-
PDF
- Split View
-
Views
-
Annotate
-
Cite
Cite
Weichao Liu, Tangchun Zheng, Like Qiu, Xiaoyu Guo, Ping Li, Xue Yong, Lulu Li, Sagheer Ahmad, Jia Wang, Tangren Cheng, Qixiang Zhang, A 49-bp deletion of PmAP2L results in a double flower phenotype in Prunus mume, Horticulture Research, Volume 11, Issue 2, February 2024, uhad278, https://doi.org/10.1093/hr/uhad278
- Share Icon Share
Abstract
The double flower is an important trait with substantial ornamental value. While mutations in PETALOSA TOE-type or AG (AGAMOUS) genes play a crucial role in enhancing petal number in ornamental plants, the complete mechanism underlying the formation of double flowers remains to be fully elucidated. Through the application of bulked segregant analysis (BSA), we identified a novel gene, APETALA2-like (PmAP2L), characterized by a 49-bp deletion in double-flowered Prunus mume. β-Glucuronidase (GUS) staining and luciferase reporter assays confirmed that the 49-bp deletion in PmAP2L reduced its binding with Pmu-miRNA172a. Phylogenetic analysis and microsynteny analysis suggested that PmAP2L was not a PETALOSA TOE-type gene, and it might be a new gene controlling the formation of double flower in P. mume. Subsequently, overexpression of PmAP2L-D in tobacco led to a significant rise in the number of stamens and the conversion of stamens to petals. Furthermore, silencing of the homologue of RC5G0530900 in rose significantly reduced the number of petals. Using transient gene expression in P. mume flower buds, we determined the functional differences between PmAP2L-D and PmAP2-S in controlling flower development. Meanwhile, DNA-affinity purification sequencing (DAP-seq), yeast hybrid assays and luciferase reporter assays indicated that PmAP2L negatively regulated the floral organ identity genes by forming a repressor complex with PmTPL and PmHDA6/19. Overall, these findings indicate that the variation in PmAP2L is associated with differences in the regulation of genes responsible for floral organ identity, providing new insights into the double-flower trait and double-flower breeding in plants.
Introduction
The flowers of angiosperms undergo at least four developmental processes: floral organ initiation and determination, morphogenesis and maturation [1, 2]. Changes in these processes induce significant variations in flowering time, size, color, arrangement, and the number of floral organs, thereby contributing to the diverse and vibrant floral palette observed in angiosperms [1, 3]. The initiation and determination of floral organs are regulated by the ABC model of flower development, which was first inferred through a set of homeotic genes identified in Arabidopsis thaliana and Antirrhinum majus [4–6]. According to the ABCDE model, five classes of floral organ identity genes control the four types of floral organs. The identity of sepals is regulated by the combination of A and E class genes, whereas the determination of petals involves A, B, and E class genes. Carpels, on the other hand, are defined by the presence of B, C, and E class genes [6].
AGAMOUS (AG), as the earliest cloned C-class gene, serves a dual role in both terminating and maintaining the floral meristem [7, 8]. In ag mutants, A class genes exhibit persistent expression in the third and fourth whorl of flower organs, resulting in ‘flower-within-flower’ phenotype [4, 8]. Hence, numerous studies have concentrated on the genetic variations caused by AG gene, especially the double flower trait. Analysis of ThtAG1 alleles in Thalictrum thalictroides showed that the insertion of a 2209 bp long terminal repeat (LTR) in exon 4 induced a nonsense mutation with K-domain deletions, and the mutated protein abolished interactions with the E class protein ThtSEP3 [9]. AG from the double flowers in Prunus serrulate and Magnolia stellata showed exon skipping as compared to the single flowers, whereas AG in Japanese Kerria (Kerria japonica) and yellowhorn (Xanthoceras sorbifolium) showed a transposon-mediated insertion [10–13]. Furthermore, AP2, a member of A-class genes, antagonizes the transcriptional activity of the C-class genes and is closely associated with the formation of double flowers. In strong ap2 mutants, the expression of AG is intensified in the first and second floral whorls, leading to the loss of petals [4, 14]. The double flower phenotype in peach and rose is correlated with the absence of the miR172 target site in PETALOSA TOE-type genes [15]. Specifically, the lack of the 10th exon in Prupe.6G242400 in peach induces premature termination, resulting in the expression of a truncated mRNA that escapes regulation by miR172 [15]. It is noteworthy that similar variants of PETALOSA TOE-type genes were found in Rosa rugosa, Rosa Chinensis, Prunus persica, Petunia hybrida, and Dianthus genus [15–19]. From the preceding discussion, it is evident that the variations in AG or PETALOSA TOE-type genes predominantly contribute to the formation of double flowers.
Prunus mume (Mei), a member of the Rosaceae family, has been domesticated as an ornamental plant, particularly across the entire East Asia. It is one of the earliest ornamental plants globally to undergo comprehensive studies involving whole genome assembly and resequencing [20–22]. During the long-term cultivation and selective breeding, P. mume has gradually developed a rich diversity of flower types, emerging as a rare material to study the genetics of floral organs in the Prunus genus and Rosaceae. Double flowers with excessive petals hold a significant ornamental value. The double flower trait in P. mume was first identified through genome wide association studies (GWAS) within a 3.6 Mb region on Chr1 [21].
To determine the cause of double-flower formation in P. mume, we pinpointed the candidate locus via BSA-seq and successfully identified the PmAP2L gene. We found that a 49-bp deletion in PmAP2L negatively regulated the multiple floral organ identity genes by affecting miR172a-PmAP2L interaction. Our results reveal the regulatory network of PmAP2L in double flower formation and provide a new perspective for studying the double-flowers in the Prunus genus or Rosaceae.
Results
Double flower morphology, development, and genetic analysis in P. mume
Female parent 'Mi Danlv' (MDL, single flower with five petals) and pollen donor 'Baixu Zhusha' (BXZS, double flowers with an average petal number of 24.1) were used as cross parents (Fig. S1a and Table S1, see online supplementary material). Comparison of the developmental process of single and double flower buds by SEM revealed a clear difference between the two types of buds after third stage of flower development (Fig. 1a). Double buds produced more petal primordia than single buds after differentiation of sepal primordia. As a result, at stage 5, the petal primordia in double buds completed their development into a petal sheet structure, and their number significantly exceeded that of single buds (Fig. 1a). In a blooming flower, the size of the petals gradually decreased from the outside to the inside, with clear traces of stamen petalization (Fig. S1b, see online supplementary material).
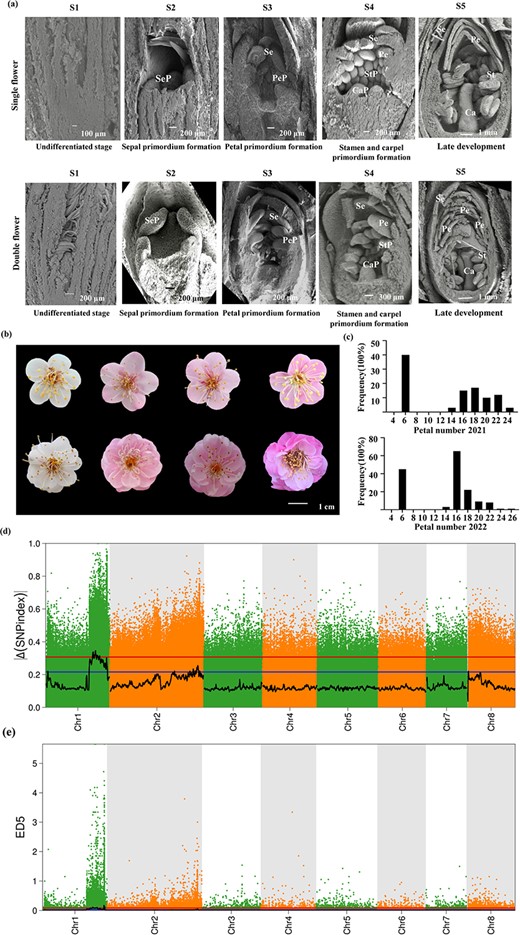
Morphology and gene locus of the double flower trait in Prunus mume. (a) SEM imaging of floral organ primordium at different developmental stages from single flower (upper panels) and double flower (lower columns). S1: undifferentiated stage; S2: sepal primordium formation; S3: petal primordium formation; S4: stamen primordium and carpel primordium formation; S5: late development. Sep, Se, PeP, Pe, StP, St, CaP, and Ca represent sepal primordium, sepal, petal primordium, petal, stamen primordium, stamen, carpel primordium, and carpel, respectively. (b) Flower morphology of different offsprings in the F1 segregation population. (c) Histogram of the frequency distribution of the number of petals in an F1 segregation population in 2021 and 2022. (d) Scatter diagram of the ΔSNP index. The black line indicates the fitted ΔSNP index value, and the blue and red lines represent thresholds of 99% and 95%, respectively. (e) Scatter diagram of ED^5. The black line indicates the fitted ED^5 value, and the red dotted line indicates the significance association threshold.
