62 displayed a substantial increase, with changes dependent on both the concentration and the exposure time to PA (Fig. 2A–F). Additionally, autophagosomes notably increased following 24 h of PA exposure in HTR-8/SVneo cells (Fig. 2G), reinforces the notion that PA exposure stimulates autophagosome accumulation and causes autophagic dysfunction. Furthermore, we identified a significant upsurge in the protein and mRNA levels of lysosome-associated membrane proteins (Lamp1 and Lamp2) in PA-treated HTR-8/SVneo cells (Fig. 2H–J). Consistent with HTR-8/SVneo cells, 0.5 mM PA-treated hTSCs-EVT also exhibited a substantial increase in both protein and mRNA expression of LC3B-II and P62 (Fig. 2K–M). In mice placentas treated with PA, there was a noticeable increase in the protein expression of LC3B-II and P62, indicating disrupted autophagy (Fig. 2N–P). This trend was consistent with the levels of LC3B-II and P62 mRNA, which significantly rose due to PA treatment (Fig. 2Q and R). Simultaneous, TEM results showed that the number of autophagosome in the PA-fed group was significantly higher in the placental than that in control group (Fig. 2S).
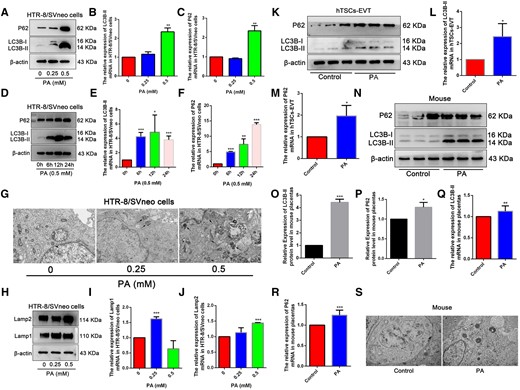
Palmitic acid (PA) exposure caused autophagic dysfunction in vitro and in vivo. (A) The protein levels of LC3B-II and P62 detected by Western blot in HTR-8/SVneo cells treated with different concentrations of PA for 24 h. (B, C) Quantitative analysis for LC3B-II and P62 mRNA by RT–qPCR. (D) The protein levels of LC3B-II and P62 detected by Western blot in HTR-8/SVneo cells treated with 0.5 mM PA for 6, 12, and 24 h. (E, F) Quantitative data of LC3B-II and P62 mRNA expression by RT–qPCR. (G) TEM images of HTR-8/SVneo cells exposed to different concentrations of PA for 24 h. Black arrows indicate autophagosomes. Scale bar: 1 μm. (H) Protein levels of Lamp1 and Lamp2 in HTR-8/SVneo cells exposed to different concentrations of PA for 24 h. (I, J) Quantitative data of Lamp1 and Lamp2 mRNA expression by RT–qPCR. (K) The protein levels of LC3B-II and P62 detected by Western blot in hTSCs-EVT treated with 0.5 mM PA for 24 h. (L, M) Quantitative analysis for LC3B-II and P62 mRNA. (N) Placental LC3B-II and P62 protein levels were detected using Western blot. (O, P) Quantified protein levels of LC3B-II and P62, relative to the protein levels of β-actin. (Q) (R) Quantitative analysis for LC3B-II and P62 mRNA by RT–qPCR. (S) Autophagy in mouse placenta tissue on D14 was observed by using TEM. The black arrow indicates autophagosome with a double membrane structure. Scale bar: 1 μm. *P<0.05, **P<0.01, ***P<0.001 compared with the control group. Each assay was repeated independently for three times.
Autophagic flow disruption in human EVT cells exposed to PA
We further validated the impact of PA exposure on autophagy modulation in EVT cell line HTR-8/SVneo and used CQ and Rap as control interventions. CQ, recognized as an autophagy inhibitor, is known to hinder autophagosome-lysosome fusion, leading to autophagosome accumulation. Our Western blot results demonstrated that co-treatment of PA and CQ induced significantly higher levels of LC3B-II and P62 proteins in HTR-8/SVneo cells compared to PA treatment alone (Supplementary Fig. S2A–C). And we observed a considerable increase of yellow spots in PA and CQ co-treated cells (Supplementary Fig. S2D). This outcome suggests that combining PA with CQ further obstructed the normal autophagic flow. Subsequently, we introduced Rap, an autophagy inducer, to the experiment. HTR-8/SVneo cells were subjected to treatment with 0, 0.25, or 0.5 mM PA in combination with or without Rap for 24 h. In contrast to the earlier findings, the combined treatment of Rap with PA mitigated the elevated levels of LC3B-II and P62 proteins induced by PA exposure (Supplementary Fig. S2E–G). Moreover, co-treatment of Rap with PA reduced the number of yellow spots in HTR-8/SVneo cells compared to treatment with PA alone (Supplementary Fig. S2H). These results provided additional evidence of how PA exposure perturbs autophagy in trophoblast cells.
