-
PDF
- Split View
-
Views
-
Cite
Cite
D. A. Clark, M. A. Lackie, Palaeomagnetism of the Early Permian Mount Leyshon Intrusive Complex and Tuckers Igneous Complex, North Queensland, Australia, Geophysical Journal International, Volume 153, Issue 3, June 2003, Pages 523–547, https://doi.org/10.1046/j.1365-246X.2003.01907.x
- Share Icon Share
Summary
This study provides reliable, precisely defined and well-dated Early Permian (286 ± 6 Ma) palaeomagnetic poles for Australia from the Mount Leyshon Intrusive Complex (MLIC) and the Tuckers Igneous Complex (TIC). Both complexes are associated with prominent negative magnetic anomalies, indicating the presence of rocks carrying stable remanence of reverse polarity, with a Koenigsberger ratio greater than unity. The characteristic remanence carried by the intrusive phases and by locally remagnetized, contact-metamorphosed host rocks is always of reverse polarity, consistent with acquisition during the Permo-Carboniferous (Kiaman) Reverse Superchron. The corresponding palaeopoles confirm that Australia occupied high latitudes in the Early Permian. The pole positions are: MLIC: lat. = 43.2 °S, long. = 137.3 °E; dp = 6.0°, dm = 6.4°; Q= 6; TIC: lat. = 47.5 °S, long. = 143.0 °E, dp = 6.0°, dm = 6.6°; Q= 6. Permian palaeomagnetic overprinting is detectable at considerable distances from the MLIC (2–3 km), well beyond the zone of visible alteration. The primary nature of the Early Permian palaeomagnetic signature is established by full baked contact/aureole tests at both localities.
Other new data from Australia are consistent with the poles reported here. Comparison of the Australian, African and South American Apparent Polar Wander Paths (APWP) suggests that mean Permian and Triassic poles from West Gondwana, particularly from South America, are biased by remagnetization in the Jurassic—Cretaceous and that the Late Palaeozoic—Mesozoic APWP for Gondwana is best defined by Australian data. The Australian APWP exhibits substantial movement through the Mesozoic. Provided only that the time-averaged palaeofield was zonal, the Early Triassic palaeomagnetic data from Australia provide an important palaeogeographic constraint that the south geographic pole was within, or very close to, SE Australia around 240 Ma.
The new Early Permian poles are apparently more consistent with Pangaea B-type reconstructions of Gondwana and Laurussia than with the Pangaea A2 configuration. This may be partly an artefact of reconstruction problems within Gondwana, as systematic differences between approximately coeval, apparently reliable, Permo-Carboniferous poles from Africa, South America and Australia are evident in standard Gondwana reconstructions. These discordances require a tighter fit of the southern continents, suggesting that some attenuation of continental margins, not accounted for in the reconstructions, has occurred during breakup of Gondwana, or that the fit between East and West Gondwana needs to be substantially modified. If stretching of continental margins during breakup of supercontinents is a general phenomenon, it may help to ameliorate, but not solve, the long-standing controversy regarding Pangaea reconstructions.
Although alternative Pangaea reconstructions, such as Pangaea B, may reconcile poles from Laurussia with Australian poles in the Late Carboniferous—Early Permian, no plausible reconstruction can bring the Early Triassic poles into agreement. This suggests that persistent departures from a pure dipole field may have been present in the Early Triassic. Lesser, but still significant, non-dipole effects may also have been present during the Late Carboniferous and Permian, and may help resolve the Pangaea A versus B controversy, without requiring substantial attenuation of continental margins or intracontinental deformation.
We suggest that the most parsimonious interpretation of the palaeomagnetic and geological information is that Laurussia and Gondwana remained in a Pangaea A2-type configuration through the Permian and Triassic. Discordance between the APWPs for these two supercontinents is attributable mainly to persistent non-dipole components of the geomagnetic field, which were most important in the Early Triassic.
Introduction
Palaeogeographic reconstructions for pre-Mesozoic times rely heavily on well-defined apparent polar wander paths from each of the continental blocks involved in the reconstructions. The tightness of the fit of the southern continents into the supercontinent Gondwana during the Palaeozoic, and the degree of subsequent intraplate deformation in Africa, can only be tested with precise, well-dated, essentially coeval, palaeomagnetic poles from its constituent continents. Similarly, differing hypotheses regarding the relative configuration of Gondwana and Laurussia within Pangaea in the Late Palaeozoic require a comparison of well-defined coeval poles from the two constituent supercontinents. Unfortunately, high-quality palaeomagnetic data for the Late Palaeozoic have been scarce for the Gondwana continents (Van der Voo 1993; McElhinny & McFadden 2000).
Over the last decade, a number of relatively high-quality palaeomagnetic poles for Carboniferous and Permian rocks of Africa have been reported (Henry 1992; Nyblade 1993; Derder 1994). However, data of comparable reliability from other Gondwana continents have been lacking until recently. This paper reports two new, precise Early Permian poles from Australia, with well-dated ages of magnetization, that can help to constrain palaeogeographic reconstructions of Gondwana and Pangaea in the Late Palaeozoic.
Regional Geology
The Ravenswood Batholith of northeast Queensland is the major element of the Lolworth—Ravenswood Province (Fig. 1). As defined by Hutton (1993), the Ravenswood Batholith comprises granitoids and gabbroic rocks that range in age from the Early Ordovician to the Middle Devonian. These authors assign a later episode of magmatism, in the Middle Carboniferous to the Late Permian, to the North Queensland Volcanic and Plutonic Province of Day (1983). For this reason the Permo-Carboniferous igneous rocks that are the subject of this study have been termed ‘post-batholithic’.
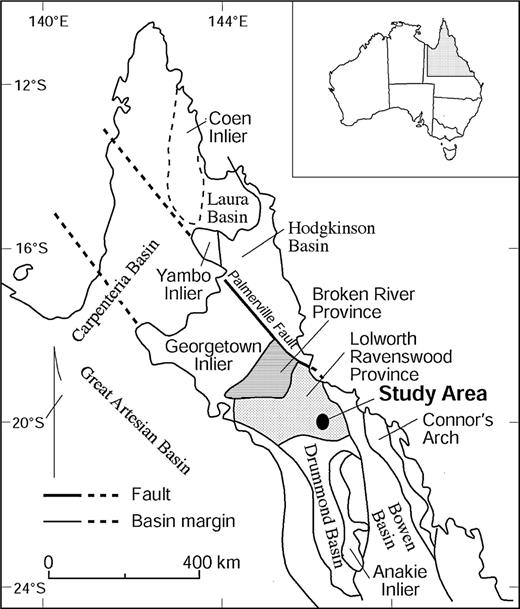
Regional geological setting of the Lolworth—Ravenswood Province (after Hutton 1994).
In the south, the granitoids intrude the Late Cambrian to Early Ordovician 70 Mile Range Group, which comprises the Puddler Creek Formation, Mount Windsor Volcanics, Trooper Creek Formation and the Rollston Range Formation. In the central and northern parts of the batholith the granitoids intrude metamorphic rocks (Charters Towers Metamorphics, Argentine Metamorphics and Kirk River Beds), which occur as isolated large rafts.
Carboniferous to Permian igneous rocks make up about 6 per cent of the batholith, predominantly in the east and southeast. This study concentrates on a prominent ENE-trending corridor of Permo-Carboniferous igneous rocks (Fig. 2), the Leyshon—Tuckers corridor (or Mount Leyshon (MTL) Corridor), which is defined by an alignment of several Permo-Carboniferous intrusions and volcanic complexes (Morrison 1988), including the Mount Leyshon Intrusive Complex (MLIC), the Fenian Diorite, Mathews Pinnacle, 70 Mile Range, the Cornishman Complex and the Tuckers Igneous Complex (TIC). The northern boundary of this corridor coincides with the linear northern margin of the TIC. This feature is visible in regional aeromagnetic images, but is less prominent than a slightly more easterly trending lineament, the Boori lineament (Tenison Woods & Rienks 1992), which passes through Mount Leyshon, intersecting the southern end of the TIC and the northern end of the Boori Complex, which is an Early Permian intrusive complex that is very similar in character to the coeval TIC. The geophysical expression of the Boori lineament is a zone of ENE-trending magnetic anomalies, particularly elongated zones of relative magnetic lows within magnetic granitoids. These magnetic lows are generally attributable to reversed remanent magnetization. Reverse polarity is characteristic of stable remanence acquired during the Permo-Carboniferous (Kiaman) Reverse Superchron, which lasted from ∼315 to ∼260 Ma (McElhinny & McFadden 2000). The Leyshon—Tuckers Corridor/Boori Lineament may represent an ancient basement structure that has localized emplacement of Permo-Carboniferous intrusions.
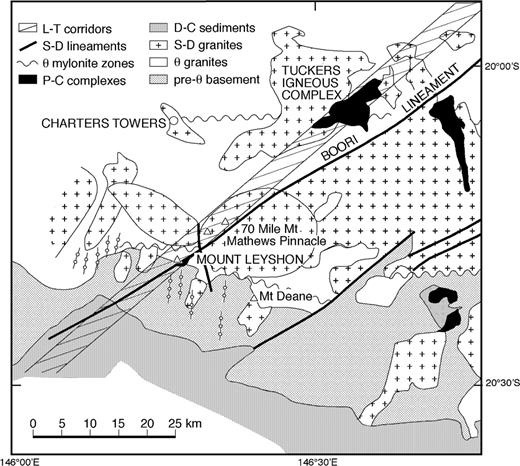
Geological context of the Leyshon—Tuckers Corridor (after Hutton 1994).
The Lolworth—Ravenswood Province has been tectonically stable, apart from shearing in local mylonite zones, since the Ordovician (Hutton 1993, 1994). The Siluro-Devonian intrusions are undeformed. Gravity data (Stockhill & Hutton 1991) indicate that the batholith, which is a sheet-like body with a N—S extent of 50 km and an E—W extent of 100 km, has a fairly consistent thickness of only 5–6 km. Furthermore, most of the batholithic plutons appear to be sheet-like, were emplaced at shallow crustal levels and are presently exposed near their roofs, with numerous large rafts of pre-batholithic rocks occurring as roof pendants (Tenison Woods & Rienks 1992). The roof contacts are flat-lying and occur at fairly constant elevation, so that the present exposure, with limited topographic relief, approximates the flat-lying batholith roof. These observations indicate negligible tilting of the batholith.
Geology of the Mount Leyshon Intrusive Complex
The Mount Leyshon mine within the MLIC has been one of the largest gold producers in Australia over the last decade. Mineralization is hosted in an Early Permian subvolcanic complex of intrusive breccias and porphyries. Recent summaries of the geology of the MLIC include those of Morrison (1988), Wormald (1991), Wormald (1993) and Orr (1994, 1995). Sexton (1995) also summarize the geology and analyse the magnetic signature of the complex. Fig. 3 shows a plan view of the geology of the MLIC, with sample locations.
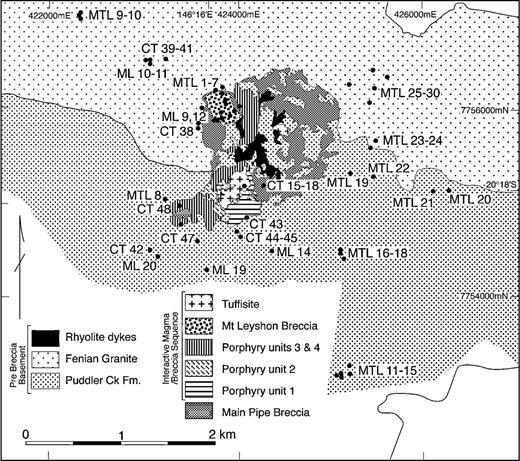
Geology of the Mount Leyshon Intrusive Complex (after Wormald 1991), with sampling localities indicated. ML, MTL and CT refer to samples from three different field trips.
The MLIC straddles the contact between the Ordovician Fenian Granite and the Late Cambrian Puddler Creek Formation. The Fenian Granite contains small amounts of primary low-Ti magnetite. In the Mount Leyshon area the Puddler Creek Formation consists of siltstones, sandstones and greywackes, metamorphosed to greenschist facies. Both the Fenian Granite and the Puddler Creek Formation are extensively intruded by dolerite dykes. Mount Leyshon lies within, and truncates, a NE-trending swarm of these dykes. The age of the dykes is only broadly constrained (mid-Silurian to Early Devonian). Clasts of Fenian Granite, Puddler Creek metasediments and dolerite dykes are abundant in the breccias of the MLIC.