The F1 population in P. mume was generated by a cross between MDL and BXZS. The double-flower trait was particularly distinct in the F1 hybrids (Fig. 1b). The single-flower progeny had five petals, while the double-flower progeny had multiple layers of petals (Fig. 1b). Petal number in the F1 hybrid population ranged from 5 to 24 in 2021 and from 5 to 25.1 in 2022 (Fig. 1c; Table S1, see online supplementary material). The distribution of petal number was similar in both years, with a discontinuous bimodal distribution, consistent with the inheritance of qualitative traits caused by a single gene (Fig. 1c).
The major gene plus polygene mixed inheritance model was used to analyse double flower trait in 2021 and 2022. These candidate models were selected based on the minimum criteria of Akaike’s Information Criterion (AIC). The AIC values for 11 genetic models of the number of petals of F1 population are presented in Table S2 (see online supplementary material). A-1 and A-3 were chosen for petal number in 2021 and 2022, with the same priority order. Based on lower AIC values, the final candidate model was A-1, indicating that double flower phenotype was controlled by one-pair additive-dominant major genes. In 2021 and 2022, the additive effects, dominant effects, and major-gene heritability were as follows: 8.3290, 3.9004, and 96.1415% for 2021, and 6.8006, 3.4087, and 85.4499% for 2022, respectively (Tables S3 and S4, see online supplementary material). By comparing the frequency distribution with the theoretical distribution curve, it was found that the models for both years fitted well, and the range predicted by the model was very close to the actual distribution (Fig. S2, see online supplementary material).
BSA-seq
To identify the key genetic locus controlling the double flower formation in P. mume, DNA from 64 double-flowered plants and 41 single-flowered plants was mixed and used for BAS-seq. The double and single flower pools generated a total of 112 million and 83 million high-quality raw reads, respectively (Q20 ≥ 96.34%, Q30 ≥ 91.26%) (Table S5, see online supplementary material). A total of 97.86% and 97.77% of the filtered data from the bulked samples were aligned to P. mume reference genome with more than 10 × depth (Table S6, see online supplementary material). Finally, 1 589 316 single nucleotide polymorphisms (SNPs) and 318 316 insertions or deletions (IN/DELs) were detected in double-flowered pool and 1 583 269 SNPs and 314 603 IN/DELS were identified in single-flowered pool (Tables S7 and S8, see online supplementary material). After eliminating the low-quality variants in two pools, 1 048 575 variants were identified across all eight chromosomes (Dataset S1, see online supplementary material).
After calculating the SNP index and the delta SNP index in the pools and plotting them against the genome positions, two candidate regions on chromosome 1 were mapped to a physical distance of 0.59 Mb from 26 720 001 to 27 310 000 bp and 3.38 Mb from 23 190 001 to 26 570 000 bp (99% confidence threshold, Fig. 1d and Table S9, see online supplementary material). In addition, two loci were also mapped on chromosome 1 by using the ED algorithm. One of the loci was located within a 0.99-Mb region from 30 180 001 to 31 170 000 bp, and the other was located within a 3.03-Mb region from 24 390 001 to 27 420 000 bp (99% confidence threshold, Fig. 1e and Table S10, see online supplementary material). The regions identified by SNP index and the ED method showed overlap. Considering the high heterozygosity of woody plants, we defined the union of the two results as the candidate interval, which was 4.23 Mb and 0.99 Mb, for subsequent analysis (Tables S9 and S10, see online supplementary material). Interestingly, the interval for the double-flower trait identified in this study partially overlapped with the region identified by our previous GWAS study [21], with a sharing of 214 genes (Dataset S2, see online supplementary material). Among these genes, there was an AP2/ERF transcription factor (TF), which belonged to the A-class genes and was named as PmAP2-like (PmAP2L). Additionally, both delta SNP index and the ED methods also showed a partially overlapping region on chromosomes 2 and 8 (95% confidence thresholds, Tables S11 and S12, see online supplementary material). This region was worthy to investigate the potential presence of new genes interacting with PmAP2L and influencing the formation of double flowers.
A 49-bp deletion in PmAP2L affected miR172a-PmAP2L interaction
To confirm the potential involvement of PmAP2L in the formation of double flowers, we cloned the CDS of PmAP2L from the single and double flower individuals. Comparing with PmAP2L-S (PmAP2L from single-flowered individuals), a 49-bp deletion in the C-terminus was found in the PmAP2L-D (PmAP2L from double-flowered individuals) (Fig. S3, see online supplementary material). Furthermore, we sequenced the CDS of PmAP2L from other varieties with different flower types. Interestingly, the deletion was also observed in two double-flowed varieties, whereas one single-flowered did not show this deletion (Fig. S3, see online supplementary material). In addition, we cloned fragments based on the 49-bp deletion region of gDNA from the double flower pool and single flower pool of the F1 population, as well as from randomly selected pools of 10 single and 10 double flower varieties. Notably, the 49 bp deletion was also present in both the double-flowered progenies and double-flowered varieties compared to the single-flowered pools (Fig. S4, see online supplementary material). Moreover, a cleaved amplified polymorphic sequences (CAPS) marker was developed based on the 49-bp deletion, which was closely associated with the double flower trait (Fig. S4, see online supplementary material). The accuracy of the CAPS marker was 96.62%, suggesting that the 49-bp deletion of PmAP2L was closely associated with the double flower trait (Fig. S5, see online supplementary material).
Phylogenetic analysis revealed that PmAP2L belonged to the TOE-type genes and it was clustered in the same clade with Arabidopsis_TOE1, Rosa_XP_024157287, and Peach_Prupe.6G091100 (Fig. 2a). Moreover, Rosa_XP_024186592, Peach_Prupe.6G242400, Dianthus_Dca21030, and Petunia_PhBOB, which were reported to be associated with the double flower formation, were distributed in the same branch, also known as PETALOSA TOE-type. Mei_PmuVar_chr1_1333 was also distributed in this branch. The sequence alignment and conserved domain distribution suggested that the gene structures and motif arrangements were similar in both branches, but PETALOSA TOE-type genes did not contain motif 10 (Fig. S6a–c, see online supplementary material). The sequence similarity analysis showed that the similarity index among PETALOSA TOE-type genes was 59%–98%. The similarity index among Mei_PmAP2L-D/S, Arabidopsis_TOE1, Rosa_XP_024157287, and Peach_Prupe.6G091100 was 60%–99% (Fig. S6d, see online supplementary material). Moreover, the two branches were clearly distinguishable based on the domain distribution and the similarity index (Fig. S6c–d, see online supplementary material). Through microsynteny analysis between PmAP2L locus and PETALOSA TOE-type genes locus, we found that mei PmAP2L locus exhibited strong collinear relationship with rose RC5G0530900 locus on chromosome 5 and peach Prupe.6G091100 locus on chromosome 6. The rose RcAP2L (Rc5G0243000) locus and the peach Prupe.6G242400 locus showed strong collinear relationship with mei PmuVar_Chr1_1333 locus (Fig. S6e–f, see online supplementary material). These results suggested that PmAP2L was not a PETALOSA TOE-type gene. It might be a new gene controlling the formation of double flower in P. mume.
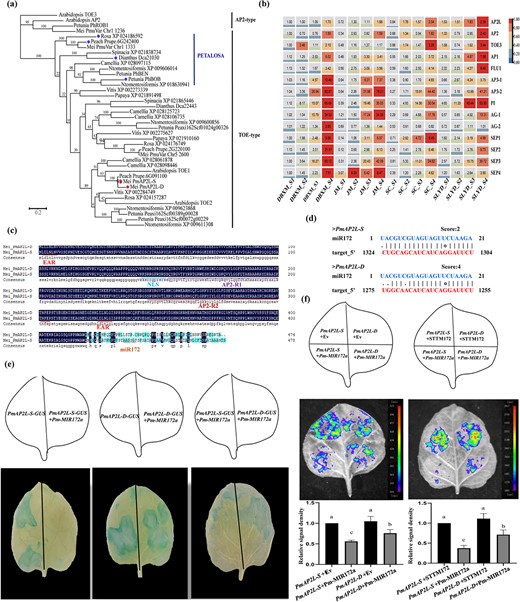
Phylogenetic and expression pattern of PmAP2L and the interaction of miR172 and PmAP2L. (a) Evolutionary relationships among euAP2 (AP2- and TOE-type) members in Prunus mume and other species. (b) Expression heatmap of PmAP2L and other ABCDE model genes at different stages in different flower type varieties of P. mume. (c) Multiple sequence alignment analysis of PmAP2L-D and PmAP2L-S. (d) Prediction of miR172 on PmAP2L-S/D in P. mume. (e) GUS histochemical staining. 35S::PmAP2L-S-GUS is a vector containing PmAP2L-S. 35S::PmAP2L-D-GUS is a vector containing PmAP2L-D. 35S::Pmu-pre-miR172a is a vector containing mature miR172a. The experiments were independently repeated three times. (f) Validation of the miR172a-PmAP2L interaction in vivo. Empty-SK refers to the empty vectors. 35S::LUC-PmAP2L-S is a vector containing the miR172 target site fragments of PmAP2L-S. 35S::LUC-PmAP2L-D is a vector containing the miR172 target site fragments of PmAP2L-D. SK-Pm-MIR172a is a vector containing mature miR172a. SK-STTM172 is a vector containing a short tandem target mimic miR172 fragment (STTM172). The experiments were independently repeated three times. The upper panels represent the mixed injection of vectors, the middle panels show representative leaf images, and the lower columns represent the statistical analyses of relative luminescence intensities. The relative luminescence intensities were calculated with the value of PmAP2L-S + EV combination (left bar chart) and PmAP2L-S + STTM172 combination (right bar chart) as 1, respectively. Data are represented as the mean ± SD of five biological replicates (n = 5). Different letters above the bars indicate a significant difference (P < 0.05, one-way ANOVA).