ACBP knockdown mitigates PA-induced impairment of human trophoblast autophagy
To shed light on the interplay between autophagy and ACBP expression in in vitro, we employed Rap, and CQ, in the culture medium of HTR-8/SVneo cells, respectively. Notably, ACBP expression exhibited an anticipated increase following treatment with CQ (Supplementary Fig. S3A and B). Conversely, when compared to the group without Rap, ACBP expression exhibited a reduction with Rap treatment (Supplementary Fig. S3C and D). These observations suggest that variations in ACBP expression are indeed linked to the autophagy level in HTR-8/SVneo cells.
To further explore the impact of ACBP on HTR-8/SVneo cells function as exposure to PA, we established an ACBP knockdown cell line (Supplementary Fig. S3E and F) using shRNAs targeting to ACBP. As depicted in Supplementary Fig. S3G–I, ACBP knockdown resulted in decreased expression of LC3B-II and P62 proteins and mRNA. Furthermore, when we compared the effects of PA exposure alone and the combined treatment of PA and ACBP knockdown, it was evident that the latter induced a substantial increase in LC3B-II and P62 protein and mRNA levels in HTR-8/SVneo cells (Supplementary Fig. S3J–M).
ACBP knockdown alleviates PA-induced impairment of mouse placental autophagy
To delve deeper into the impact of ACBP on PA-induced abnormal development of mouse placentas in an in vivo setting, we divided ACBPflox/flox female mice into two groups. One group received a standard control diet, while the other was provided with normal mouse chow supplemented with 5% PA. After 12 weeks of dietary intervention, both groups were mated with ACBPflox/flox/ELF5-Cre males to obtain fetuses with either the ACBPflox/flox (Control) or ACBPflox/flox/ELF5-Cre (CKO) genotypes, either exposed to PA or not. Among pregnant mice exposed to PA, a noticeable increase in absorbed fetuses was observed among the Control group when compared to the CKO group in terms of absorbed fetuses (Fig. 3A–C). Furthermore, the combination of CKO with PA treatment increased body weight in fetal mice and their respective placentas, when compared to PA treatment alone (Fig. 3D and E).

Palmitic acid (PA) exposure favors embryonic development in placental ACBP-conditioned knockout mice. (A) Uterus anatomy of D14 in PA group. (B) Resorption rates in PA exposed and CKO combined PA exposed mice. (C) Embryonic resorption rate of D14 in normal mice and mice exposed to CKO combined with PA. (D) Placental weight. (E) Fetus weight. (F) Placental ACBP, LC3B-II, and P62 protein levels were detected using Western blot. (G–I) Quantified protein levels of ACBP, LC3B-II, and P62, relative to the protein levels of β-actin; *P<0.05 compared with the control group, **P<0.01. ***P<0.001. #P<0.05 compared with the CKO group. Each result is from three independent experiments.
Changes in autophagic function were subsequently examined, revealing that LC3B-II and P62 proteins were reduced in CKO mouse placentas compared with the Control group (Fig. 3F). Western blot results demonstrated that when PA was combined with CKO treatment, the protein levels of ACBP, LC3B-II, and P62 significantly increased compared to the placenta of CKO mice alone, essentially maintaining normal levels (Fig. 3G–I). These findings suggest a potential protective role of ACBP in mitigating PA-induced autophagic flux injury.
Discussion
It has been observed that maternal consumption of a high-fat diet can have detrimental effects on placental health (Ceasrine et al., 2022). Notably, the adverse impact of a high-fat diet in mothers is particularly associated with saturated fatty acids, most notably PA (Rocha et al., 2017). Various studies have indicated that PA may disrupt placental development by triggering inflammatory responses in trophoblastic cells (Rampersaud et al., 2020). Although there is some evidence linking PA to pregnancy complications (Lager et al., 2014; Sano et al., 2020), the precise molecular mechanisms behind how PA leads to adverse pregnancy outcomes and impacts fetal development remain inadequately understood. In our study, we present findings that underscore the adverse effects of PA exposure in trophoblast cells of human and mouse. These results shed light on the potential mechanisms underlying the link between high-fat diets, PA, and adverse outcomes during pregnancy and placental development in humans.
ACBP, also known as fatty acid-binding protein, plays a crucial role in the metabolism and utilization of fatty acids in a variety of cellular processes (Duman et al., 2019; Lebrun et al., 2021). In individuals affected by obesity, ACBP levels are anomalously elevated, making it one of the few appetite stimulators heightened in human obesity (Joseph et al., 2020). Throughout the developmental stages, from early embryogenesis to adulthood in mammals, ACBP is consistently expressed and is recognized as a central hub in lipid metabolism (Burgi et al., 1999). Our study showed that PA induction increased ACBP expression in human trophoblasts in vitro and mice in vivo. Elevated ACBP expression correlated with lower fetal and placental weights in mice. This suggests that ACBP might play a role in the functional development of placenta and could be linked to PA-induced abnormalities in human or mouse embryonic development.