The complex has undergone a complex history of magmatic, brecciation and hydrothermal events. Wormald (1991) and Wormald (1993) subdivided the complex into three main elements: ‘basement’, ‘Main Pipe Breccia’ and an ‘interactive breccia/magma sequence’. The earliest breccia event formed the Main Pipe Breccia, which is the most volumetrically significant unit within the MLIC. The Main Pipe Breccia is a predominantly matrix-supported breccia consisting of clasts and matrix derived from basement lithologies. Emplacement of rhyolitic early dykes commenced during formation of the Main Pipe Breccia and continued during formation of the slightly younger Mount Leyshon Breccia. Emplacement of the larger porphyry plugs was broadly contemporaneous with the formation of the Mount Leyshon Breccia. These intrusive and breccia phases have been affected to varying extents by a series of potassic, propylitic and phyllic alteration events. Weakly altered to unaltered trachytic late dykes, some with hornblende phenocrysts, intrude the other porphyries and both breccia phases.
K—Ar dating of igneous phases and alteration assemblages implies that the magmatic and hydrothermal evolution of the MLIC occurred over the interval 292 Ma, which is the age of the earliest intrusions, to 280 Ma, which is the age of an unaltered porphyritic basalt dyke that cuts all other intrusive and breccia phases (Blevin & Morrison 1997). Five K—Ar age determinations of hydrothermal muscovite from intervening phyllic alteration events give an average age of 283 ± 4 Ma (2σ). Thus the age of the MLIC is Early Permian.
The primary magnetic mineral in the intrusive phases of the MLIC is low-Ti magnetite, which occurs in amounts ranging from ∼1 per cent by volume in relatively mafic hornblende trachyte porphyries to less than 0.1 per cent in rhyolite porphyries (Lackie 1991; Clark 1996). The primary magnetic mineral in the dolerite dykes that intrude the host rocks of the MLIC is magnetite with ilmenite lamellae, produced by deuteric oxidation of early crystallizing titanomagnetite. These dykes are truncated by the MLIC, but are incorporated as clasts into the intrusive breccias of the MLIC.
Early potassic (secondary biotite—magnetite) alteration is extensively developed in basement metasediments and dolerite dykes adjacent to the MLIC and in the Main Pipe Breccia (Sexton 1995). The secondary magnetite is sufficiently fine-grained to carry intense, stable remanent magnetization with reverse polarity (Lackie 1991; Sexton 1995; Clark 1996). The complex is associated with a distinct negative magnetic anomaly, as is a nearby coeval intrusion, the Fenian Diorite, owing to the stable reversed magnetization. The palaeomagnetic sampling was carried out both in and around the complex (Fig. 3), allowing a full contact test of the age of magnetization. Where phyllic alteration locally overprints the early potassic alteration, primary and secondary magnetite are almost completely destroyed, being replaced by pyrite. The magnetic carriers in the weakly magnetic phyllically altered rocks are haematite with trace amounts of relict magnetite.
Geology of the Tuckers Igneous Complex
The Tuckers Igneous Complex (TIC) is a T-shaped feature in outcrop plan (Fig. 4), with a NE-axis of 13.5 km, and a SE-oriented axis, ∼5 km in length. A small outlier occurs SE of the main complex. Earlier mapping of the TIC has been discussed by Clarke (1971) and Hutton (1994). Detailed remapping of the TIC was carried out in 1994 (Beams 1994). The mapping and petrological data have established that the TIC is a nest of very hot, oxidized, melt-rich comagmatic intrusions, which evolved by fractional crystallization at depth and were then intruded as batches with compositions ranging from dry mafic diorite and gabbro, through quartz monzodiorite to relatively quartz-poor, more fluid-rich granodiorite. Minor aplites are also present. There is also evidence of some internal zoning within intrusions, owing to in situ differentiation. The early intrusions were so hot that they converted country rock granitoids at the contacts of the complex into anhydrous pyroxene hornfels. Fluids derived from these dehydration reactions, possibly mixed with magmatic fluids, moved further out into the aureole, forming a secondary amphibole and biotite hornfels zone. Secondary magnetite was produced in both the pyroxene and biotite hornfelses. The alteration in the biotite hornfels zone is similar to the early potassic alteration at Mount Leyshon.
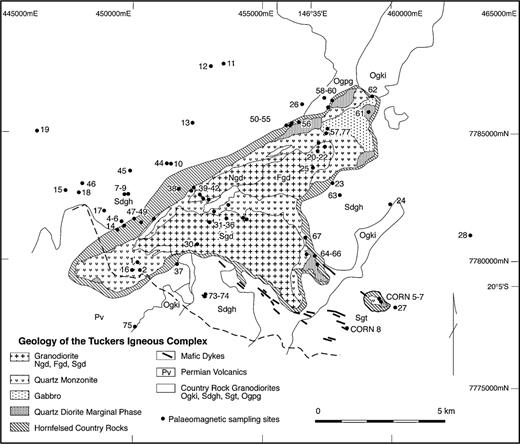
Geology of the Tuckers Igneous Complex. Ogki, Kirklea Granite; Sdgh, Heathfield West Tonalite; Sgt, Rishton Granodiorite; Ogpg, Ordovician Hornblende Porphyry; Ngd, Northern Granodiorite; Sgd, Southern Granodiorite; Fgd, Felsic Granodiorite (after Beams 1994). The distribution of palaeomagnetic sampling sites in and around the TIC is indicated.
The mafic units of the TIC (quartz diorite and gabbro) occupy the margins of the complex, suggesting that the initial intrusion had this composition, but was largely stoped out by the later intrusions. Inclusions of quartz diorite composition are common within the younger intrusions, supporting this hypothesis. The main quartz monzodiorite phase occurs as a relatively mafic rind around most of the complex and as a screen separating the northern (Ngd), felsic (Fgd) and southern (Sgd) granodiorite masses. The Ravenswood Batholith granitoids that make up the country rock are the Kirklea Granite (Ogki); an Ordovician hornblende porphyry (Ogpg); the Rishton Granodiorite (Sgt) and the Heathfield West Tonalite (Sdgh).
Geochemical analyses have established that the intrusive phases of the TIC are comagmatic with each other and with the andesitic volcanics (Pv) that abut the SW end of the complex (Blevin & Morrison 1997). The andesites appear to be extrusive equivalents of the quartz diorites. The TIC has intruded its volcanic equivalents and has produced a biotite hornfels zone within the andesites adjacent to the margin of the complex. Andesitic and rhyolitic dyke swarms are associated with the TIC. They occur outside the TIC and are cut by the main intrusive phases. Overall the TIC and the MLIC show close geochemical affinities, with the MLIC intrusions representing the felsic end and the main TIC phases representing the mafic-to-intermediate end of a single differentiation series, derived by fractional crystallization from a common parent magma.
A prominent magnetic low rims much of the TIC (Fig. 5). Lackie (1992a) showed that this feature is associated with reversed thermoremanent magnetization in the country rocks, acquired during emplacement of the TIC. The magnetic low lies slightly outboard of the metasomatized contact aureole, suggesting that it reflects thermal resetting of the remanence carried by the country rocks, rather than thermochemical remanence associated with secondary magnetite in the hornfels zones.
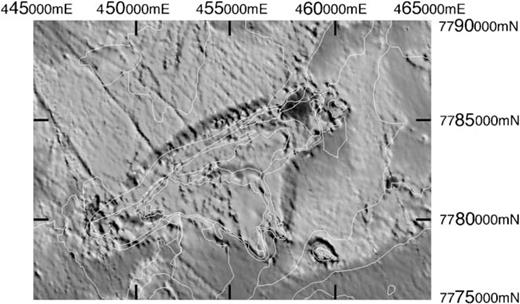
Image of the reduced-to-the-pole total magnetic intensity over the Tuckers Range area. The direction of magnetization used in the reduction algorithm was the Early Permian palaeofield direction recorded by the TIC. Mapped geological boundaries from Fig. 4 are shown in white. The dark bands that surround the main body of the TIC and the TIC outlier are reduced-to-the-pole highs (total magnetic intensity lows in the original data) that represent zones of reversed NRM owing to thermal overprinting of host rocks by the TIC in the Early Permian. After Schmidt & Clark (1998). This figure is in colour in the online version of the journal on Blackwell Synergy, where the dark bands are represented by magenta.
Webb (1969) obtained a K—Ar age of 283 ± 9Ma for the TIC, which is indistinguishable from the age of the MLIC. Recently, a U—Pb age of 287.4 ± 3.6Ma, based on analysis of 27 zircons, was found for the granodiorite phases (Blevin & Morrison 1997). This confirms that the TIC and the MLIC are coeval, in accord with their close geochemical affinities. Combining the radiometric dates from the two complexes, a common age of 286 ± 6 Ma can be assigned to the MLIC and the TIC.
Fig. 4 shows all sampling sites in the Tuckers Range (TR) area, superimposed on the detailed geology map of the TIC.
Methods
Standard palaeomagnetic sampling methods and laboratory techniques were used for this study (Butler 1992; McElhinny & McFadden 2000). Wherever possible, a suncompass was used to determine strike directions as well as a magnetic compass. Several standard cylindrical palaeomagnetic specimens (25 mm diameter, 22 mm height) were prepared from each sample. A CTF cryogenic (SQUID) magnetometer was used to measure remanent magnetization, except for very intense remanences, which were measured using a modified Digico spinner fluxgate magnetometer interfaced to a PC. Remanence components of differing stabilities were distinguished using alternating field (AF) and thermal demagnetization for all samples. Typically, at least one specimen from each sample was treated using each of the following demagnetization treatments or combinations of treatments.
AF demagnetization until the specimen is effectively demagnetized or the residual remanence is swamped by noise.
Thermal demagnetization until the specimen is effectively demagnetized or the residual remanence is swamped by noise.
Preliminary AF demagnetization to remove soft isothermal remanent magnetization (IRM) components, followed by thermal demagnetization.
Low-temperature (LT) cleaning (cooling specimens in liquid nitrogen and rewarming to room temperature in zero field), followed by AF and/or thermal treatment.
Well-resolved remanence components were defined by applying principal-component analysis (PCA) to the demagnetization data (Kirschvink 1980).
The magnetic mineralogy of samples has been characterized by techniques such as: (1) AF, LT and thermal demagnetization of Natural Remanent Magnetization (NRM); (2) low-field thermomagnetic (k-T) curves; (3) thermal demagnetization of three mutually orthogonal artificial remanence components with low, intermediate and high coercivity (Lowrie 1990); (4) high-field (∼800 mT) hysteresis loops; (5) high-field thermomagnetic (Ms-T) curves; and (6) low-temperature measurements involving the cooling of samples in field-free space, apply a saturating remanence at liquid nitrogen temperatures and then monitoring changes in this remanence as the sample warms up to room temperature.
Magnetic susceptibilities of palaeomagnetic specimens were measured using the CSIRO transformer susceptibility bridge (Ridley & Brown 1980), which is also equipped with a small furnace into which a silica tube containing a small crushed sample together with a platinum resistance thermometer can be placed for determination of k-T curves. Hysteresis loops, Ms-T curves and low-temperature measurements were carried out using a magnetic measurements variable field translation balance, developed at the University of Munich.
Rock Magnetism
Magnetic susceptibilities and Koenigsberger ratios of NRM are summarized in Tables 1 and 2 for the MLIC and TIC, respectively. Unblocking and AF demagnetization characteristics of NRMs (unblocking mainly in the range 500–580 °C and median destructive fields of several tens of millitesla) indicate that magnetite in the pseudosingle domain (PSD) size range (∼1–20 µm) is the dominant remanence carrier in most samples, but in some rock types the characteristic remanence is also carried by haematite and is not completely unblocked until 650–680 °C. When samples from the TIC and the MLIC are plotted on a plot of Mrs/Ms versus Hcr/Hc (Fig. 6), a continuous trend between the PSD field and the MD field is observed, consistent with a mixture of fine and coarse grains, in varying proportions (Dunlop & Özdemir 1997, p. 323).