PmAP2L possesses several conserved domains, including the two AP2 domains (AP2-R1 and AP2-R2), nuclear localization sequence (NLS), and two typical ethylene-responsive element binding factor-associated amphiphilic repression (EAR) motifs (Fig. 2c). To further determine whether there were differences in the target sites, we used the TAPIR online tool to identify the miR172 target site in PmAP2L. Interestingly, the 49 bp deletion was spanned in the miR172 target sequence, causing sequence differences between the miR172 cleavage site of PmAP2L-D and PmAP2L-S (Fig. 2d). The miR172 target site differed by only two bases in the sequence of PmAP2L-S from single flower plants, while the double flower plants differed by four bases (Fig. 2d).
To explore the prospective function of PmAP2L in controlling flower development, as well as the differential regulation by miR172, we measured the relative expression levels of PmAP2L in the buds of single and double flower individuals. As shown in Fig. S7 (see online supplementary material), the expression of PmAP2L in the double-flower individual was significantly higher than that of the single flower individual, possibly due to the 49-bp deletion leading to a decrease in the ability of miR172 to bind to the PmAP2L-D. In addition, we selected 14 genes in ABCDE model for gene expression patterns in four varieties, including single flower varieties DBXM and JM, and double flower varieties SC and SLYD (Fig. 2b). The transcript level of the A-class genes (PmAP2L, PmAP2, PmTOE3, PmAP1, and PmFLU1) increased gradually during floral bud differentiation (Fig. 2b). Notably, except for the undifferentiated stage (S1), the expression of PmAP2L was significantly high in double-flower varieties as compared to single-flowered, consistent with the results of flower buds of F1 population (Fig. 2b). Regarding class B genes, PmAP3–1, PmAP3–2, and PmPI showed a gradually increasing trend during flower development. Especially, the expression level of PmAP3–2 and PmPI rose sharply after the petal primordium formation stage (S3) (Fig. 2b). PmAG-1 and PmAG-2 expressed during the petal primordium formation stage (S3), and the expression level was higher in single flowers as compared to double-flower varieties at S3–S4. This suggested that the expression of PmAG-1 and PmAG-2 was correlated with the formation and differentiation of petal primordia (Fig. 2b). The transcript of the E-class genes PmSEP1/2/3/4 increased gradually at S1–S4, with PmSEP3 showing the highest expression level at the primordium formation stage of stamen and carpel (Fig. 2b).
The interaction between miR172 and PmAP2L was validated by GUS histochemical staining and luciferase imaging assay. Compared with PmAP2L-D and PmAP2L-S group, the decreased GUS expression in PmAP2L-D-GUS + Pm-MIR172a and PmAP2L-S-GUS + Pm-MIR172a group suggested that miR172a could target and degrade the mRNA of PmAP2L-D and PmAP2L-S (Fig. 2e). Interestingly, the reduction in GUS expression in PmAP2L-D-GUS + Pm-MIR172a group was considerably weaker when compared to PmAP2L-S-GUS + Pm-MIR172a group (Fig. 2e). This indicated that miR172a in the double-flower alleles had a lower cleaving efficiency of PmAP2L than that in the single-flower alleles. The results of the LUC luminescence signal are shown in Fig. 2f. The control group of PmAP2L-D/S-LUC + Empty-SK and PmAP2L-D/S-LUC + SK-STTM172 produced a strong luminescence signal, while the signals of PmAP2L-S-LUC + SK-Pm-MIR172a and PmAP2L-D-LUC + SK-Pm-MIR172a groups were weak. These results indicated that PmMIR172a had an inhibitory effect on PmAP2L-D and PmAP2L-S, hindering the expression of the LUC and making the signal weaker. Importantly, the LUC luminescence signal of PmAP2L-D-LUC + SK-Pm-MIR172a group was significantly higher than that of PmAP2L-S-LUC + SK-Pm-MIR172a group, indicating that the efficiency of miR172 in cutting the PmAP2L in the double-flower alleles was lower than that in the single flower alleles. The interaction between miR172 and PmAP2L was validated by the dual luciferase reporter assay.
PmAP2L-D but not PmAP2L-S regulates stamen petaloids
The results of subcellular localization showed that the green fluorescent protein (GFP) signal of the control group was detected in the nucleus and cell membrane, while PmAP2L-D and PmAP2L-S were only localized to the nucleus (Fig. 3a).
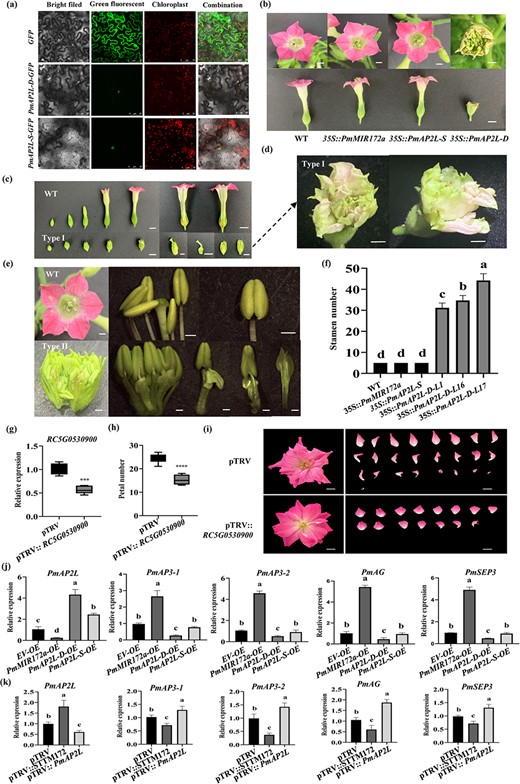
The function of PmAP2L-D in regulating stamens into petals. (a) Subcellular localization of PmAP2L-S/D in tobacco leaves. (b) Phenotypic observation of 35S::Pmu-pre-172a, 35S::PmAP2L-S, and 35S::PmAP2L-D transgenic tobacco plants. Scale bar = 1 cm. (c)35S::PmAP2L-D tobacco (type I) show the formation of two rounds of floral structures within the sepals, forming another corolla within the corolla. Scale bar = 1 cm. (d) Amplification of the type I phenotype. Scale bar = 1 cm. (e)35S::PmAP2L-D tobacco (type II) show the transformation of stamens to petals. Scale bar = 1 cm. (f) The number of stamens in transgenic tobacco with miR172a, PmAP2L-S, and PmAP2L-D genes. Data are represented as the mean ± SD of five biological replicates (n = 5). Different letters above the bars indicate a significant difference (P < 0.05, one-way ANOVA). (g) qRT-PCR of RC5G0530900 in TRV control and silenced plants. The mean ± SD from three biological replicates (n = 3) are shown. Asterisks indicate statistically significant differences (two-sided Student's t test; ***P < 0.001). (h) The number of petals in TRV and TRV-RC5G0530900. The mean ± SD from 12 biological replicates (n = 12) are shown. Asterisks indicate statistically significant differences (two-sided Student's t test; ****P < 0.0001). (i) Images of TRV and TRV- RC5G0530900 infected plants were taken 50 d after infiltration. (j) qRT-PCR of PmAP2L and floral development genes (PmAP3–1, PmAP3–2, PmAG, and PmSEP3) in flower buds after PmAP2L-D/S and PmMIR172a overexpression. EV, empty vector; OE, overexpression. The mean ± SD from three biological replicates (n = 3) are shown. Different letters above the bars indicate a significant difference (P < 0.05, one-way ANOVA). (k) qRT-PCR of PmAP2L and floral development genes (PmAP3–1, PmAP3–2, PmAG, and PmSEP3) in flower buds after VIGS treatment. The mean ± SD from three biological replicates (n = 3) are shown. Different letters above the bars indicate a significant difference (P < 0.05, one-way ANOVA).
In order to verify the functions of PmAP2L-D/S and miR172 in the regulation of flower organ development, we overexpressed the Pmu-pre-miR172a (miR172-OE), PmAP2L-S (PmAP2L-S-OE), and PmAP2L-D (PmAP2L-D-OE) in tobacco leaves by Agrobacterium-mediated transformation. The quantitative real-time (qRT)-PCR analysis showed that the expression levels of miR172a, PmAP2L-S, and PmAP2L-D in the leaves of transgenic lines were higher than those in WT. The expressions of miR172a, PmAP2L-S, and PmAP2L-D were increased by more than 150, 50, and 300-fold, respectively, in the severely affected transgenic plants (Fig. S8, see online supplementary material). Phenotypically, all miR172a-OE and PmAP2L-S-OE transgenic plants showed no significant difference in flower color, stamen, pistil, and other traits compared to WT plants (Fig. 3b). However, three of the PmAP2L-D-OE plants (OE1, OE16, and OE17) were found to have small flower organs and light flower color, along with an increase in the number of stamens, reaching up to 44 stamens (Fig. 3c–f). The PmAP2L-D-OE lines had two major types of stamens turned into petals with downregulation ofendogenous genes related to flower development (Fig. S9, see online supplementary material). Type I transgenic lines showed smaller flower organs and shorter corollas compared to WT (Fig. 3c). The flower organs exhibited incomplete development, as vertical sections revealed the formation of two rounds of floral structures within the sepals. This resulted in the creation of an additional corolla within the original corolla, with stamens and pistils still present, showing incomplete development of the flower organs (Fig. 3c–d). Type II transgenic lines showed an increase in the number of stamens, with some stamens becoming smaller, and the transformation of stamens to petals (Fig. 3e). These results indicated that the 49-bp deletion reduced post-transcriptionally the regulation of PmAP2L-D by miR172.