Autophagy, a dynamic process involving lysosome-mediated degradation of cellular components and foreign substances, encompasses various stages, including initiation, formation, maturation, and the eventual degradation of autophagosomes, collectively known as autophagic flux (Mittal et al., 2017; Yang and Lin, 2023). Dysregulated autophagy may induce placental dysfunction by failing to maintain homeostasis in vivo, thereby affecting a variety of cellular behaviors (Zhao et al., 2020). Studies have shown that PA promotes autophagosome formation and reduces autophagosome degradation during in vitro development of mouse embryos before implantation and reduces blastocyst development (Leung et al., 2022). In our current study, exposure to PA before and during pregnancy in mice resulted in increased levels of the autophagic proteins LC3B-II and P62 in the placenta. Similarly, PA exposure increased the levels of LC3B-II and P62, along with the number of autophagic vesicles, in HTR-8/SVneo cells. Moreover, exposure to PA also increased the levels of LC3B-II and P62 in hTSCs-EVT. These findings strongly suggest that PA may impair placental development by disrupting the autophagic process. Furthermore, the presence of autophagic flux blockage in PA-treated cells suggests that autophagy plays a pivotal role in maintaining placental homeostasis. The impairment of autophagic flux may, in part, be responsible for the accumulation of autophagosomes resulting from PA exposure. These insights shed light on the potential mechanistic link between PA, autophagy dysfunction, and their effects on placental and embryonic health.
Notably, ACBP proteins have been observed to inhibit autophagy and induce obesity in mice (Leidal et al., 2018; Lopez-Otín and Kroemer, 2019), thus highlighting the interplay between ACBP and autophagy in the context of a high-fat diet (Udupa et al., 2023). Consequently, PA exposure might disrupt placental autophagy function by altering ACBP expression, consequently impairing placental function in both humans and mice. Then, by administering PA in combination with CKO treatment, we observed a significant increase in the protein levels of ACBP, LC3B-II, and P62 compared to CKO mice alone, effectively restoring them to normal levels in the placenta. These findings were consistent with the results obtained from HTR-8/SVneo cells, strengthening the validity of our observations across both experimental models. Collectively, our results underscore the crucial role of ACBP in the context of PA-induced autophagy dysfunction, which is closely associated with impaired placental function.
Despite our efforts to utilize primary EVT cells from patients, we encountered challenges as these cells were fragile and did not survive exposure to PA. This limitation hindered further experimentation. Additionally, while the HTR-8/SVneo cell line is useful for simulating trophoblast behavior in placental research, its origin from a transformed cell line may not fully reflect the complexity of primary trophoblasts in vivo, potentially impacting signaling pathways and gene expression. Furthermore, its immortalized nature may compromise its translatability to clinical settings. To address these concerns, we employed EVT cells derived from hTSCs, aiming to enhance the robustness and transparency of our findings. Despite logistical challenges in studying the persistence of placental dysfunction post-PA exposure cessation in mice, existing literature suggests potential amelioration through dietary modification (Wallace et al., 1999; Salati et al., 2019). Additionally, the complexity of placental issues extends beyond preimplantation embryo exposure to high-fat diets, highlighting the intricate interplay between maternal nutrition, embryo development, and placental function (Cross et al., 1994; Bellver et al., 2010; Jungheim et al., 2010). Further investigations are warranted to elucidate the underlying mechanisms of PA-induced placental dysfunction.
In summary, our study elucidates the mechanistic impact of PA on placental development, emphasizing the critical need for meticulous control of fat-rich diets before and during pregnancy.
Data availability
The data underlying this article will be shared on reasonable request to the corresponding author.
Acknowledgements
We express our gratitude to the clinicians for their invaluable assistance in harvesting placental villi.
Authors’ roles
Y.-B.D., L.-J.F., and X.-Y.C. initiated and planned the project. Y.Z., M.-R.L., Q.F., Y.-L.X., and L.Y. executed the experiments and wrote the manuscript. Y.Z., F.-F.L., and J.T. conducted data analysis and oversaw the project. Y.-B.D., Y.Z., L.-L.R., and Y.Z. revised the manuscript. The final manuscript was reviewed and approved by all authors.
Funding
National Natural Science Foundation of China (82171664, 82301909); Natural Science Foundation of Chongqing Municipality of China (CSTB2022NS·CQ-LZX0062, cstc2019jcyj-msxmX0749, cstc2021jcyj-msxmX0236).
Conflict of interest
The authors declare that they have no conflict of interest.
References
Author notes
Yi Zhang and Ling-Ling Ruan authors contributed equally to this work.