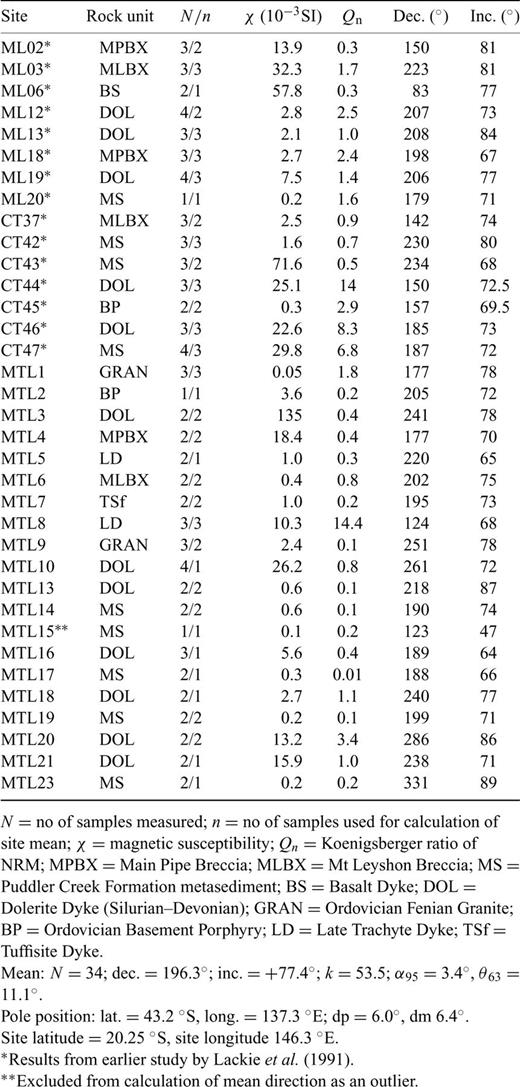
Permian site mean palaeomagnetic directions from the MLIC and its alteration zone.
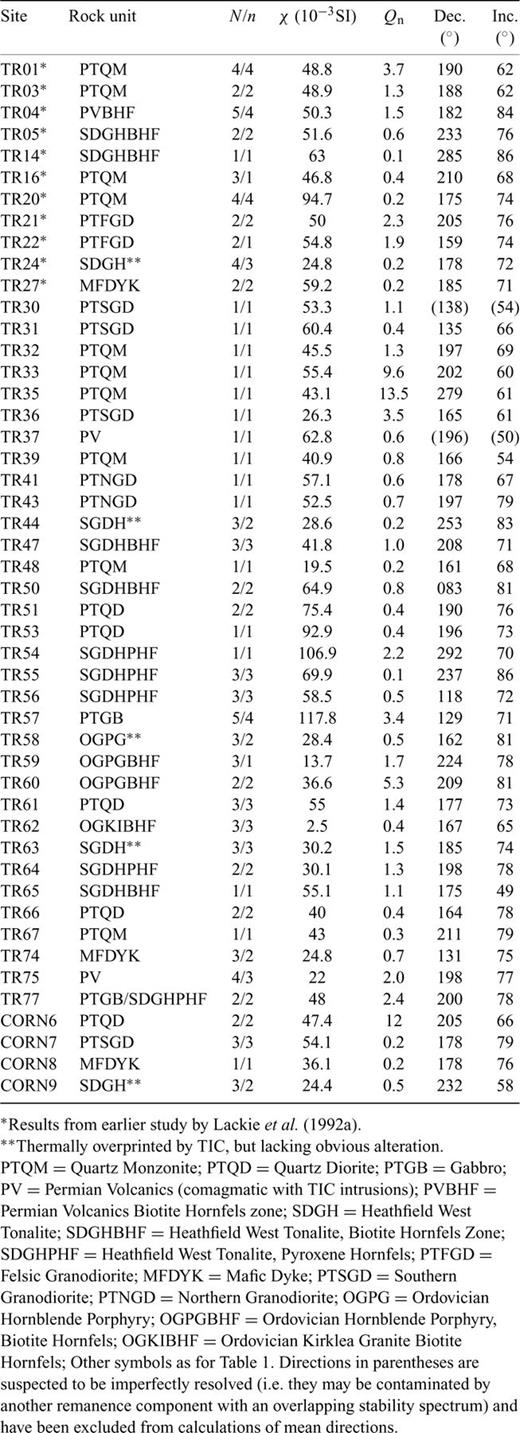
Permian palaeomagnetic directions from the tuckers igneous complex and its aureole.
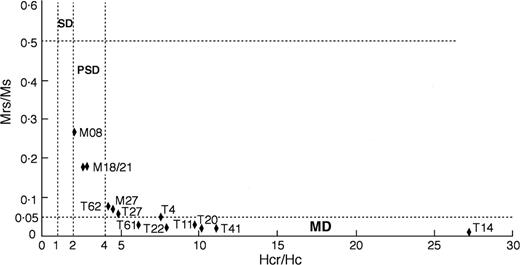
Plot of Mrs/Ms versus Hcr/Hc of samples from the MLIC and the TIC. The SD/PSD boundary at Hcr/Hc= 2 (Dunlop & Özdemir 1997, p. 321) differs from the somewhat arbitrary value of Hcr/Hc= 1.5 used by Day (1977).
High-field thermomagnetic curves (Ms-T) also confirm the presence of magnetite with Curie temperatures ∼580 °C (see Fig. 7). Most samples from the TIC (e.g. Figs 7c and d) show reversible thermomagnetic curves, whereas 50 per cent of the samples from the MLIC showed reversible curves. The irreversibility in all samples from the MLIC was a result of the presence of maghemite as well as magnetite (Fig. 7A and B), which breaks down to weakly magnetic haematite above 300 °C, producing a substantial decrease in magnetization between 300 and 400 °C. The cooling curve, after heating to 700 °C indicates pure magnetite as the only remaining strongly magnetic phase. The occurrence of maghemite is not attributable to weathering, as the samples from Mount Leyshon are essentially unweathered, but reflects subsolidus alteration during initial cooling of the volatile-rich, highly differentiated igneous phases and widespread propylitic alteration of the surrounding rocks.
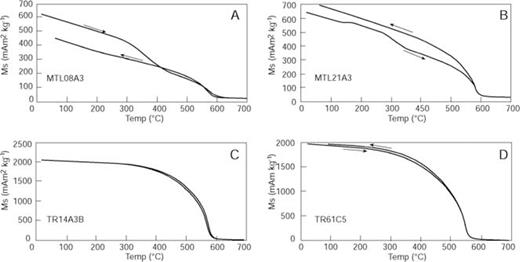
High-field thermomagnetic (Ms-T) curves. A, Trachytic Dyke; B, Dolerite Dyke; C, Heathfield West Tonalite Biotite Hornfels; D, Quartz Diorite.
The low-temperature experiments (Fig. 8) generally showed the characteristic drop in remanence at the Verwey transition (Figs 8A, C and D) of magnetite (Dunlop & Özdemir 1997, p. 53). The Verwey transition is suppressed in sample MTL08A3 (Fig. 8B). This is consistent with the high coercivities of samples from this site, reflecting single-domian (SD) grains that are dominated by shape anisotropy and can therefore retain most of their remanence, even when the magnetocrystalline anisotropy goes to zero. Partial oxidation of the SD magnetite grains, consistent with the presence of maghemite inferred from the thermomagnetic curves, would also suppress the Verwey transition in MTL08A3 (Özdemir 1993).
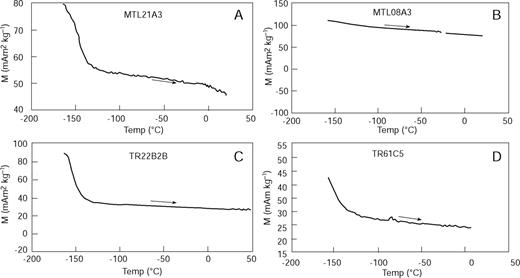
Low-temperature experiments. Samples were cooled in field-free space, then given a near-saturation remanence in an applied field of 800 mT at −170 °C and rewarmed in zero field to room temperature. A, Dolerite Dyke; B, Trachytic Dyke; C, Felsic Granodiorite; D, Quartz Diorite.
Palaeomagnetism of the Mlic and Host Rocks
AF and thermal demagnetization of most samples from the MLIC and adjacent host rocks generally reveals two components. Fig. 9 shows representative orthogonal plots for a number of samples, of differing lithologies. Low-stability components are present to varying degrees, but rarely dominant, and are usually readily removed. Many of the low-stability components are directed close to the present field direction and are interpreted as viscous remanent magnetization (VRM) acquired recently.
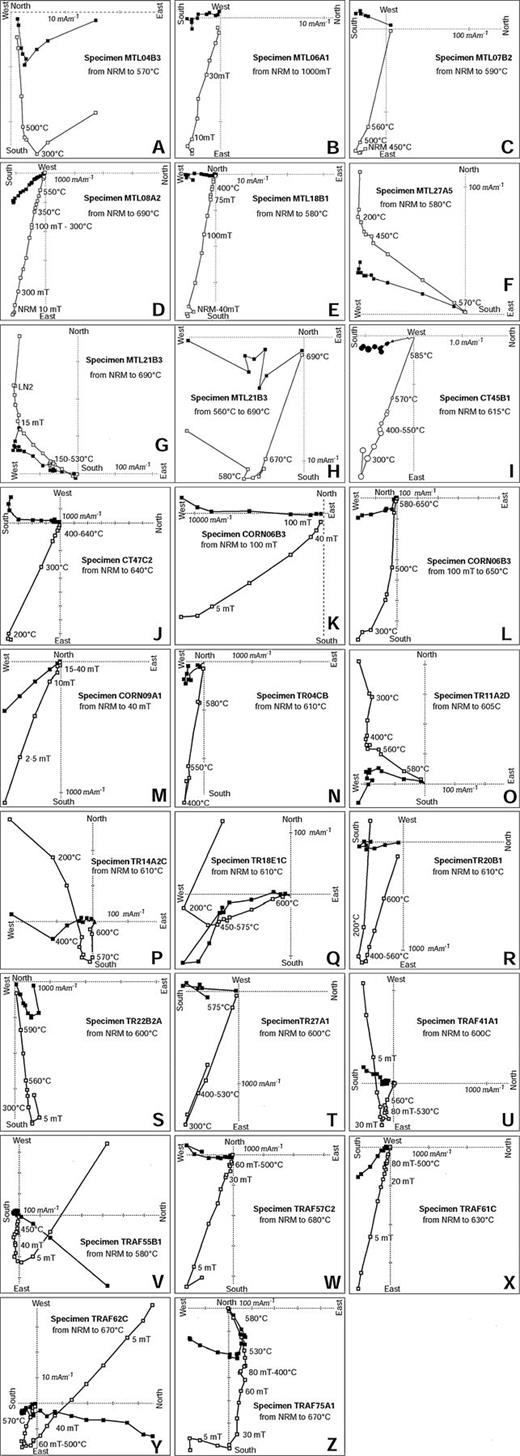
Representative vector component plots (Zijderveld 1967) for a number of oriented samples from the MLIC and its alteration zone and the TIC and its contact zone. LN2 indicates that the specimen was cooled in liquid nitrogen and rewarmed to room temperature in zero field. The vector component plots show successive remanence vector endpoints, projected on to the horizontal plane (closed symbols) and a vertical plane (open symbols). Plots H and L are expanded views of the near-origin part of plots G and K, respectively. Plots A to J are from MLIC specimens and plots K to Z are from TIC specimens. A, Main Pipe Breccia; B, Mt Leyshon Breccia; C, Tuffisite; D, Trachytic Late Dyke; E, Dolerite Dyke; F, overprinted Fenian Granite; G, H, I, J, overprinted Puddler Crk Formation; K, L, X Quartz Diorite Marginal Phase; M, Rishton Granodiorite (Sgt); O, Q, overprinted Heathfield West Tonalite (Sdgh); N, P, V, Y, Hornfels zones; R, Quartz Monzonite; S, Felsic Granodiorite (Fgd); T, Mafic Dyke; U, Northern Granodiorite (Ngd); W, Gabbro; Z, Andesite (Pv).