Furthermore, we selected rose, which is closely related to P. mume in the Rosaceae family, to further characterize the AP2L and determine whether knockdown of AP2 homologs would reduce petal number. Using the same sequence (78 amino acids) shared between PmAP2L and the rose homologue RC5G0530900 (Fig. S10, see online supplementary material), a VIGS vector was constructed to reduce the expression level of RC5G0530900. Compared with TRV-only controls, the expression level of RC5G0530900 was decreased by 34% in TRV-RC5G0530900 lines, and the petal number of RC5G0530900-silenced plants was significantly decreased (Fig. 3g–i).
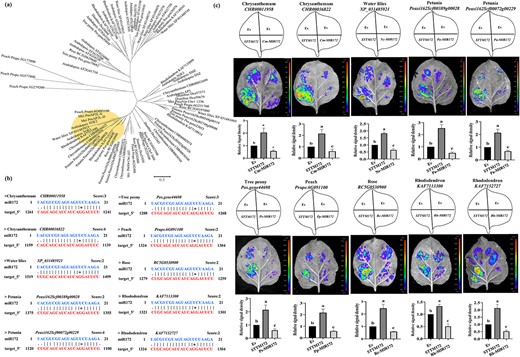
Analysis of miR172 regulation on AP2L in various ornamentals. (a) Phylogenetic tree analysis of AP2Ls in plants. (b) Prediction of miR172 on AP2L homologs in other ornamental plants. (c) Validation of the miR172-AP2L interaction in different ornamental plants. The upper panels represent the mixed injection of vectors, the middle panels show representative leaf images, and the lower columns represent the statistical analyses of relative luminescence intensities. The relative luminescence intensities were calculated with the value of EV combination as 1, respectively. Data are represented as the mean ± SD of five biological replicates (n = 5). Different letters above the bars indicate a significant difference (P < 0.05, one-way ANOVA).
To further verify the function of PmAP2L-D/S and miR172 in the regulation of floral organ development, we generated PmAP2L and Pmu-pre-miR172a overexpression and silencing vectors. The vectors were transferred into P. mume flower buds using agroinfiltration. qRT-PCR results showed that the expression of PmAP2L was significantly increased after the overexpression of PmAP2L-D/S and silencing of PmMIR172 (Fig. 3j and k). In contrast, the expression of PmAP2L was significantly reduced after the overexpression of PmMIR172 and silencing of PmAP2L (Fig. 3j and k). Subsequently, the expression levels of floral development genes (PmAP3–1, PmAP3–2, PmAG, and PmSEP3) were reduced after the overexpression of PmAP2L-D/S and silencing of PmMIR172. Contrarily the expression levels of these genes were increased after the overexpression of PmMIR172 and silencing of PmAP2L (Fig. 3j and k). Notably, overexpression of PmAP2L-D inhibited the expression of PmAP3–1, PmAP3–2, PmAG, and PmSEP3 genes more significantly than that of PmAP2L-S (Fig. 3j and k). Thus, these results suggested the functional differences between PmAP2L-D and PmAP2-S in the regulation of flower development in P. mume.
mi172-AP2L module regulates the double-flower trait in various ornamentals
To examine the potential conservation of the miR172-AP2L module in regulating flower organ development, AP2 genes from eight ornamental plants (petunia, rose, peach, tree peony, water lily, rhododendron, carnation, and chrysanthemum) and Arabidopsis were selected to construct a phylogenetic tree (Fig. 4a; Fig. S11, see online supplementary material). It was found that except for carnations, other plants had AP2L homologous genes that were clearly clustered with mei PmAP2L-D/S and AtTOE1 (Fig. 4a, Fig. S10, see online supplementary material). Bioinformatics predictions found that the subfamily of AP2L related to the double-flower trait had high sequence complementarity with miR172, and the homology of proteins from different ornamentals was high (Fig. 4b). Furthermore, LUC results showed that miR172 repressed the expression of homologs of AP2L (Fig. 4c). Thus, the miR172-AP2L module appears to be conserved in regulating the double-flower phenotype in various ornamentals. The interaction between miR172 and AP2L in different ornamentals was validated by the dual luciferase reporter assay.
The 49-bp variation of PmAP2L affects its binding ability to floral organ identity genes
To determine the regulatory function of PmAP2L in the flower development, DAP-seq was used. A total of 7093 PmAP2L-D binding sites were identified, corresponding to 5760 genes (Dataset S3, see online supplementary material). Among them, 35.49% (2517 peaks), 20.68% (1467 peaks), 20.47% (1425 peaks), 12.83% (910 peaks), and 10.53% (747 peaks) were located in intronic, distal intergenic, promoter, exon, and downstream regions, respectively (Fig. 5a; Dataset S3, see online supplementary material). Through MEME-CHIP analysis of the binging peaks, the most significantly enriched motif sequence distribution was TTTT(G/T)TTTTT(G/T)TTT(A/T)T(G/T)(A/T)TTTTTTT,occupying approximately 63.26% of the peaks (Fig. 5b). GO enrichment analysis of these potential PmAP2L target genes showed that they were mainly enriched in DNA-binding transcription factor activity, regulation of transcription, DNA-templated, and sequence-specific DNA binding, while the auxin-activated signaling pathway had the lowest P value (Fig. 5c). KEGG analysis annotated the largest number of genes in plant hormone signal transduction pathway (Fig. 5d). Among these potential target genes, many genes related to flower development in the ABCDE model were found, such as class A gene PmTOE3, class C gene PmAG, class E gene PmSEP2/3, as well as flowering-related genes PmAGL24 and PmFUL1 (Fig. 5e; Dataset S3, see online supplementary material).
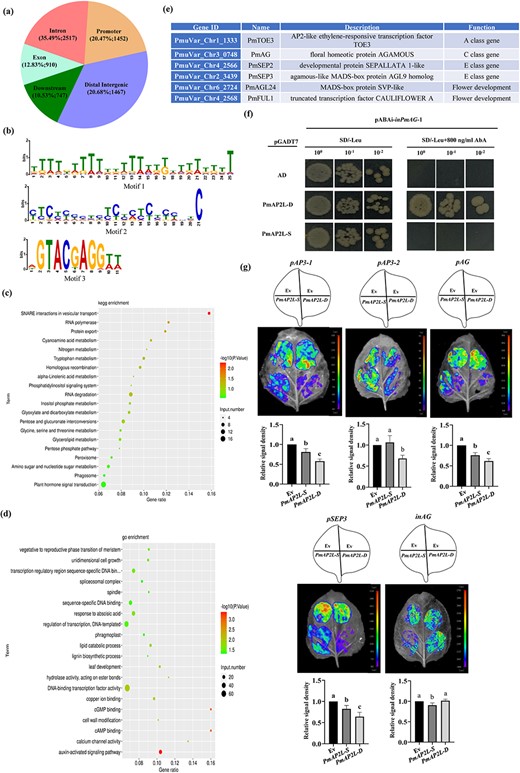
A 49-bp variation of PmAP2L affects its binding ability to floral organ identity genes. (a) Distribution of PmAP2L-D binding regions in Prunus mume genome based on DAP-seq analysis. (b) Conserved motifs of PmAP2L-D identified from the DAP-seq data. (c) KEGG enrichment analysis of all putative target genes regulated by PmAP2L. (d) GO enrichment analysis of all putative target genes regulated by PmAP2L. (e) PmAP2L may directly regulate genes related to flower development. (f) Y1H assay showing that the PmAP2L-D can bind directly to the second intron of PmAG, while PmAP2L-S cannot bind. (g) Effect of PmAP2L-D/S on the activity of the PmAP3–1, PmAP3–2, PmAG, and PmSEP3 promoters and the second intron of PmAG by dual-luciferase assay. The upper panels represent the mixed injection of vectors, the middle panels show representative leaf images, and the lower columns represent the statistical analyses of relative luminescence intensities. The relative luminescence intensities were calculated with the value of EV combination as 1, respectively. Data are represented as the mean ± SD of five biological replicates (n = 5). Different letters above the bars indicate a significant difference (P < 0.05, one-way ANOVA).