A remanence of high stability and reverse polarity, with steep inclination (Figs 9A-E and G-J) is the characteristic remanence observed from the MLIC. Unblocking and AF demagnetization characteristics (unblocking mainly in the range 500–580 °C, see Figs 9A and C) and median destructive fields of several tens of millitesla (see Figs 9B and D) indicate that magnetite in the pseudosingle domain size range (∼1–20 µm) is the dominant carrier in most samples, but in some rock types the characteristic remanence is also carried by haematite and is not completely unblocked until 650–680 °C (see Figs 9D and H). In these cases the directions of the magnetite and haematite components are indistinguishable (e.g. Sample 08A in Fig. 9D). Multidomain (MD) magnetite (>20 µm) is usually present and in some samples, coercivities extending above 100 mT (see Fig. 9E) in conjunction with unblocking just below 580 °C demonstrate the presence of significant quantities of single-domain magnetite.
Far (>2 km) from the margins of the MLIC samples show no trace of the Permian component. Some of the Silurian/Devonian dykes exhibit a distinctive WNW shallow up characteristic component that is interpreted to be a remanence of much greater age than the Early Permian MLIC component (e.g. the dyke sampled at site MTL27, see Fig. 9F). Samples at intermediate distances show variable degrees of overprinting of this older component by the south, steep down MLIC component. Adjacent to the MLIC the country rock samples are completely remagnetized. As discussed below, this pattern of overprinting constitutes a positive aureole test that confirms that the characteristic component of the MLIC samples was acquired during emplacement and early alteration of the intrusive complex.
Table 1 lists site mean Permian remanence directions from oriented samples that record the emplacement of the MLIC, either as a primary magnetization or as an overprint. The vector component plots for some samples exhibited curvature of the characteristic component, suggesting that the characteristic component was imperfectly resolved. For other samples the vector component plots were somewhat noisy and the characteristic component was poorly defined (maximum angular deviation >15°). After exclusion of such samples, results from 69 of the total 88 samples were used for calculation of the site mean directions in Table 1.
Fig. 10 shows an equal-area stereogram of the site mean Permian remanence directions. The Permian remanence components are well grouped about a mean direction of dec. = 196.3°; inc. =+77.4 °(N= 34; k= 53.5; α95= 3.4°, θ63= 11.1°). The palaeomagnetic pole corresponding to this direction is: lat. = 43.2 °S, long. = 137.3 °E (dp = 6.0°, dm = 6.4°). There is no indication in the data of systematic differences in directions recorded early in the evolution of the complex, such as the biotite—magnetite alteration in the country rocks and Main Pipe Breccia, and those recorded later (late dykes and porphyritic basalt dyke). This result confirms the earlier work of Lackie (1991), but represents a more complete sampling of the major events in the evolution of the complex, establishes a full baked contact/aureole test that proves the originality of the remanence, and improves the precision of the mean direction and associated pole.
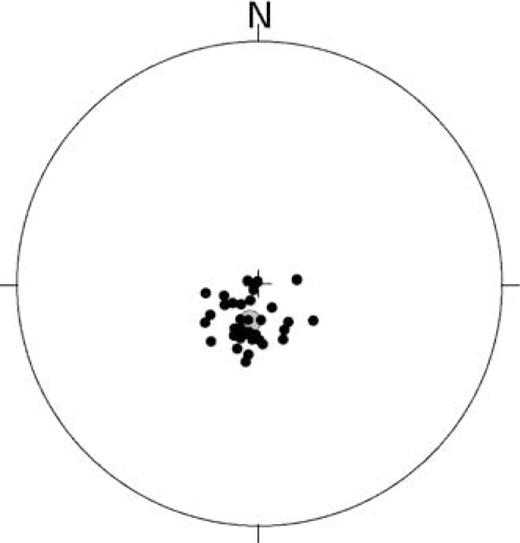
Site mean Permian remanence directions that record the emplacement of the MLIC, either as a primary magnetization or as an overprint step (filled symbols indicate directions on lower hemisphere). The shaded circle indicates the mean direction and associated error.
Palaeomagnetism of the Tic and its Aureole
Lackie (1992a) reported a preliminary petrophysical and palaeomagnetic study of the TIC and its host rocks. The present study substantially supplements those results. The total sample collection now comprises all intrusive phases of the TIC, apart from the minor aplites, as well as the comagmatic volcanics and mafic dykes, and covers much of the areal extent of the complex and its aureole. Sampling of country rocks at varying distances from the TIC provides a baked contact test of the originality of the stable remanence carried by the TIC rocks.
There are broad similarities in the palaeomagnetic character of the TIC and the MLIC. After removal of low-stability components by initial AF or thermal demagnetization, a stable magnetization directed south and steeply downwards is revealed for most TIC rocks (see Fig. 9). A few exposures are characterized by high remanent intensities, exceptionally high Koenigsberger ratios, and scattered remanence directions. These sites are almost certainly lightning-affected and in some of these samples the stable reverse polarity component could not be isolated. Many of the soft components removed during initial demagnetization are directed north with negative inclinations. As at Mount Leyshon, some of these directions are close to the present field and are attributed to recently acquired VRM, but other, generally somewhat more stable, components are significantly steeper than the present field and may represent an ancient overprint, possibly of Cretaceous age (e.g. Fig. 9R).
The stable reverse polarity remanence is found in all intrusive phases of the TIC (Fig. 9L, R, S, U, W and X), in its comagmatic volcanics (Fig. 9Z) and related mafic dykes (Fig. 9T) and in the aureole (Fig. 9N, P, V and Y). The directions of this component are very similar to those from the MLIC and are interpreted as Early Permian remanence acquired during initial cooling of the TIC. There is a systematic variation in the capacity to retain stable remanence between the igneous phases. The mafic rocks (gabbro and quartz diorite) contain PSD/SD, as well as MD, magnetite and these samples exhibit high coercivity and square-shouldered unblocking curves. These rocks retain the Permian remanence most consistently. The quartz monzonite is a moderately good palaeomagnetic recorder, but the granodiorite phases contain predominantly MD magnetite and retention of the Permian magnetization is less consistent in these rocks.
There is a distinct zoning of magnetic properties around the TIC, which is reflected in the detailed aeromagnetics (Schmidt & Clark 1998), as shown in Fig. 5. Owing to the deposition of secondary magnetite the metasomatized aureole around the TIC has a somewhat higher susceptibility than the unaltered equivalent rocks away from the complex. The pyroxene and biotite hornfels zones, which are up to a kilometre wide in places, exhibit the same steep reversed component that characterizes the igneous phases of the complex (Fig. 9N, P, V and Y). Although this component is ubiquitous around the margins of the TIC it is variably overprinted by low-stability normal polarity remanence and the resultant remanent intensities are reduced by competition between these opposing components. The relatively low Koenigsberger ratios and high susceptibilities of the hornfelsed Heathfield West Tonalite are similar to those of the major igneous phases of the TIC (Table 2), reducing the magnetization contrast at the margins of the complex. The magnetic signature of the TIC margin is therefore somewhat subdued and blurred.
Immediately outboard of the hornfels zone, the remanence of the Heathfield West Tonalite is still strongly overprinted by the TIC (Fig. 9M). The Heathfield West Tonalite contains primary PSD magnetite grains that are good palaeomagnetic recorders. Thermal overprinting of the unaltered tonalite is detectable within 0.5–1 km of the outer boundary of the hornfels zone, i.e. up to about 1.5 km from the TIC margin. The remanence carried by the thermally overprinted zone of the tonalite produces the distinct magnetic lows that surround the TIC, but lie slightly outboard of the metasomatized aureole. At distances greater than 1.5–2 km from the TIC the Silurian Heathfield West Tonalite retains a stable WNW shallow characteristic remanence, with no signs of Permian overprinting (Fig. 9O and Q). This characteristic direction is quite similar to that of a number of the dykes sampled in the Mount Leyshon area (see Fig. 9F and G), suggesting that they are also Silurian.
The pattern of overprinting around the TIC provides an excellent aureole test of the stability and originality of the characteristic remanence for the TIC. The aureole test comprises a classic baked contact test, as well as a consistency test that compares directions from the metasomatized aureole, the intrusive rocks and the thermally reset country rocks. The timing of remanence acquisition throughout the aureole probably depends on local proximity to particular intrusive phases and should reflect variable overprinting by subsequent intrusions. This suggests that the palaeomagnetic record from widely dispersed samples around the aureole may span much of the history of the TIC.
Table 2 lists site mean directions for well-defined Permian components from the Tuckers Range area. The selection criteria for excluding samples that were applied to the results in Table 1 were also used for the data of Table 2. Sample mean Permian components from the Tuckers Range area, grouped according to rock type, are plotted in Figs 11A-D, with their corresponding mean directions and 95 per cent cones of confidence. The directions from the mafic, intermediate and felsic intrusive phases, and also from the aureole, are well-grouped, suggesting that they are drawn from a single population that represents the average palaeofield direction during emplacement of the individual intrusions that comprise the TIC.
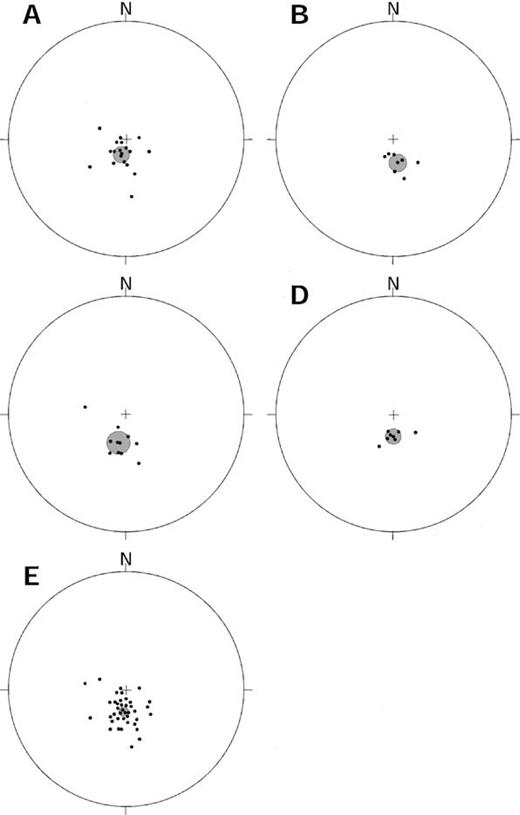
Sample mean Permian remanence directions from the Tuckers Range area, grouped according to rock type. A, hornfels and thermally overprinted zones; B, granodiorites; C, quartz monzonite; D, quartz diorite and gabbro; E, all the data. Shaded circles indicate the mean direction and the associated error.
It is clear from Fig. 11 and from the ‘rock unit means’ in Table 3 that there is no significant difference between the various rock types. The angular dispersion (θ63= 13.7°) of the combined site mean directions in Fig. 11E is slightly less than that expected for secular variation at high latitudes. For a palaeolatitude of 62°, which corresponds to the palaeoinclination of 75°, the dispersion owing to secular variation is expected to be about 20°(Butler 1992, pp. 164–166). This suggests that secular variation has been partially averaged, even within sites. Given the timescale of remanence acquisition, relative to that of secular variation, it is reasonable to average all site means to give a time-averaged palaeofield direction for the TIC: dec. = 188.7°, inc. =+75.2°; N= 42; k= 35; α95= 3.8°. The corresponding palaeopole position is: lat. = 47.5 °S, long. = 143 °E (dp = 6.0°, dm = 6.6°). The aureole test affords good evidence that the characteristic remanence of the TIC is primary and represents the palaeofield direction during emplacement of the TIC around 287 Ma.
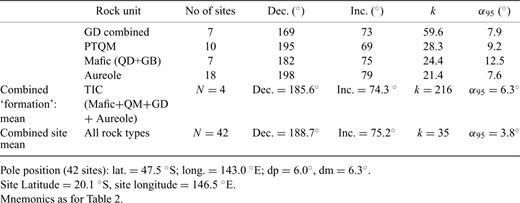
Mean permian palaeomagnetic directions from the TIC and its aureole.
Age of Magnetization
The acquisition of remanence by the various lithologies at Mount Leyshon spanned about 10 Ma, thus secular variation should be well averaged by the total sample collection. This conclusion is supported by the relatively high precision parameter (k= 53.5) and correspondingly low angular dispersion (11°) of the site mean directions. The overprint magnetizations present at many sites are of thermochemical origin and were probably acquired over periods sufficient (>10 000 yr) to completely average secular variation. The total time span is insufficient to produce significant dispersion of directions owing to apparent polar wander, except at times of very rapid continental drift, and there is no evidence of any streaking of directions, which could reflect apparent polar wander. Thus the relatively tight clustering of site mean directions, and the magnetic petrology, are consistent with some averaging of secular variation within sites and possibly even within samples. A fortiori the mean direction calculated from all sites averages secular variation and can be used to calculate a palaeomagnetic pole that corresponds to the ancient axis of rotation (as opposed to a virtual geomagnetic pole (VGP), which represents a spot reading of the palaeofield and can depart from the geographic palaeopole by up to 20°).
The Early Permian remanence direction of the MLIC and host rocks is recorded by (in order of acquisition):
potassically altered (i.e. containing secondary biotite and magnetite) country rocks adjacent to the MLIC, including Puddler Creek metasediments, dolerite dykes, Fenian Granite and Basement Porphyry (earliest overprint magnetization; ca. 290 Ma);
Main Pipe Breccia (earliest overprint magnetization; ca. 290 Ma);
Early dykes (early? primary magnetization; ca. 290 Ma);
Mount Leyshon Breccia (early to late overprint magnetizations; ca. 290–280 Ma);
Mine Porphyry, Wallaby Tail Porphyry and Southern Porphyry (early to late? primary magnetizations; ca. 290–280 Ma);
Late dykes and Tuffisites (late primary magnetization; ca. 285–280 Ma);
Porphyritic Basalt dyke (latest primary magnetization; ca. 280 Ma).
The tight clustering of directions (Fig. 10) throughout this sequence of remanence acquisition events supports the geological, geochemical and radiometric dating evidence that the evolution of the MLIC was completed rapidly (relative to the timescale appropriate to apparent polar wander). The resolution of the palaeomagnetic method is normally insufficient to constrain events of this age to closer than 10 Ma. The wide range of lithologies, with magnetic carriers of variable composition and microstructure, which exhibit the characteristic palaeomagnetic direction affords a consistency test of the stability of the remanence.
A notable feature of the palaeomagnetism of the MLIC is that most rock types are excellent palaeomagnetic recorders, with fine-grained magnetite as the dominant magnetic carrier. In the late dykes and associated porphyries this magnetite is a primary igneous mineral that should have retained a primary thermoremanent magnetization, unless the rocks have been subsequently altered or strongly heated. The Basalt Porphyry dyke (formerly known as the trachyandesite) has a radiometric age of 281 Ma and post-dates all other igneous and hydrothermal events at Mount Leyshon. This dyke is expected to carry a primary Early Permian remanence. The early dykes have a pervasive phyllic alteration that is probably autometasomatic. Thus remagnetization of these dykes owing to the alteration would have occurred immediately following emplacement, at ∼292 Ma, and the remanence carried by these dykes can therefore be regarded as effectively primary (unless subsequently overprinted locally by other alteration). In the Main Pipe Breccia and the wall rocks of the complex, the magnetite is generally associated with the early potassic (biotite—magnetite) alteration and is therefore secondary, albeit early in the history of the complex. The remanence acquired by this magnetite, and any coeval haematite, is therefore thermochemical remanent magnetization. The prominent negative magnetic anomaly at Mount Leyshon is largely attributable to remanent magnetization carried by secondary magnetite associated with this alteration (Sexton 1995). The early potassic alteration appears to immediately precede emplacement of the early dykes.
In the earlier study of Lackie (1991), sampling of country rocks was restricted to the immediate vicinity of the MLIC. Those samples were remagnetized by the Mount Leyshon alteration system. The more recent sampling has allowed palaeomagnetic signatures that pre-date the MLIC to be studied, where they are preserved in country rocks that are weakly affected or unaffected by the Mount Leyshon alteration (Fig. 12). This sampling also allows a contact test of the primary nature of the characteristic remanence component from Mount Leyshon. At distances of several kilometres from Mount Leyshon, the effects of the Mount Leyshon alteration on the magnetization are generally undetectable (see Fig. 9F). Closer to the complex, a Permian component often partially overprints more ancient high-stability components. For example, Figs 9G and H show the behaviour of a relatively distal dyke sample, which has a WNW shallow up component carried by magnetite, weakly overprinted by a steep down component carried by haematite. This is interpreted as chemical overprinting of the remanence carried by primary magnetite in this dyke by distal fluids emanating from the MLIC. Distal fluids in such systems are cooler, are often mixed with oxygenated ground waters and produce haematite as part of the lower-temperature propylitic alteration assemblage, whereas the higher-temperature proximal fluids associated with early potassic alteration tend to produce secondary magnetite in equivalent country rocks.
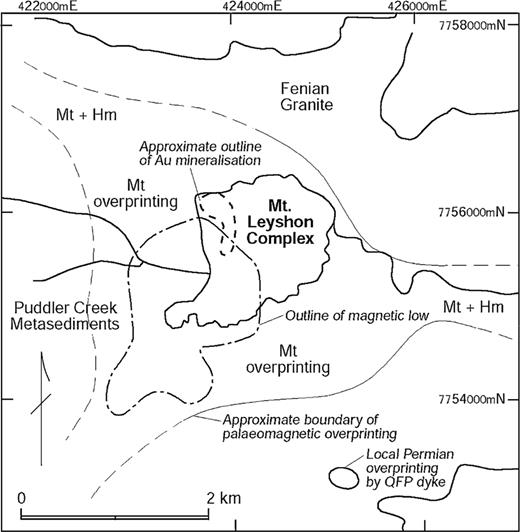
Zonation of alteration overprinting around the MLIC. The outline of the magnetic low corresponds to the zone of intense early potassic (biotite—magnetite) alteration. Complete remagnetization of host rocks occurs adjacent to the MLIC and grades outwards into partial overprinting of older remanence, well beyond the detectable aeromagnetic anomaly, and is zoned from proximal magnetite to distal haematite as the secondary magnetic minerals within pre-Permian host rocks. The thick lines (solid = accurate; dashed = approximate) indicate the outer limits of detectable overprinting.
Somewhat closer to the MLIC, some similar dykes exhibit an intermediate-temperature Early Permian component, carried by magnetite, which partially overprints the high-temperature magnetite component that is characteristic for the dyke. This overprint may in some cases represent purely thermal partial overprinting of the characteristic component, carried by primary magnetite, of the dykes. However, given the evidence for thermochemical magnetizations carried by hydrothermal magnetite adjacent to the MLIC, the overprints at intermediate distances may in some cases also be thermochemical. Within the zone of intense alteration around the complex the rocks are completely remagnetized and only carry the Permian component (Fig. 9E). These observations provide an aureole test of the effectively primary nature of the characteristic remanence, analogous to the classic baked contact test of palaeomagnetic stability (Butler 1992, pp. 128–129).
The additional sampling carried out for this study has confirmed the result obtained earlier by Lackie (1991), but has improved the precision and reliability of the palaeopole from the MLIC. This palaeopole records events that effectively span the full 10 Ma evolution of the complex. There is now a full aureole test of the stability and originality of the characteristic remanence of the MLIC and the corresponding pole may be regarded as a key palaeomagnetic pole for the Early Permian (286 ± 6 Ma) of Australia.
Given the large size of the main component intrusions of the TIC and of the complex as a whole, and the fact that thermal effects are detectable 1–2 km from the margins, cooling of the intrusions must have occurred over a considerable period. This would tend to average secular variation, especially since the samples were collected over a wide area. The low dispersion of the site mean directions indicates some averaging of secular variation within sites. The even tighter grouping (k= 216; θ63= 5.5°) for the four main events (emplacement of mafic, intermediate and felsic phases; hornfels formation) suggests first that each of the four ‘formation mean’ directions given in Table 3 has a largely averaged secular variation and, secondly, that negligible apparent polar wander has occurred during formation of the TIC. It is therefore appropriate to calculate the palaeopole position from the mean direction of all sites, as given above.
The thermal effects of intrusions have been modelled quantitatively by Jaeger (1957, 1959, 1964). Schmidt (1990) uses this theory to derive profiles of maximum temperature versus distance from the centre of a basaltic dyke. These profiles can be used to predict the approximate extent of thermal resetting of remanence adjacent to the TIC. If the ambient temperature of the wall rocks into which the TIC magmas were injected was ∼100 °C, the maximum temperature attained by the wall rocks was ∼540 °C at a distance of 1 km from the contact, ∼430 °C at 2 km and <320 °C greater than ∼4 km from the contact (assuming an intrusion temperature of 1150 °C, a dyke thickness of 4 km, latent heat of crystallization of 90 cal g−1, specific heat of magma = 0.3 cal g−1°C). At the contact, the calculated increase in temperature is ∼680 °C. The pyroxene hornfels assemblage developed adjacent to the contact is consistent with temperatures of ∼700–800 °C, in agreement with the calculated temperature rise. If the host rocks were 50 °C cooler at the time of emplacement the maximum temperatures attained are reduced by approximately 50 °C at each distance. The observed pattern of palaeomagnetic overprinting (resetting within ∼1 km, negligible overprinting >2 km from the contact) appears to be consistent with purely thermal overprinting of PSD magnetite by a steep-sided, hot, mafic intrusion. The extent of the overprinted zone does not require outward-dipping contacts for the main body of the TIC. However, the relatively broad magnetic low around the southern TIC outlier suggests that at shallow depths this multiple intrusion may be broader than its current exposure.
Figs 10 and 11E show the mean directions from the TIC and the MLIC, both of which are well defined (α95 < 4°), are indistinguishable within the error bounds. Using the discrimination test of McFadden & Lowes (1981) for the TIC and MLIC data we obtain a G statistic of 0.93, which is much less than the 95th percentile value for F(2, 148) of 3.06, showing that the directions are not significantly different. This accords with their indistinguishable radiometric ages and the geochemical evidence that demonstrates a genetic link between their parent magmas.
Late Palaeozoic Apwp for Australia
The palaeopoles from this study contribute to a substantial set of reliable Palaeozoic palaeomagnetic poles from Australia and other continents that have been published in recent years and it is timely to revisit the question of the Australian and Gondwana Palaeozoic APWPs using this enhanced database. Table 4 lists apparently reliable palaeomagnetic poles from Australia that are used to construct the proposed mid-Palaeozoic to Mesozoic APWP for Australia (Fig. 13). Stratigraphic and radiometric ages are correlated using the recently revised Phanerozoic timescale produced by the Australian Geological Survey Organization (Jones 1995). The most significant differences with the Harland (1990) timescale relate to the Carboniferous, in particular, and to a lesser extent the Permian. These differences are quite significant for intercontinental comparisons of pole positions, for determination of the duration of the Permo-Carboniferous Reverse Superchron (PCRS) and for detailed determination of the APWP of Gondwana, as rapid continental motion occurred during the Carboniferous. The Late Carboniferous—Late Permian interval is characterized by the longest known period of single geomagnetic polarity, the Permo-Carboniferous Reverse Superchron, which was originally termed the ‘Kiaman Magnetic Interval’ by Irving & Parry (1963). The upper and lower limits of the PCRS are not defined very precisely. McElhinny & McFadden (2000) give 262–316 Ma as the probable duration of the PCRS. Recent data from the New England Fold Belt in eastern Australia suggest that the base of the PCRS occurs at 317 ± 1 Ma (Opdyke 2000).