To confirm whether PmAP2L can regulate ABCDE model genes and to explore whether the 49-bp variation in AP2L affects its regulatory efficiency on ABCDE model genes, we performed yeast one-hybrid (Y1H) and luciferase complementation assay. First, through Y1H assays, it was found that except for the second intron of PmAG, other genes’ promoters were self-activated and could not be further tested. Y1H results showed that yeast cells containing either pGADT7-PmAP2L-D/S or pGADT7-empty grew well on non-selective medium SD/−Leu. Only the bait yeast cells co-transformed with pGADT7-PmAP2L-D survived on selective medium SD/−Leu/ + 800 ng/mL AbA (Fig. 5f). This indicated that the PmAP2L-D might bind directly to second intron of PmAG, while PmAP2L-S might not bind.
To detect if the PmAP2L-D and PmAP2L-S can inhibit the expression of ABCDE model genes in plants, we co-expressed 35S::PmAP2L-D/S vectors (effectors) with pPmAP3–1::LUC, pPmAP3–2::LUC, pPmAG::LUC, pPmSEP3::LUC, and inPmAG::LUC (reporters) in dual-luciferase reporter assays. The activity of the PmAP3–1, PmAG, and PmSEP3 promoters fused with the LUC gene was significantly inhibited by PmAP2L. Here, PmAP2L-D inhibited the LUC activity stronger than PmAP2L-S (Fig. 5g). Overexpression of PmAP2L-S had no significant effect on the LUC activity driven by the PmAP3–2 promoter compared to empty vector, while LUC activity was significantly depressed by the expression of PmAP2L-D (Fig. 5g). Conversely, compared to using the empty vector, PmAP2L-D had no significant inhibitory effect on the LUC activity driven by the second intron of PmAG, while PmAP2L-S significantly inhibited the LUC activity of inPmAG (Fig. 5g). The effect of PmAP2L-D/S on the activities of the PmAP3–1, PmAP3–2, PmAG, and PmSEP3 promoters and the PmAG second intron was validated by the dual luciferase reporter assay.
PmAP2L interacts with the PmTPL and PmHDA6/19
Proteins harboring EAR motifs play a crucial role in diverse biological processes by suppressing target gene expression. Transcription factors containing the EAR motif were reported to interact with TOPLESS (TPL) corepressor. The PmAP2L contained two typical EAR motifs (LxLxL), EAR1 and EAR2, located in the N-terminal and middle regions, respectively (Fig. 6a). We identified the mei TPL/TPR protein by phylogenetic analysis and named as PmTPL (PmVar_chr4_0936), which was close to AtTPL from the Arabidopsis (Fig. 6b). In order to explore whether PmAP2L-D/S functions as transcriptional repressors, the EAR motifs in PmAP2L-D/S were mutated and named as PmAP2L-D-mEAR1/2/3 and PmAP2L-S-mEAR1/2/3, respectively (Fig. 6c). Y2H assays between PmAP2L-D/S and PmTPL were performed and the results showed that PmA2PL-D/D-mEAR1 and PmA2PL-S/S-mEAR1 interacted with PmTPL, whereas mutation of the EAR2 motif in PmAP2L-D/S failed to do so (Fig. 6d). This suggested that the EAR2 motif rather than EAR1 was crucial for the interaction between PmAP2L-S/D and PmTPL. Luciferase complementation imaging (LCI) assays were performed and the results showed that the EAR3 (PmA2PL-D-mEAR3 and PmA2PL-S-mEAR3) abolished the interaction between PmAP2L-D/S and PmTPL (Fig. 6e). This indicated that the EAR was required for PmAP2L to interact with PmTPL.
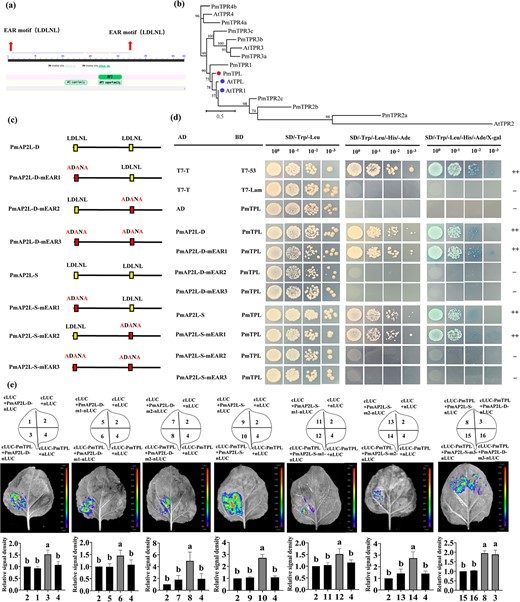
PmAP2L interacts with the PmTPL (a) Schematic representation of the PmAP2L protein structure. (b) Phylogenetic analysis of the TPL/TPR proteins. (c) Mutation of the EAR motif (mEAR) in the PmAP2L-D/S protein sequence. (d) Y2H assays showing that PmAP2L-D/S interacts with PmTPL through the EAR2 motif (e) LCI assays showing the interaction between PmTPL and PmAP2L through the EAR1 and EAR2 in tobacco leaves. The upper panels represent the mixed injection of vectors, the middle panels show representative leaf images, and the lower columns represent the statistical analyses of relative luminescence intensities. The relative luminescence intensities were calculated with the value of combinations 2, 2, 2, 2, 2, 2, 8 as 1, respectively. Data are represented as the mean ± SD of five biological replicates (n = 5). Different letters above the bars indicate a significant difference (P < 0.05, one-way ANOVA).
Histone deacetylases (HDAs) are additional components of TPL-dependent transcriptional repression complex. HDAs actively repress gene expression in many eukaryotes, including Arabidopsis HDA6 and HDA19 [23]. Y2H and LCI assays showed that PmAP2L-D/S and PmTPL interacted with PmHDA6/19, respectively (Fig. 7a–c; Fig. S12, see online supplementary material). The interaction between PmTPL and PmAP2L and between PmHDA6/19 and PmAP2L-D/S was validated by the firefly luciferase complementation imaging assay.
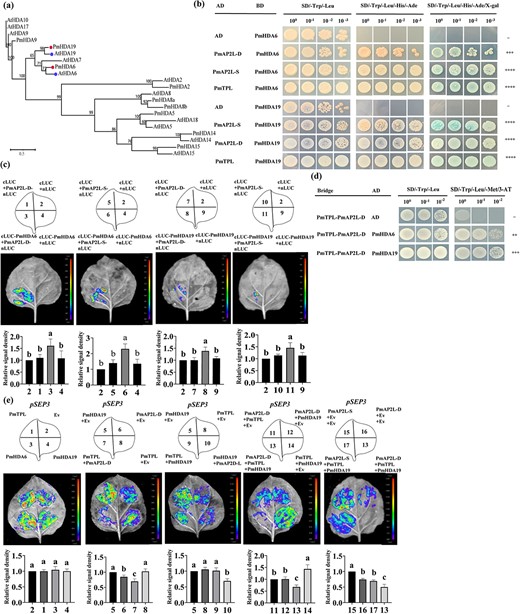
The 49-bp variation of PmAP2L affects PmAP2L-PmTPL-PmHDA19 binding ability to floral organ identity genes. (a) Phylogenetic analysis of PmHDA6/19 with HDAs from Arabidopsis thaliana. (b) PmAP2L interacts with the PmTPL and PmHDA6/19 by Y2H assay. (c) LCI assays showing the interaction between PmHDA6/19 and PmAP2L-D/S in tobacco leaves. The upper panels represent the mixed injection of vectors, the middle panels show representative leaf images, and the lower columns represent the statistical analyses of relative luminescence intensities. The relative luminescence intensities were calculated with the value of combination 2 as 1, respectively. Data are represented as the mean ± SD of five biological replicates (n = 5). Different letters above the bars indicate a significant difference (P < 0.05, one-way ANOVA). (d) Y3H was carried out to detect the interaction among PmAP2L, PmTPL, and PmHDA6/19. (e) Dual-luciferase assay showed that the activity of the PmSEP3 promoters fused with the LUC gene was significantly inhibited by PmAP2L-D-PmTPL-PmHDA19 complex compared with PmAP2L-S-PmTPL-PmHDA19 complex. The upper panels represent the mixed injection of vectors, the middle panels show representative leaf images, and the lower columns represent the statistical analyses of relative luminescence intensities. The relative luminescence intensities were calculated with the value of combinations 2, 5, 5, 11, 15 as 1, respectively. Data are represented as the mean ± SD of five biological replicates (n = 5). Data are represented as the mean ± SD of five biological replicates (n = 5). Different letters above the bars indicate a significant difference (P < 0.05, one-way ANOVA).
The 49-bp variation of PmAP2L affects PmAP2L-PmTPL-PmHDA19 binding affinity to floral organ identity genes
The interactions among PmAP2L-D/S, PmTPL, and PmHDA6/19 were verified by Y2H assays. Our prior analyses indicated that HDAs were involved in TPL-dependent transcriptional regulation. To determine whether the PmAP2L-TPL complex recruits HDAs to repress the expression of floral development identity genes, a Y3H assay was performed to study the interaction between the PmAP2L-TPL complex and PmHDA6 and PmHDA19. Yeast cells containing Bridge-PmTPL-PmAP2L-D and AD-PmHDA6/19 constructs grew well on SD/−Trp/−Leu and SD/−Trp/−Leu/−Met/3-AT medium (Fig. 7d), suggesting that PmAP2L-D, PmTPL, and PmHDA6/19 apparently formed a tripartite complex.
To examine whether PmAP2L recruits PmTPL and PmHDA19 to the promoter of PmAP2L target genes, a dual-luciferase reporter assay was performed. PmTPL, PmHDA6, and PmHDA19 barely influenced the activity of the LUC reporter. The LUC activity was significantly decreased only in the presence of PmAP2L-D, especially in the PmAP2L-PmTPL-PmHDA19 complex (Fig. 7e), suggesting that PmAP2L-D, PmTPL, and PmHDA19 might form a tripartite complex to repress the expression of floral development identity genes. In addition, compared with PmAP2L-S-PmTPL-PmHDA19 complex, our results showed that the activity of the PmSEP3 promoters fused with the LUC gene was significantly inhibited by PmAP2L-D-PmTPL-PmHDA19 complex (Fig. 7e). Therefore, we proposed that the 49 bp in PmAP2L affected the binding of the PmAP2L-PmTPL-PmHDA19 complex to floral organ identity genes, leading to double flower formation. The interaction of PmAP2L recruiting PmTPL and PmHDA19 to the PmSEP3 promoter was verified by the firefly luciferase complementation imaging assay.
Discussion
The double-flower trait holds significant ornamental value. Variations in TOE-type AP2 genes causing the double-flower phenotype have been reported in R. rugosa, petunia, carnation, and peach [15–17]. All the genes identified in these species belong to a single PETALOSE TOE-type subgroup, suggesting a robust conservation of the double flower phenotype throughout the course of evolution. However, the likelihood of differences between various plants and the appearance of new A class genes remains to be elucidated. The predominant focus in current research has been on identifying gene locations, with less emphasis on exploring the regulatory mechanisms governing these genes. Here, we used BSA analysis to identify a new gene, PmAP2L, which contributes to double-flower trait. It has a 49 bp variation that affects its binding with miR172. Meanwhile, we demonstrate that PmAP2L, an AP2 subfamily member containing EAR motifs, negatively regulates the transcription of multiple floral organ identity genes by forming a repressor complex with PmTPL and PmHDA6/19. We further elaborate that a 49-bp deletion in PmAP2L affects this regulatory process.
PmAP2L is a new gene that controls the double-flower trait in P. mume
The AP2 TFs play critical roles in plant developmental transitions [24–26]. In Arabidopsis, there are six members of the AP2-like gene family, including AP2, TARGET OF EAT1 (TOE1), TOE2, TOE3, SCHLAFMUTZE (SMZ), and SCHNARCHZAPFEN (SNZ) [25]. The AP2-type genes, AP2 and TOE3, are necessary for cell maintenance and specification of floral organ identity [24, 27, 28], whereas the TOE-type genes, TOE1, TOE2, SMZ, and SNZ, regulate flowering time [27, 29]. In this study, we found that PmuVar_Chr1_1333, Rosa_XP_024186592, Prupe.6G242400, Dianthus_Dca21030, and PhBOB belong to a single TOE-type, which is also named as PETALOSA [16]. The PETALOSA TOE-type genes are involved in the formation of double-flower in many plant species, such as peach, rose, carnation, and petunia [15–17, 24, 28]. Interestingly, PmAP2L-D/S, Rosa_XP_024157287, Peach_Prupe.6G091100, AtTOE1, and AtTOE2 also belong to the TOE-type, but are not PETALOSA TOE-type (Fig. 2a; Fig. S6 and Tables S13 and S14, see online supplementary material). Moreover, the two branches can be clearly distinguished based on the domain distribution, similarity, and microsynteny analysis (Fig. S6c–f). The genes in these branches have not been reported for their role in the regulation of flower development, especially the double flower formation [30]. We speculate that PmAP2L is a novel gene that determines the formation of double flowers in P. mume. The primary evidence comes from the transgenic tobacco experiments, wherein the transgenic lines show an increase in the number of stamens and the transformation of stamens to petals (Fig. 3b–e). Indirect evidence is that PmAP2L homologs are directly associated with the petal numbers in rose. Reducing the transcription level of RC5G0530900 in roses through VIGS significantly decreases the number of petals (Fig. 3h–j). Therefore, these results collectively support a new function of PmAP2L in floral organ identity.
A conserved role for the miR172-AP2L pathway on the double flower formation trait in various ornamentals
The AP2 TF family, including AP2, TOE1, TOE2, TOE3, SNZ, and SMZ, are regulated post-transcriptionally by miR172 [25, 26]. The miRNA172-mediated repression of AP2 plays a central role in floral induction and flowering [25, 27]. GUS staining and luciferase reporter assays confirmed that the presence of miR172 caused a significant reduction in GUS activities or luciferase activities driven by PmAP2L-D/S (Fig. 2e–f). These findings validate the regulation of PmAP2L by miR172. Notably, miR172 shears PmAP2L-S and PmAP2L-D differently, with miR172 repressing the single petal gene PmAP2L-S more significantly. Compared to wild type plants, there was no significant difference in the heterologous expression of PmAP2L-S in tobacco (Fig. 3b–e), while the heterologous expression of PmAP2L-D resulted in the transformation of stamens to petals. These results indicate that the 49 bp deletion-mediated miR172 target site loss in PmAP2L has a vital role in the double flower formation.
In Arabidopsis, AtTOE3 overexpression had no obvious phenotype. However, the overexpression of an miR172-resistant TOE3 gene resulted in an indeterminate floral structure [28]. In addition, mutation of the miR172 target site of tobacco PET genes resulted in double flowers [16]. These results further support the role of miR172 target site loss in PmAP2L in the double flower formation. Although the roles of miR172 or AP2-like have been reported in regulating flower development [27, 28], the PmAP2L clusters into a different subgroup from the currently reported AP2 TFs. However, the function of AP2-like in this branch has not been demonstrated in the double flower formation. This provides an important reference gene for the study of double flowers in other ornamentals.
The miR172 is a conserved microRNA, and the majority of miR172-AP2 studies have centered around flower development and floral transition [24, 25]. Previous studies reveal that AP2-like TFs are extremely important for floral organ development [24, 27]. As an upstream regulatory factor of AP2 transcription factors, miR172 can eliminate the influence of AP2-like TFs on flower development [27, 28]. Given the importance of miR172 and the conserved influence of AP2-like on flower development, it is crucial to investigate the conserved role of miR172-AP2 in floral organ development. It has been partially confirmed in seven ornamental plants (petunia, rose, peach, tree peony, water lily, rhododendron, and chrysanthemum) (Fig. 4a–c). In these ornamental plants, miR172 significantly inhibited the AP2-like TFs in vivo. Therefore, the miR172-AP2L pathway appears to contribute to the double-flower phenotype in various ornamentals.
PmAP2L interacts with PmTPL and recruits PmHDA proteins to negatively regulate the transcription of multiple floral organ identity genes
Despite the role of miR172-mediated repression of PmAP2L in the formation of double flower, it is important to know whether PmAP2L has the A-class gene function and whether the 49-bp variants affect its function. In Arabidopsis, TOE1 directly suppresses FT expression and flowering by binding to the promoter of FT and inhibiting the CO transcriptional activation activity [31]. The TOE1 homologs, ZmRAP2.7 in maize and AP2L1 in wheat, are also known as suppressors of flowering [32, 33]. Unlike them, the TOE1 homologs, PmAP2L in P. mume and RC5G0530900 in rose, has negligible impact on the timing of flowering. Notably, overexpression of PmAP2L-D in tobacco produces flowers with similar morphology to those of 35S::AP2m1 lines in Arabidopsis (Fig. 3c–e), indicating that PmAP2L also functions as an A-class gene [27]. Consistent with this, AG and SEP3 homologs, that are repressed by AP2 in Arabidopsis, are up-regulated in single-flower P. mume and wild-type tobacco (Fig. 2b; Fig. S9, see online supplementary material). Contrarily, these homologs are down-regulated in double-flower P. mume and in tobacco overexpressing the PmAP2L gene [23, 24]. In addition, DAP-seq also identified multiple genes involving flower development (Fig. 5e). These results suggest that PmAP2L and AtAP2 may specify floral organ identity through similar mechanisms.
Since the overexpression of PmAP2L-D was more effective in double flower formation compared to PmAP2L-S, we hypothesized that there might be some differences in the A-class gene functions between PmAP2L-D and PmAP2L-S. Here, we found that the 49-bp variance of PmAP2L affected its binding ability to floral organ identity genes and it negatively regulated the expression of PmAP3, PmAG, and PmSEP3 (Fig. 5g). PmAP2L-D was more significant in inhibiting flower development-related genes than PmAP2L-S. Although AP2-like gene has been reported to potentially affect AG expression and plays a role in double flower formation in some species, such as carnation, peach, and rose [15–17, 34], these studies are mostly speculative and have no direct evidence. In our study, PmAP2L-S became weaker in its ability to bind to the promoter and second intron of PmAG, whereas PmAP2L showed an enhanced ability to bind to the promoter and second intron of PmAG, and inhibited the expression of PmAG more significantly (Figs 2b and 5f and g). These results imply that the 49 bp variation in PmAP2L leads to differences in the regulation of AG gene, acting as a direct cause of double flower formation in P. mume.