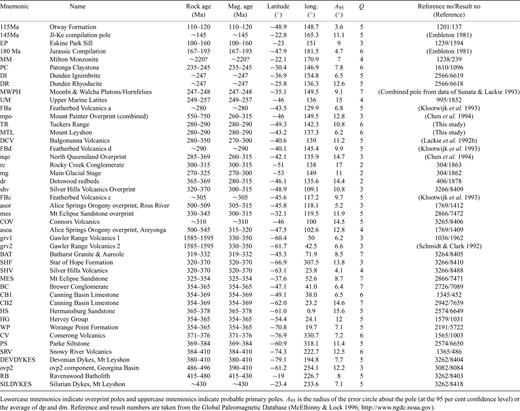
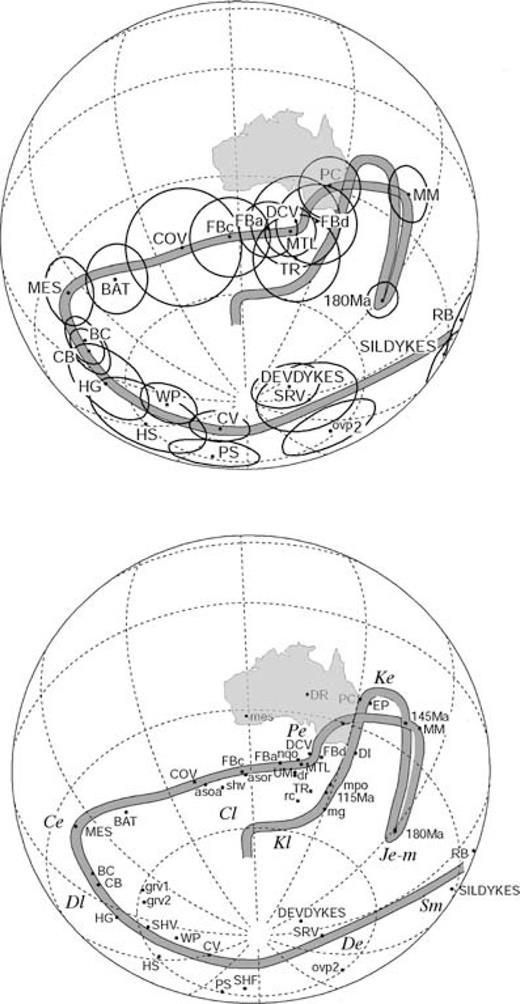
(a) Selected Silurian—Jurassic palaeopoles from Australia, with 95 per cent ovals of confidence, (b) Mid-Silurian to Late Cretaceous APWP for Australia with poles from the present study and selected other poles from Australia (mnemonics listed in Table 4).
The new poles from Mount Leyshon and Tuckers Range are both precise and the magnetization age (286 ± 6 Ma) is tightly constrained by radiometric dating, field relationships and baked contact/aureole tests. They can therefore be regarded as key poles for the Early Permian. These poles are consistent with, and lie between, the rather imprecise Early Permian (275 Ma) and the better defined Late Carboniferous A (305 Ma) mean poles for Australia, calculated from older data by McElhinny & McFadden (2000). The MTL and TR poles also lie very close to the DCV pole from the Late Devonian to Permo-Carboniferous volcanics of the Bulgonunna Volcanic Field (Lackie 1992b). Klootwijk (1993) sampled this sequence in detail and provided constraints on the age of magnetization, as well as obtaining very similar palaeopoles. The remanence of the Bulgonunna Volcanics is interpreted as being acquired very soon after extrusion (syn-sagging) in the youngest rocks, and an essentially identical component is recorded as an overprint in the older units. Radiometric dating suggests that the age of magnetization in these rocks is between 295 Ma (the age of the youngest rocks) and 280 Ma (the end of hydrothermal alteration), which is consistent with the age of the TIC and MLIC.
Klootwijk (1993) identified a Permian overprint in a number of other Permo-Carboniferous rock units in North Queensland. Chen (1994) calculated a combined North Queensland Overprint (nqo) pole from these data, which lies close to the group of well-dated Early Permian poles. The Late Devonian Dotswood Redbeds, Queensland, carry a reverse polarity magnetization (Chamalaun 1968). This magnetization is interpreted here as a Permo-Carboniferous overprint, as the corresponding pole (dr) falls close to the Early Permian group of poles, as does an overprint pole (mpo) associated with Permo-Carboniferous hydrothermal activity within the uraniferous breccia horizon at Mount Painter, South Australia (Idnurm & Heinrich 1993).
Klootwijk (1993) also studied the Permo-Carboniferous Featherbed Volcanics of North Queensland. Poles FBa (280 Ma) and FBd (290 Ma) are consistent with the Early Permian poles of this report, whereas FBc (305 Ma, i.e. Late Carboniferous) is displaced slightly west of the Early Permian Group. The Main Glacial Stage (mg) and Rocky Creek Conglomerate (rc) poles of Irving (1966), from Carboniferous sequences in the Tamworth Belt (NSW), have traditionally been used to define the Late Carboniferous pole position. These poles plot close to the FBa, FBd, MTL and TR poles, which suggests that the age of magnetization for mg and rc may be Early Permian, as originally suggested by Lackie & Schmidt (1993). Alternatively, the mg and rc poles may be affected by local block rotations about vertical axes, which are known to occur elsewhere throughout the New England Fold Belt (Geeve 2000).
Most other older results from Carboniferous and Permian rocks from Australia are now regarded as unreliable, being accorded C or D ratings by Powell (1990), where only poles with A or B ratings should be used to define the APWP and only A poles are sufficiently well defined and constrained in age to calibrate the path.
A magnetostratigraphic study of the Late Permian Newcastle Coal Measures and correlated sequences (Sydney and Gunnedah Basins) has defined a dual-polarity high-temperature component, after removal of the ubiquitous Cretaceous overprint (Théveniaut 1994). This magnetization is interpreted as being a primary remanence acquired around 258 Ma, although recent dating suggests that the age of the sampled sequence might be closer to 250 Ma (Roberts 1994). The dual polarity indicates that the magnetization postdates the end of the PCRS. The corresponding pole falls in the Great Australian Bight (H. Théveniaut, private communication, 2001) and is supported by the UM pole from the mid- to Late Permian Upper Marine Latites of the southern Sydney Basin (Irving & Parry 1963). The magnetizations from the Upper Marine Latites (now known as the Gerringong Volcanics) are uniformly reversed, suggesting that the volcanics were extruded during the PCRS. Given the crude attitude control and possible insufficient averaging of secular variation, Powell (1990) gave the UM pole a B−rating. Because of its relatively large error (A95= 15°), the 95 per cent confidence circle around the UM pole also overlaps the Early Permian group of poles.
The earliest Triassic VGPs from the Dundee Ignimbrite (DI) and Dundee Rhyodacite (DR) determined by Lackie (1988) supplement the reliable Early Triassic PC pole from the Patonga Claystone of the Sydney Basin (Embleton & McDonnell 1981). An Early Triassic pole position within SE Australia, close to PC, is supported by results from Early Triassic sedimentary rocks of the Sydney and Gunnedah Basins (Théveniaut & Klootwijk, in preparation). The inclination error, which can be significant for terrestrial sediments in particular, is negligible for the rocks of the Sydney and Gunnedah Basins, as the recorded inclination is almost vertical. The discordance between the DR and DI poles, which are assigned identical (albeit imprecise) ages, is probably caused by insufficient averaging of secular variation, whereas the penecontemporaneous PC pole is derived from a stratigraphic section that records at least three field reversals and is probably a true palaeomagnetic pole for the Early Triassic (∼245 Ma). Other latest Permian—earliest Triassic (247–48 Ma) poles from the post-tectonic Moonbi and Walcha Road plutons and their respective hornfels aureoles in the New England Fold Belt confirm that the palaeofield at that time was almost vertical, placing the palaeopole within, or very close to SE Australia (Sunata & Lackie 1993). Results from these units have been combined in Table 4 to give a well-defined Early Triassic palaeopole (MWPH: lat. = 35.1 °S, long. = 149.5 °E, A95= 9.1°; Q= 7) with a positive contact test. Here Q is the quality index of Van der Voo (1990).
The well-defined pole (MM) from the? Early Triassic Milton Monzonite (Schmidt & Embleton 1981) has been regarded as discordant, with the suggestion that the unpublished radiometric age of ∼245 Ma may be unreliable. This pole position, and the probable primary nature of the characteristic component, have recently been confirmed by Dunlop (1997). The MM pole position lies on the Middle to Late Jurassic ‘Coral Sea loop’ of Schmidt & Embleton (1982), which suggests that intrusion could be much younger (∼150 Ma). However, given the rapid and complex motion of the Australian continent during the Mesozoic, the MM pole may simply record a hitherto missed portion of a slightly more complicated APWP. This possibility, however, requires that the reported K—Ar age of the Milton Monzonite be slightly too old, perhaps owing to excess argon, given that the nominal ages of the DR, DI, MWPH and MM poles are indistinguishable. An interpolated age of ∼220–230 Ma for the Milton Monzonite would be consistent with fairly constant motion of the pole around a loop linking the mean Early Triassic (DR, DI, PC, MWPH) pole in SE Australia and the cluster of poles SW of New Zealand that belong to the Early Jurassic quasistatic interval, which lasted from ∼200 to ∼170 Ma (Embleton 1981). Another possibility is that the MM pole has not averaged the secular variation sufficiently, i.e. it is a VGP rather than a palaeomagnetic pole. However, the MM pole is derived from a number of intrusive phases of a large composite intrusion, which would suggest that the primary magnetization has been acquired over a timescale of duration sufficient to largely average the secular variation.
McElhinny & McFadden (2000) show an excursion of the Australian APWP to the present geographic pole ca. 320 Ma. This ill-defined Late Carboniferous B mean pole is based on only two results: the Yetholme Adamellite (Facer 1977) and the Paterson Toscanite (Irving 1966). The magnetization direction of the Yetholme Adamellite is indistinguishable from the present dipole field direction and probably represents a recently acquired viscous remanence. The Paterson Toscanite is now known to be much older (ca. 328 Ma) than its originally assigned age and its pole is incompatible with poles from rocks of similar age in other parts of Australia. The Paterson Toscanite occurs within a part of the southern New England Fold Belt that is affected by rotations of fault-bounded blocks about vertical axes (Geeve 2000), so its pole is probably not representative of cratonic Australia.
Rather than exhibiting an excursion to the vicinity of the present pole, the 330–310 Ma segment of the APWP is constrained by the Connors Volcanics (COV) pole of Clark (1994) and the BAT pole of Wahyono (1992) to follow a fairly consistent NE trend from the Early Carboniferous position, well to the SW of Australia, to the Permo-Carboniferous position immediately south of Australia. The latter pole is derived from dual-polarity magnetizations, interpreted as primary on the basis of positive contact tests, from the mid-Carboniferous (325 ± 5 Ma) Bathurst Granite, related mafic intrusions and contact aureoles. Thus the high-quality (Q= 7) BAT pole helps to define and calibrate the northeastward movement of the pole during the mid-Carboniferous. Two recent results from central (cratonic) Australia (MES and BC poles) suggest that the APWP underwent a sharp bend around the Devonian/Carboniferous boundary, with the Mount Eclipse Sandstone (MES; ∼345 Ma) pole approximately marking the southwestern extremity of the APWP (Chen 1993).
The extent of the polar shift implies rapid motion of Australia during the Carboniferous, as was first recognized by Irving (1966). Australia occupied low latitudes in the Early Carboniferous, but had moved to near-polar latitudes by the Late Carboniferous. Movement of the pole through 60° in about 30 Ma translates to ∼20 cm yr−1, which is at the upper range of plate velocities since the breakup of Pangaea. This estimated rate of apparent polar motion actually represents a lower bound for the plate motion, since components of plate motion along parallels of palaeolatitude are unresolvable by palaeomagnetism.
The poles (asoa, asor) derived from overprint magnetizations associated with the mid-Carboniferous (315 ± 10 Ma) Alice Springs Orogeny of central Australia fall west of the Permo-Carboniferous pole position (Klootwijk 1980). Because these overprints are of dual polarity, they pre-date the PCRS, favouring a mean acquisition age of 316–325 Ma. A pre-folding (i.e. pre-Triassic) magnetization carried by upper sequences of the Carboniferous Connors Volcanics in central Queensland yield a pole (COV) to the SW of the Late Carboniferous pole position (Clark 1994). These rocks were all reversely magnetized, suggesting that they were extruded during the PCRS. An overprint magnetization of reverse polarity found in the Devonian/Carboniferous Silver Hills Volcanics of the Drummond Basin (Klootwijk 1993) yields a pole (shv) that lies close to COV and the Alice Springs Orogeny overprint poles. Thus the rapid polar motion of the Early Carboniferous continued into the beginning of the PCRS, but appears to have slowed dramatically shortly thereafter as a prelude to the Permian quasistatic interval.
Fission track data from the Gawler Craton (Ferguson 1981) have revealed a slow cooling event around the Early Carboniferous (mean of 13 ages = 331 ± 30 Ma, 1σ). Schmidt & Clark (1992) found that steeply dipping intermediate and felsic lavas of the Proterozoic Gawler Range Volcanics, South Australia, carry a NE up magnetization. This magnetization is identical to the characteristic component of flat-lying Gawler Range Volcanics that were extensively sampled by Chamalaun & Dempsey (1978). That component was originally interpreted as a primary magnetization and the Gawler Range Volcanics pole (grv1) was regarded as a key pole for Australia ca. 1600 Ma. It is now apparent that the characteristic magnetization of the Gawler Range Volcanics is an overprint, acquired post-tilting. The timing of movement on the bounding fault that is responsible for the tilting is uncertain, but is probably much younger than the volcanics. The grv1 and grv2 poles lie close to the HG and SHV poles from Australia, suggesting a Late Devonian age of magnetization. It seems likely that this magnetization was acquired during the thermal event that affected the Gawler Craton and reset the fission track ages. The unimodal, negatively skewed distribution of fission track lengths reflects a slow, steady cooling history and the ages recorded by the fission track data represent cooling through the ∼100 °C isotherm, perhaps tens of millions of years after the peak temperatures were attained and associated overprint magnetizations were acquired.
Fig. 13 shows that rapid westward movement of the south pole position with respect to Australia occurred through the Devonian, before the cusp in the Early Carboniferous near the MES pole. This trend is defined by poles from both cratonic Australia (BC, CB1, CB2, HS, PS) and eastern Australia (HG, SHV, WP, CV, SHF, SRV). The DEVDYKES pole (Clark 1996) from dolerite dykes in the Mount Leyshon area, outside the MLIC aureole, agrees with the Early Devonian (∼400 Ma) Snowy River Volcanics (SRV) pole. Because these results are from sites that are separated by about 1600 km and belong to different fold belts within eastern Australia (Thompson and Lachlan Fold Belts, respectively), their concordance supports the autochthony of the terrane from which the SRV pole is derived. The SRV pole is also consistent with the Aïr pole from Africa (Hargraves 1987), which was originally assigned an age of ∼435 Ma but has been redated with a revised age of 407 ± 8 Ma (Moreau 1994) that is compatible with the Snowy River Volcanics.
In Fig. 13 the trend of the Devonian APWP extends back to mid-Silurian (∼430 Ma) poles (Clark 1996) from older dykes around Mount Leyshon (SILDYKES) and from Silurian plutons of the Ravenswood Batholith and their contact metamorphosed host rocks (RB). The APWP shown in Fig. 13 indicates that rapid apparent polar wander also occurred from the mid-Silurian to the Early Carboniferous, suggesting that motion of Australia and, by implication, Gondwana was relatively rapid throughout a substantial portion of the Palaeozoic.
Tectonic and Palaeogeographic Implications of New Results
Mean poles compiled by McElhinny & McFadden (2000) for Australia and other Gondwana continents are plotted in Australian coordinates, together with the proposed late Palaeozoic APWP, in Fig. 14. Rotations of data from other continents to the Australian frame of reference used the Euler poles given by Lottes & Rowley (1990), which allow for post-Permian intracontinental deformation of Africa. There is broad agreement between the mean poles from continents other than Australia and the corresponding segments of the Australian APWP, although gaps in the record for some other continents and some systematic differences with the Australian APWP remain.
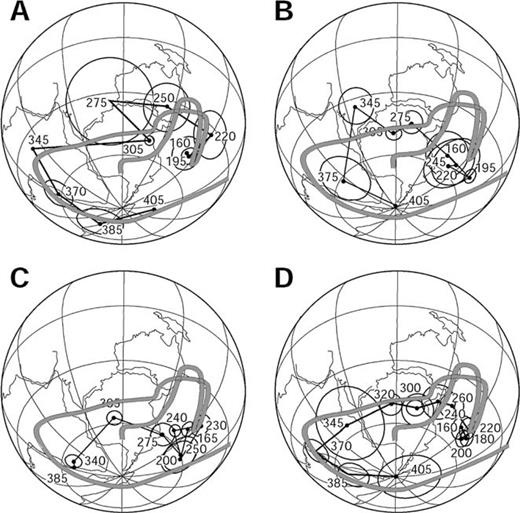
Proposed Palaeozoic APWP for Gondwana, based on the Australian APWP of Fig. 14. For comparison, means of apparently reliable poles from Australia and other continents, as calculated by McElhinny & McFadden (2000), are plotted, with corresponding mean ages juxtaposed: (a) mean poles from Australia, (b) Africa, (c) South America (d) Gondwana (combined). The Gondwana reconstruction is according to Lottes & Rowley (1990).
Most interpretations of the Gondwana APWP have inferred a prolonged quasi-static interval during the Late Carboniferous—Late Permian period, which coincides approximately with the PCRS. Recognition of the prevalence of remagnetization during the Late Palaeozoic suggests that this apparent quasistatic interval may be partly an artefact of Permian overprinting of Carboniferous rocks. However, the evidence from the more reliable results certainly indicates that polar motion was quite slow in the interval from the latest Carboniferous to the earliest Triassic. The Australian data now confirm that the pole position moved from immediately south of the continent into eastern Australia during this period. This track lies significantly north (in present Australian coordinates) of many proposed Gondwana APWPs for the Late Palaeozoic (e.g. Bachtadse & Briden 1990), which have been largely based on older results from Africa, many of which are of low quality or can now be recognized as unreliable.
One older result, the Dwyka Varves pole of McElhinny & Opdyke (1968), was long regarded as a key Late Carboniferous pole for Africa and was accordingly used to constrain older versions of the Gondwana APWP. As discussed by McElhinny & McFadden (2000), it has become increasingly apparent with the accumulation of new data that this pole is quite discordant, probably owing to tectonic complexities associated with rifting in the Galula coalfield that were originally unrecognized. The older result is now superseded by a study (Opdyke 2001) that is based on extensive resampling of the Dwyka System, avoiding structural complications that affected the earlier study, which yields a substantially different palaeopole. Rotated to Australian coordinates, the new reliable (Q= 7) Dwyka System Late Carboniferous pole, based on 22 sites, is: lat. = 46.8 °S, long. 127.6 °E (A95= 12°), which is in good agreement with the Late Carboniferous section of the Australian APWP shown in Fig. 13.
A new palaeopole from Lower Serpukhovian to Lower Moscovian (∼325–305 Ma) sedimentary rocks of Algeria (Derder 2001) is the first Carboniferous pole from Africa that is based on both positive reversal and fold tests. The results suggest that the base of the PCRS occurs within the Lower Serpukhovian. Rotated to Australia, the palaeopole is: lat. = 57.0 °S, long. = 104.8 °E (A95= 4.7°; Q= 7), which lies slightly south of the mid-Carboniferous portion of the Australian APWP drawn through COV in Fig. 13, but is nevertheless consistent with the COV pole, which has a relatively large error (A95= 14.5 °).
A high-quality data set (Q= 6) from Tanzania (Nyblade 1993) gives the best indication of the Late Permian palaeopole position for Africa. Based on stratigraphic ages of the sampled Karoo sequences this pole represents the 264 ± 10 Ma palaeofield in Tanzania. The directions used to define this pole position are all of reverse polarity, suggesting that the entire sampled sequence was probably deposited during the PCRS. After rotation to Australian coordinates using the Euler poles of Lottes & Rowley (1990), the pole position becomes 35 °S, 142 °E (A95= 7°), which is in good agreement with the Late Permian/Early Triassic group of poles from Australia.
The Early Permian poles in the present study, and other recent data from Australia, have implications for the configuration of Pangaea after its formation by collision of Laurussia and Gondwana in the Late Carboniferous. Morel & Irving (1981) referred to four different reconstructions as Pangaea A1, A2, B and C. It has long been recognized that Pangaea A1-type reconstructions (e.g. Bullard 1965), which accurately represent Pangaea immediately before its breakup in the Jurassic, produce a systematic mismatch between palaeopoles from Laurussia and Gondwana in the Permian. A number of workers (e.g. Muttoni 1996; Torcq 1997) have argued recently for Pangaea B configurations in the Permian, which evolved to a Pangaea A2 configuration in the Late Permian or Triassic by ∼3500 km of dextral strike-slip between Laurussia and Gondwana. Other researchers argue against this scenario on geological grounds (e.g. Smith & Livermore 1991).
Van der Voo (1993) and McElhinny & McFadden (2000) give extensive discussions of the alternative models and possible resolutions of the controversy. These authors favour Pangaea A2 models on geological grounds and note that the discordance between APWPs is substantially reduced if available East Gondwana poles, which were mostly of low quality when those compilations were made, are eliminated from consideration. Residual mismatches may reflect a combination of data quality problems with West Gondwana poles, which produces rather imprecise mean poles, reconstruction problems within Gondwana, and possibly some contribution from a persistent non-dipole component of the palaeofield.
The substantial improvement in the quality of the newer Australian data justifies a reassessment of this issue. In particular, the 275 Ma mean pole for Australia (McElhinny & McFadden 2000), based on older data, has a large A95 (23°), whereas the two 285 Ma poles reported here have much smaller errors. It is evident from Fig. 14 that the Late Palaeozoic—Jurassic APWP of Australia shows much more structure than is resolved by mean poles from Africa and South America. The Early Jurassic pole positions from all major Gondwana continents are in reasonable agreement in a Lottes & Rowley (1990) reconstruction. The APWPs of the major constituents of West Gondwana appear to indicate a gradual movement from the Permo-Carboniferous pole position to this Jurassic position, with a quasistatic interval in the Triassic—Jurassic.
There is no sign of the early Triassic excursion of the APWP into SE Australia in the trend defined by the mean poles from Africa and South America, although the above-mentioned individual high-quality pole from the Tanzanian Redbeds (Nyblade 1993), supports this part of the track. Comparison of the APWPs suggest that the mean poles from South America and Africa are biased by remagnetizations in the Mesozoic. Many putative Permian and Triassic African pole positions are consistent with Early Jurassic remagnetization, probably associated with the massive Karoo magmatism. Penecontemporaneous magmatism occurred through Antarctica and continued into Tasmania (producing the Ferrar Dolerites and Tasmanian Dolerites, respectively), but did not significantly affect the Australian mainland. Pervasive Early Jurassic overprinting, attributable to igneous activity associated with initial opening of the Atlantic Ocean, is also found in NW Africa (e.g. Derder 2001). Similarly, the apparent Permian—Triassic quasistatic interval for South America may be an artefact of widespread remagnetization in the Jurassic—Cretaceous, probably associated with episodes of igneous activity that preceded breakup of West Gondwana. Comparison of mean poles from West Gondwana with contemporaneous poles from Laurussia, in a Pangaea reconstruction (e.g. McElhinny & McFadden 2000, Fig. 7.9) shows systematic offsets along the APWPs that strongly suggest the West Gondwana pole positions, particularly in the Triassic, are biased towards younger apparent ages by Jurassic to Early Cretaceous overprinting. A number of Late Palaeozoic to Triassic South American poles may also be affected by local block rotations that have the effect of moving the poles to apparently younger positions (Re & Rapalini 1997; S. Geuna, pers. comm., 2002).
Jurassic magmatism is volumetrically minor in mainland Australia and there is no evidence for regional Jurassic remagnetization. In fact, the substantial APW recorded in Australia throughout the Late Palaeozoic, Mesozoic and Tertiary argues strongly against any pervasive remagnetization event. Widespread partial overprinting of older rocks in the Late Cretaceous (ca. 90 Ma), immediately preceding opening of the Tasman Sea, is well recognized along the eastern seaboard of Australia, but these relatively low-grade overprints are easily recognizable and readily removed by thermal demagnetization (Schmidt & Embleton 1981; Dunlop 1997).
Given that the West Gondwana continents are substantially affected by remagnetization during or after the Jurassic, the Late Palaeozoic—Early Mesozoic APWP for Gondwana may be better defined by Australian data than by data from West Gondwana. For Permo-Carboniferous rocks in West Gondwana, primary magnetization directions differ sufficiently from the Mesozoic directions that Mesozoic overprinting is more readily recognized. The consistently reversed geomagnetic polarity in the PCRS also assists discrimination between Permo-Carboniferous and younger magnetizations. These points may explain the fact that the Permo-Carboniferous poles from West Gondwana appear to be much less biased by overprints, and agree better with the Australian data. The fact that the 305 Ma mean poles for Africa and South America have substantially higher precisions than the corresponding Permian—Triassic mean poles (McElhinny & McFadden 2000, Table 6.13) also supports the proposition that the Permo-Carboniferous data are less contaminated by overprints.