The AP2 can act both as a transcriptional activator and a direct repressor [24]. We find that PmAP2L contains two conserved EAR motifs known to repress the transcription of downstream genes [23, 30, 35]. PmAP2L-D can inhibit the expression of floral organ identity genes, PmAP3–1, PmAP3–2, PmAG, and PmSEP3 by binding to their promoters. This repressor activity is gradually enhanced by the upregulation of the PmAP2L after the start of flower development in double flower, causing the continuous downregulation of genes such as AG and SEP3 in the second and third rounds of flower organ development (Figs 2b and 5f and g), which favors double flower formation in P. mume. The double flower formation in ornamental plants like P. mume is genetically programmed and involves complex interactions between multiple plant developmental factors. Whilst the specific underlying mechanisms are not yet clear, it is generally believed that both inhibitors and activators are at work [23, 24].
The AP2 containing the EAR motif in Arabidopsis has recently been proven to negatively regulate floral homeotic genes by recruiting TPL and HDA19 [23]. Here, we demonstrate that PmAP2L in P. mume interacts with the co-repressor PmTPL through the EAR motif to recruit the histone deacetylase PmHDA19 to form a complex that negatively regulates floral organ identity genes. Interestingly, compared with PmAP2L-S, PmAP2L-D has a more significant inhibitory effect on the expression of floral organ identity genes when forming a complex with PmTPL and PmHDA19. In summary, these data support a model where PmAP2L regulates the formation of double flower in P. mume (Fig. 8). PmAP2L is negatively regulated by miR172. In double flower, a 49 bp deletion in PmAP2L induces a decrease in the ability of miR172 to repress its expression, resulting in an increase in PmAP2L-D expression compared to PmAP2L-S. PmAP2L interacts with PmTPL and PmHDA19 to form a complex that inhibits the transcription of flower development-related genes. When PmAP2L is not mutated, PmAP2L-S becomes too weak to repress the promoters of PmAP3–1, PmAP3–2, PmAG, and PmSEP3. This results in a significant increase in genes expression and the single-flower phenotype in P. mume. A 49 bp deletion in PmAP2L changes its function and it binds to the second intron of PmAG, resulting in enhanced repression of PmAG expression. At the same time, its inhibitory effect on PmAP3–1, PmAP3–2, PmAG, and PmSEP3 promoters becomes more intense, resulting in the double flower trait in P. mume. Therefore, our work reveals that the 49 bp variation in PmAP2L leads to differences in the regulation of flower development-related genes, including PmAP2L-D and PmAP2L-S. The discovery of PmAP2L provides valuable insights into complex regulatory pathways underlying the floral diversity of ornamental plants and sets new directions for future molecular breeding of P. mume.
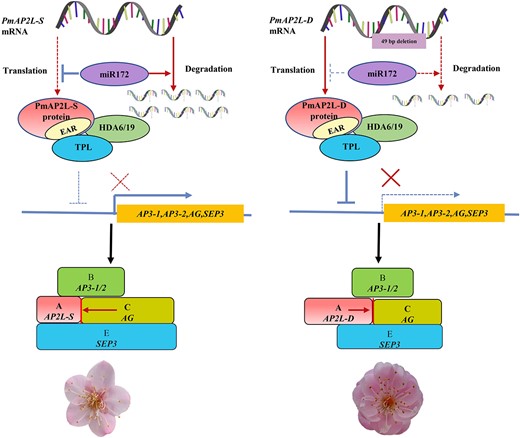
Proposed model for PmAP2L function in the formation of double flower in Prunus mume. In the absence of PmAP2L mutations, PmAP2L-S is more significantly regulated by miR172 than PmAP2L-D. The protein encoded by PmAP2L interacts with PmTPL and PmHDA19 to form a complex, which has a weaker inhibitory effect on flower development-related genes, resulting in a significant increase in genes expression and the single-flower phenotype in P. mume. When PmAP2L has the 49 bp variant, the regulation of PmAP2L-D by miR172 decreases. PmAP2L-D is highly expressed, and it forms an inhibitory complex with PmTPL and PmHDA19, which has a more significant inhibitory effect on flower development-related genes in the second and third floral organs, resulting in the appearance of double flower in P. mume.
Materials and methods
Plant materials
An F1 population of 162 individuals generated from the female parent 'Mi Danlv' and the pollen donor 'Baixu Zhusha' was used to identify the genetic locus controlling the double-flower formation in P. mume. The plants were cultivated in Jiangle State-owned Forest Farm, Fujian Province, China. The genomic DNA from young leaves of 64 double-flowered individuals and 41 single-flowered individuals was used for BSA-seq. Nicotiana benthamiana, Nicotiana tabacum, R. chinensis ‘Old Blush’ were grown as described previously [36, 37].
Scanning electron microscopy
Floral tissues were collected every 14 d from mid-August to mid-November 2022 and stored in 2.5% glutaraldehyde solution. The flower buds were fixed at 4°C for 24 h or longer, dehydrated for 20 min through a series of alcohol and isoamyl acetate, dried at critical-point, mounted on the scanning electron microscopy (SEM) platform, and coated with gold. Finally, the buds were observed by a S-3400N scanning electron microscopy (Hitachi, Tokyo, Japan).
Phenotyping and data analysis
The double flower phenotypes of F1 hybrids were ascertained during the flowering periods from January to February of 2021 and 2022, respectively. The double flower trait was assessed by the average petal number of five or more flowers in each plant. When the number of petals is 5–7, it is considered a single flower, and when the number is 15 or more, it is considered a double flower. The descriptive statistical analysis and frequency distribution histogram were performed using Microsoft Office EXCEL 2019 software. Considering that P. mume is a woody flower with a highly heterozygous background, and that there is obvious segregation of traits such as petal number and flower color in the F1 hybrids, the phenotypic data of the F1 hybrids were considered as pseudo F2 hybrids for genetic analysis. The SEA package (https://cran.r-project.org/web/packages/SEA/index.html) in R (https://www.r-project.org/) was used to analyse the major gene plus polygene mixed inheritance model in this study. Specific genetic model analysis was described with reference to the description of SEA software.
BSA sequencing and analysis
Genomic DNA from young leaves was isolated using DNAsecure Plant Kit (Tiangen Biotech, Beijing, China). The extracted DNA samples were tested for quantity and quality using a Nanodrop 2000 Spectrophotometer (Thermo Scientific, USA). F1 individuals showing double or single flower were selected. The BSA extreme pools were divided based on the single-petaled pool with five petals and the double-petaled pool with 15 or more petals. A total of 64 plants were selected for the double flower pool, and 41 plants were selected for the single flower pool. An equal amount of DNA sample was collected from F1 population by phenotype. The pooled DNAs were sequenced on an Illumina Hiseq X10 PE150 (Genedenovo Biotechnology Co., Ltd, Guangzhou, China).
The raw reads were first filtered to obtain high-quality clean reads. The clean reads were aligned to the P. mume reference genome using Burrows-Wheeler Aligner [36, 38], and the results were sorted and tagged with duplicated sequences using Picard (Http://sourceforge.net/projects/picard/). Coverage statistics were performed using bedtools (v2.27.1) [38]. The processed alignment files were used for variant calling, which was performed on each sample using GATK’s Variant Filtration with appropriate parameter settings. SNPs and INDELs were annotated by ANNOVAR software [39]. The Δ(SNP/InDel-index) and the Euclidean distance calculation were used for mapping candidate regions associated with double flower phenotype [40, 41].
Cloning of PmAP2L, genotyping, and phylogenetic analyses
The coding sequence (CDS) of PmAP2L was cloned from the buds of the single flower (named PmAP2L-S) and double flower (named PmAP2L-D) varieties of F1 population (primers used are listed in Table S15, see online supplementary material). Double-flowered varieties of P. mume included ‘Longyou’ (LY) and ‘Fentai Chuizhi’ (FTCZ), and the single-flowered variety included ‘Liuban’ (LB). The fragments of the 49 bp variant in PmAP2L were cloned from the double flower pool and single flower pool of the F1 population, as well as from randomly selected pools of 10 double and 10 single flower varieties. A CAPS marker was developed based on the 49-bp variant in PmAP2L, and genotyping was performed in the double and single flower pools of the F1 population. We applied MUSCLE to align protein sequences under default parameters. The phylogenetic tree was constructed by using the Maximum Likelihood method in iqtree tool, and 2000 bootstrap replicates were set to assess the reliability of the phylogenetic tree.
RNA extraction and qRT-PCR
Total RNA was obtained from each sample using the RNAprep Pure Plant Plus Kit (DP441, TIANGEN), and first-strand cDNAs were synthesized from 1 μg of total RNA using the PrimeScript™ RT reagent Kit with gDNA Eraser (RR047, TaKaRa, Bejing, China). Gene-specific primers used in qRT-PCR were designed by the NCBI primer tool (Table S15, see online supplementary material). The qRT-PCR was performed as described previously for the reaction system and conditions using the SYBR Premix Ex Taq II kit (RR820, TaKaRa) on a PikoReal real-time PCR system (Thermo Fisher Scientific, Waltham, MA, USA) [36, 42]. The relative expression levels of genes were calculated using the 2 – ΔΔCt formula with PmPP2A, NtActin, and RcTCTP as the internal reference genes in P. mume, tobacco and rose, respectively.