The well-defined Late Carboniferous—Early Permian poles from Australia are incompatible with configurations similar to Pangaea A2 that incorporate standard Gondwana reconstructions. However, the systematic offset between precisely defined Australian and West Gondwana (African and South American) Permo-Carboniferous poles (ca. 305 Ma) that can be seen in Fig. 14 confirms that the discordance between the APWPs of West and East Gondwana is now significantly greater than the uncertainties in the data. Thus it appears that the Gondwana reconstruction may require amendment to a tighter fit. There is evidence that the extent of attenuation of continental margins during breakup may have been underestimated hitherto. Tikku & Cande (1999) have reinterpreted seafloor spreading magnetic anomaly data between Antarctica and Australia to infer considerable continental extension and/or deformation of oceanic crust in the Australia—Antarctic Basin during the earliest period of seafloor spreading between these continents. Neglecting this extension produces up to ∼5° of continental overlap in reconstructions. Ambiguous relationships between East and West Antarctica and uncertainties concerning the position of India within East Gondwana may also permit some modification of the standard reconstructions between East and West Gondwana to produce a tighter overall fit.
If extension of continental margins during breakup is a general phenomenon, as seems likely, and if it is of sufficient magnitude to explain the discordance between Permo-Carboniferous palaeopoles of East and West Gondwana, by analogy the Pangaea controversy might be partly resolvable with a slightly tighter Pangaea A2-type fit. Independent geological evidence of the extent of stretching of passive margins that has occurred during breakup is required to test this explanation. Fig. 15 illustrates the extent of the problem in the Early Permian. The MLIC and TIC poles lie about 15° NE of the corresponding age mean pole for Laurussia (McElhinny & McFadden 2000), rotated to Australia using the Euler poles of Lottes & Rowley (1990). A substantial modification of the fit of Pangaea is required to reconcile the Early Permian Laurussian pole with the Australian poles.

Comparison of Late Carboniferous—Early Jurassic APWPs for Australia and Laurussia (rotated into Australian coordinates according to Euler poles of Lottes & Rowley 1990). Ages (in Myr) are annotated adjacent to poles and A95 circles are indicated. The Laurussian APWP is defined by mean poles calculated at 20 Myr intervals from 300 to 180 Ma (McElhinny & McFadden 2000). The Australian poles are: 180 Ma (compilation pole of Embleton 1981); 195 Ma mean pole of McElhinny & McFadden (2000); 240 Ma (Patonga Claystone, Embleton & McDonnell 1981); 285 Ma (MLIC and TIC poles—this study); and 305 Ma mean pole (McElhinny & McFadden 2000).
Although Pangaea B-type reconstructions can bring the Early Permian poles from Laurussia and West Gondwana into acceptable agreement, it is clear from Fig. 15 that the Early Triassic (240 Ma) poles for Australia and Laurussia, although somewhat closer in such a reconstruction, cannot be reconciled by a Pangaea B configuration. Attempts to remove the discordancy of the Early Triassic poles by invoking large-scale, complex relative motions between Laurussia and Gondwana in the Permian and Triassic would cause insuperable geological difficulties. Thus we conclude that small, but persistent, departures of the palaeofield from the axial geocentric dipole model occurred in the Early Triassic. It is then plausible that the lesser, but still significant, discordance of the Early Permian poles in an A2-type reconstruction is also attributable to non-dipole effects, rather than requiring a Pangaea B-type configuration. We suggest that the most parsimonious interpretation of the geological and palaeomagnetic data retains a Pangaea A2-type reconstruction through the Permian and Triassic, with systematic offsets between APWPs for Laurussia and West Gondwana explained predominantly by non-dipole effects. It is possible that a tighter fit of Laurussia against West Gondwana may also reduce the discordance of the APWPs, although this effect cannot fully explain the mismatch.
Van der Voo & Torsvik (2001) have recently presented an analysis of palaeomagnetically estimated latitudes versus palaeogeographically predicted palaeolatitudes for poles from Laurussia. Their results suggest that the average octupole field was about 10 per cent of the dipole field in the 200–300 Ma interval, which is sufficient to account for typical misfits of palaeomagnetic poles from different Pangaean continents in Pangaea A2-type reconstructions. However, the larger-than-average discrepancy between the Laurussian and Australian poles in the Early Triassic suggest that there was a greater contribution from non-dipole fields at this time.
The method of Van der Voo & Torsvik (2001) assumes that there is no long-term contribution from the quadrupole field, based on concordance between palaeomagnetic and palaeoclimatic equators in the Fundy Basin, Canada, in the Late Triassic (Kent & Olsen 2000). Provided only that the time-averaged palaeofield was zonal, the Early Triassic palaeomagnetic data from Australia provide an important palaeogeographic constraint that the south pole was within, or very close to, SE Australia around 240 Ma, irrespective of substantial quadrupole, octupole, or higher-order multipole contributions to the geomagnetic field.
Another effect, which could produce substantial systematic errors in palaeopoles, is inclination error for depositional and post-depositional remanent magnetizations carried by sedimentary rocks, particularly terrestrial sediments. Inclination shallowing in such rocks produces systematically lower apparent palaeolatitudes, thereby mimicking the effects of a persistent positive octupole contribution to the palaeofield. The error in estimated palaeolatitude is greatest at mid-latitudes and can be as large as 20 °. Rochette & Vandamme (2001) note that Permo-Triassic poles from Laurussia come from low-latitude northern hemisphere sites, whereas the West Gondwana Permo-Triassic data come predominantly from mid-southern latitudes, and that both data sets are dominated by sedimentary rocks. For earlier and later times the poles are derived mainly from rocks (marine sediments and volcanics) that exhibit little or no inclination error. They suggest that inclination errors could account for much of the discordance between Permo-Triassic poles from Laurussia and West Gondwana by apparently shifting both supercontinents towards the equator. The dominant effect is the apparent northward shift of West Gondwana. The resulting overlap that occurs in a Pangaea A-type configuration can only be removed by invoking a Pangaea B-type relative rotation of Laurussia and Gondwana. Quantitatively plausible corrections for inclination error can largely reconcile poles from the two components of Pangaea in an A2-type reconstruction.
The Permo-Triassic palaeopoles from Australia, however, are not subject to inclination error, as they come from igneous as well as sedimentary rocks and the inclinations are very steep (>85°). Systematic shallowing of directions recorded by sites in Africa and South America that were in mid-latitudes of the southern hemisphere would produce south palaeopoles that are apparently too far from their corresponding sites. Thus corrections for inclination errors will move the Permo-Triassic palaeopoles closer to their sites and increase their discordance with coeval Australian poles. The same problem applies to purely octupole contributions to the non-dipole field. A 10–20 per cent octupole component, as suggested by Van der Voo & Torsvik (2001), can reconcile the palaeopoles from Laurussia and West Gondwana with a Pangaea A2 configuration for Late Carboniferous and Permo-Triassic times, but cannot resolve the discordance of the Early Triassic Australian poles with coeval poles from West Gondwana. A more complex configuration of the time-averaged field at this time, such as a substantial quadrupole component, in addition to an octupole, may be required to ameliorate this problem. The available evidence suggests that any such quadrupole field had decreased to negligible levels by the end of the Triassic (Kent & Olsen 2000).
We note in passing that the problems with reconciling APWPs from various components of Pangaea offer no comfort to proponents of an expanding Earth (e.g. Carey 1976), as the requirement of a tighter fit to bring the APWPs into agreement is in the wrong sense for the expanding Earth hypothesis.
Conclusions


The palaeomagnetic poles from the MLIC and the TIC are indistinguishable and support evidence from radiometric dating and geochemical affinities that these complexes are coeval. Based on these results and other recent studies, the Early Permian pole for Gondwana lies within East Antarctica, significantly closer to Australia than can be accommodated in Pangaea A1 or A2 reconstructions that incorporate conventional fits of the Gondwana continents. The new Early Permian poles are apparently more consistent with Pangaea B-type reconstructions of Gondwana and Laurussia than with the Pangaea A2 configuration, if non-dipole fields or other sources of systematic error can be neglected.
With the greater precision and age control provided by the new Early Permian poles, supplemented by other high-quality data, it is now apparent that there is a small, but statistically significant, discrepancy between Permo-Carboniferous poles from East Gondwana (as defined by Australian data) and West Gondwana (African and South American poles). This suggests that there are problems with existing Gondwana reconstructions, or with data quality. We suggest that good quality data for the Carboniferous to Jurassic from Australia are now sufficiently abundant that the Australian APWP, including some detailed structure, is well determined for this interval. A careful re-examination of the problematic data from West Gondwana is necessary to eliminate from consideration poles that are associated with remagnetized or poorly dated rock units and poles that come from areas with uncertain structural corrections.
After eliminating dubious data from West Gondwana and retaining only the most reliable poles, reconciliation of data from the two halves of Gondwana requires a tighter fit of the continents that comprise Gondwana. More accurate reconstructions that take into account stretching of continental margins during breakup of the supercontinent may partly resolve this problem. Some modifications of the fit between East and West Gondwana that reduce the discrepancy between their respective APWPs may be required. If, as seems likely, attenuation of continental margins is a general occurrence during breakup, this may also help to explain the long-standing controversy over Pangaea reconstructions. Pangaea A2 reconstructions, which are generally favoured on geological grounds, if modified to allow for stretching of the continental margins to give a tighter fit, might bring the Permo-Carboniferous APWPs of Laurussia and Gondwana into somewhat better agreement. Independent estimates of the extent of attenuation of the passive margins are required to assess whether this explanation suffices to reconcile the APWPs of the two supercontinents for the Permo-Carboniferous.
Although alternative Pangaea reconstructions, such as Pangaea B, may reconcile poles from Laurussia with Australian poles in the Late Carboniferous—Early Permian, no plausible reconstruction can bring the Early Triassic poles into agreement. This suggests that persistent departures from a pure dipole field were present in the Early Triassic. A dipole + octupole field alone cannot reconcile the Australian data with poles from West Gondwana, suggesting that widespread remagnetization of Permian and Triassic rock units in Africa and South America has largely erased the record from West Gondwana of the Triassic loop into SE Australia, or that a more complex paleofield may have been important in the Early Triassic. Provided only that the time-averaged palaeofield was zonal, the Early Triassic palaeomagnetic data from Australia provide an important palaeogeographic constraint that the south geographic pole was within, or very close to, SE Australia around 240 Ma, irrespective of substantial quadrupole, octupole or higher-order multipole contributions to the geomagnetic field. Lesser, but still significant, non-dipole effects may also have been present during the Late Carboniferous and Permian, and may help resolve the Pangaea A versus B controversy, without requiring substantial attenuation of continental margins or intracontinental deformation.
A solution of the Pangaea problem is hampered by the variable quality of the palaeomagnetic data, ambiguities in reconstructions and probable complexity of the palaeofield configuration. With the current state of knowledge, no simple, unambiguous resolution of the apparent paradoxes is possible. We believe, however, that the most parsimonious interpretation of the extant palaeomagnetic and geological information is that Laurussia and Gondwana remained in a Pangaea A2-type configuration through the Permian and Triassic. Discordance between the APWPs for these two supercontinents is attributable mainly to persistent non-dipole components of the geomagnetic field, which were most important in the Early Triassic.
Acknowledgments
Gregg Morrison, Phil Blevin, and Simon Beams have guided the sampling programmes and assisted greatly with their insights into the geological and geochemical aspects of the study areas. Tom Orr and John Kay of Mount Leyshon Mines Ltd have assisted and encouraged the Mount Leyshon study. World Geoscience Corporation supplied the aeromagnetic data over the TIC. This work was partially supported by Macquarie University research grants and ARC funding to ML. Phil Schmidt, Silvana Geuna and GJI reviewers (Mike McElhinny and Zhengxiang Li) suggested improvements to the manuscript. This is contribution 256 from the ARC National Key Centre for Geochemical Evolution and Metallogeny of Continents (GEMOC).
References