GUS staining analysis
35S::PmAP2L-D-GUS and 35S::PmAP2L-S-GUS constructs were generated by inserting the CDS of PmAP2L-D/S into the pCAMBIA1304-GUS vector through Nco I restriction site (Table S15, see online supplementary material). P. mume microR172a precursor gene (Pmu-pre-miR172a) was inserted into the pCAMBIA1304-GUS vector according to a previous sequence synthesis [43]. GUS staining was executed as previously specified [37].
Dual luciferase reporter assay
The precursor sequences of Pmu-miR172a and other ornamental plant miR172 and STTM172 were recombined into pGreenII0029 62-SK, whereas the miR172 target site fragments of PmAP2L-D/S and homologous AP2Ls were inserted into the pGreenII 0800-35S-LUC. STTM172 was designed and synthesized according to the mature sequence of miR172 [44]. For the transcriptional activity assay, the CDS of PmAP2L-D/S, PmTPL, and PmHDA6/19 were PCR amplified from cDNA of P. mume and cloned into the pGreenII 0029 62-SK vector to construct effectors, and the promoter sequences of PmAP3–1, PmAP3–2, PmAG, and PmSEP3. The second intron sequence of PmAG was amplified from gDNA and inserted into the pGreenII 0800-LUC vector to construct the reporter (Table S15, see online supplementary material). The LUC reporter assay was executed as previously specified [31].
Subcellular localization
35S::PmAP2L-D-GFP and 35S::PmAP2L-S-GFP constructs were generated by inserting the CDS of PmAP2L-D/S into the pCAMBIA1300super vector through Kpn I restriction site (Table S15, see online supplementary material). Subcellular localization was performed according to a method described previously [37].
Tobacco transformation
35S::PmAP2L-D and 35S::PmAP2L-S constructs were generated by inserting the CDS of PmAP2L-D/S into the pCAMBIA1304 vector through Nco I and Bst EII restriction sites (Table S13, see online supplementary material). The constructed vectors were transformed through Agrobacterium tumefaciens-mediated transformation in tobacco leaves and positive lines were confirmed by qRT-PCR as described previously [42].
Virus-induced gene silencing assay of rose
The CDS of RC5G0530900 was PCR amplified from cDNA of R. chinensis ‘Old blush’. A gene-specific fragment of RC5G0530900 (234 bp in length) was inserted into the Kpn I-Sac I site of pTRV2 vector to produce the pTRV2-RC5G0530900 construct (Table S15, see online supplementary material). The rose VIGS experiments were executed as previously specified [45]. We collected newly emerged young leaves after silencing by VIGS for the qRT-PCR analysis.
Transient gene expression in P. mume flower buds
Transiently overexpressing PmAP2L-D/S and PmMIR172a was performed using the 35S::PmAP2L-D/S and 35S::Pmu-pre-172a constructs that were used in tobacco transformation. For VIGS, a gene-specific fragment of PmAP2L (234 bp in length) and STTM172 was inserted into the Kpn I-Sac I site of pTRV2 vector to produce the pTRV2-PmAP2L and pTRV2-STTM172 constructs, respectively (Table S15, see online supplementary material). Transient transformation experiments were executed as previously specified with some modifications.
The constructs were then transformed into A. tumefaciens GV3101 cells and cultured in Luria-Bertani medium with 50 mg/L kanamycin and 25 mg/L rifampicin. The cells were resuspended in buffer (10 mM MgCl2, 10 mM 2-(N-Morpholino)-ethanesulfonic acid, pH = 5.8, 150 μM acetosyringone) until OD600 = 0.8, and then placed at room temperature in the dark for 4 h. 'Jiangmei' shoots were collected from natural conditions in mid-November 2022 and then cut into single-node shoots at room temperature conditions. The junction of the bud and branch were pierced with syringe needles. Subsequently, the shoots were soaked in the infiltration buffer and exposed to vacuum for 15 min. The infiltrated shoots were washed with distilled water and placed on water-soaked floral foam. The floral foams were placed in the dark for 24 h and then incubated at 25°C/23°C (light/dark). After 5 days of infection, the flower buds were collected for the qRT-PCR analysis.
DAP-seq
The genomic DNA was extracted from the leaves of P. mume using the CTAB method to prepare the DNA library. The CDS of PmAP2L-D was inserted into the HaloTag expression vector to generate recombinants. A mixture of the Magne HaloTag Beads and Halo-PmAP2L-D was incubated with the DNA library. The DNA fragment bound to the PmAP2L was eluted from the beads and sequenced on the Illumina NovaSeq 6000 platform. The clean reads were aligned to the P. mume reference genome using BWA-MEM [46]. The sequenced data were identified for peaks using MACS [47]. We employed MEME-ChIP in order to identify the core motifs [48]. DAP-seq was executed as previously specified [49, 50].
Yeast one-hybrid assay
The CDS of PmAP2L-D/S was inserted into the Eco R I site of the pGADT7 as prey. The promoter sequences of PmAP3–1, PmAP3–2, PmAG, and PmSEP3, the second intron sequence of PmAG, and its partial sequences (with AP2 binding sites predicted by PlantPAN 3.0), containing a triplication of AP2 binding site variants plus three nucleotides of the flanking sequence on both sides from pPmAG and inPmAG, were ligated in the Sac I-Kpn I sites of the pAbAi vector as baits (Table S15, see online supplementary material). A total of 19 recombinant plasmids were transformed into the Y1H Gold strain after being linearized by Bst BI, and their auto-activation was detected on the SD/-Ura/AbA medium. Subsequently, the Y1HGold strain without auto-activation was re-transformed with the pGADT7-PmAP2L-D/S vector or empty vector. The transformant cells were then spotted on the SD/−Leu + 800 ng/mL AbA medium and cultured for 72 h to identify DNA-protein interactions.
Yeast two-hybrid assay
The CDSs of PmTPL, PmAP2L-D/S, and PmHDA6/19 were inserted into the Eco R I site of the pGBKT7 as baits. The CDSs of PmAP2L-D, PmAP2L-D-mEAR1 (mutation of the first EAR motif), PmAP2L-D-mEAR2 (mutation of the second EAR motif), PmAP2L-D-mEAR3 (mutation of all EAR motif), PmAP2L-S, PmAP2L-S-mEAR1/2/3, and PmTPL were inserted into the Eco R I site of the pGADT7 as preys (Table S15, see online supplementary material). Subsequently, the various preys and baits were co-transformed into the yeast strain Y2Hgold. The transformants were then cultured on different media (SD/−Trp/−Leu; SD/−Trp/−Leu/-His/−Ade + 3AT; SD/−Trp/−Leu/-His/−Ade + 3AT + X-α-Gal) and grown for 72 h to identify the interaction between two proteins.
Yeast three-hybrid assay
The CDS of PmTPL was inserted into the Not I-Bgl II sites of the pBridge vector to generate the pBridge-PmTPL plasmid (Table S15, see online supplementary material). Subsequently, the CDSs of PmAP2L-D/S and PmHDA6/19 were cloned into the Sal I site of the pBridge-PmTPL plasmid to produce the pBridge-PmTPL-PmAP2L-D/S and pBridge-PmTPL-PmHDA6/19 vector as baits (Table S15, see online supplementary material). The CDSs of PmAP2L-D/S and PmHDA6/19 were inserted into the Eco R I site of the pGADT7 as preys (Table S15, see online supplementary material). The Yeast three-hybrid assay was executed as previously specified [51].
Firefly luciferase complementation imaging assay
The CDSs of PmAP2L-D, PmAP2L-D-mEAR1/2/3, PmAP2L-S, PmAP2L-S-mEAR1/2/3 were cloned into the Kpn I-Sal I sites of the pCAMBIA1300-nLUC (Table S15, see online supplementary material). The CDSs of PmTPL and PmHDA6/19 were cloned into the Kpn I-Sal I sites of the pCAMBIA1300-cLUC (Table S15, see online supplementary material). Luciferase complementation imaging assay was executed as previously specified [31].
Acknowledgements
The research was supported by the Fundamental Research Funds for the Central Universities (No. QNTD202306), National Natural Science Foundation of China (No. 32371947, 32071816), Beijing High-Precision Discipline Project, Discipline of Ecological Environment of Urban and Rural Human Settlements and the Special Fund for Beijing Common Construction Project.
Author contributions
W.L., Q.Z., and T.Z. planned and designed the research. T.Z. and W.L. conducted experiments and material collection. W.L. and T.Z. analysed data. W.L., L.Q., X.G., L.P., X.Y., L.L., J.W., and T.C. conducted fieldwork and material maintenance. S.A. modified the language. W.L. and T.Z. wrote the manuscript. T.Z. and Q.Z. revised the manuscript and finalized the manuscript. All authors have read and approved the final manuscript.
Data availability
The raw sequence data of BSA-seq is available in the National Genomics Data Center (NGDC) with the accession number PRJCA017066.The DAP-seq data have been deposited to the National Genomics Data Center (NGDC) with the accession number PRJCA017094.
Conflict of interest statement
The authors declare no conflict of interest.
Supplementary data
Supplementary data is available at Horticulture Research online.