-
PDF
- Split View
-
Views
-
Cite
Cite
Dona R Wisidagama, Carl S Thummel, Regulation of Drosophila Intestinal Stem Cell Proliferation by Enterocyte Mitochondrial Pyruvate Metabolism, G3 Genes|Genomes|Genetics, Volume 9, Issue 11, 1 November 2019, Pages 3623–3630, https://doi.org/10.1534/g3.119.400633
- Share Icon Share
Abstract
Multiple signaling pathways in the adult Drosophila enterocyte sense cellular damage or stress and signal to intestinal stem cells (ISCs) to undergo proliferation and differentiation, thereby maintaining intestinal homeostasis. Here we show that misregulation of mitochondrial pyruvate metabolism in enterocytes can stimulate ISC proliferation and differentiation. Our studies focus on the Mitochondrial Pyruvate Carrier (MPC), which is an evolutionarily-conserved protein complex that resides in the inner mitochondrial membrane and transports cytoplasmic pyruvate into the mitochondrial matrix. Loss of MPC function in enterocytes induces Unpaired cytokine expression, which activates the JAK/STAT pathway in ISCs, promoting their proliferation. Upd3 and JNK signaling are required in enterocytes for ISC proliferation, indicating that this reflects a canonical non-cell autonomous damage response. Disruption of lactate dehydrogenase in enterocytes has no effect on ISC proliferation but it suppresses the proliferative response to a loss of enterocyte MPC function, suggesting that lactate contributes to this pathway. These studies define an important role for cellular pyruvate metabolism in differentiated enterocytes to maintain stem cell proliferation rates.
Like most animals, the intestine of the fruit fly Drosophila is a self-renewing tissue in which a population of stem cells generates new differentiated cell types to replace those that are damaged and lost during normal life (Micchelli and Perrimon 2006; Ohlstein and Spradling 2006; Jiang et al. 2009; Lemaitre and Miguel-Aliaga 2013). The intestinal stem cells (ISCs) reside in the basal region of the intestine, adjacent to the visceral muscle, and undergo regulated division to maintain tissue homeostasis with complete replacement of the intestinal epithelium every few weeks under normal conditions. Dividing ISCs give rise to a new stem cell through the process of stem cell self-renewal as well as an enteroblast (EB) or secretory enteroendocrine (EE) cell. The EB, in turn, differentiates without cell division into an absorptive enterocyte (EC). The EEs and ECs, with a predominance of ECs, constitute the single cell layer of the intestinal epithelium, with an apical brush border that faces the lumen. This system of regulated stem cell self-renewal and differentiation is conserved through animal evolution, establishing Drosophila as an ideal genetic model to define the molecular mechanisms that maintain intestinal physiology and function.
Detailed studies over the past ten years have characterized signaling pathways in the Drosophila intestine that maintain proper levels of stem cell proliferation, self-renewal, and differentiation (Bonfini et al. 2016; Guo et al. 2016; Jiang et al. 2016; Miguel-Aliaga et al. 2018). These include a stress response pathway in ECs that senses cellular injury or infection and signals to ISCs to promote their proliferation. Acting in part through JNK signaling, this pathway results in the secretion of cytokines of the Unpaired family that activate canonical JAK/STAT signaling in ISCs (Buchon et al. 2009a; Jiang et al. 2009; Beebe et al. 2010; Lin et al. 2010). Genetic studies have demonstrated that this response in ISCs is dependent on the Domeless receptor and results in the downstream induction of the canonical JAK/STAT target gene Socs36E. The Sox21a transcription factor is also up-regulated by JAK/STAT signaling in ISCs and promotes their proliferation and differentiation (Meng and Biteau 2015; Zhai et al. 2015; Zhai et al. 2017). These signaling interactions, along with inputs from multiple other pathways, act together to maintain intestinal physiology and function in the adult fly.
Although multiple forms of stress, infection, and damage have been investigated as signals that trigger stem cell proliferation and differentiation, less is known about how changes in cellular metabolism might affect these pathways. Most of these studies have investigated how dietary nutrients can impact intestinal homeostasis. For example, dietary methionine or S-adenosylmethionine synthesis regulates protein synthesis in ISCs and Upd3 cytokine signaling from ECs to support stem cell division in fed animals (Obata et al. 2018). Similarly, dietary lipids acting through the Notch signaling pathway regulate EE cell number in the posterior midgut (Obniski et al. 2018), and the hexosamine biosynthetic pathway facilitates the proliferative effect of the insulin signaling pathway on ISCs (Mattila et al. 2018).
Our studies focus on genetic manipulation of the Mitochondrial Pyruvate Carrier (MPC) as a means of modulating mitochondrial pyruvate metabolism in a cell type-specific manner. The MPC is an evolutionarily-conserved obligate heterodimer of two proteins, MPC1 and MPC2, that resides in the inner mitochondrial membrane and transports pyruvate into the mitochondrial matrix (Bricker et al. 2012; Herzig et al. 2012). The MPC thus provides a key metabolic step that links cytoplasmic glycolysis with mitochondrial oxidative metabolism. Accordingly, a loss of the MPC results in reduced mitochondrial activity while MPC overexpression leads to increased pyruvate flux into mitochondria and increased oxidative metabolism (Bricker et al. 2012; Schell et al. 2014). These functions for the MPC place it in a critical position to modulate rates of aerobic glycolysis with downstream effects on cell proliferation. Consistent with this, our past studies have shown that the MPC is both necessary and sufficient in a stem cell autonomous manner to regulate ISC proliferation and maintain intestinal homeostasis in Drosophila (Schell et al. 2017).
Here we extend our earlier studies of the MPC in the Drosophila intestine by addressing roles for cellular pyruvate metabolism in differentiated ECs. We noticed that although a clonal loss of the MPC in ISCs resulted in increased proliferation it did not block their differentiation, leading to the formation of MPC mutant enterocytes. This led us to examine the possibility that a loss of the MPC in differentiated intestinal cells might have a non-autonomous effect on wild-type stem cell proliferation. Here we show that cell-specific loss of the MPC in ECs acts through the JNK and JAK/STAT signaling pathways to activate ISC proliferation in a non-autonomous manner. These studies establish a role for mitochondrial pyruvate metabolism in ECs to regulate ISC proliferation rates.
Materials and Methods
Fly strains
Drosophila stocks were maintained on standard food containing 3% sucrose, 6% glucose, 8% yeast, and 1% agar in a 25° incubator. For dMPC1 genetic studies, control flies were homozygous for a precise excision of the P(XP)CG14290[d00809] P-element insertion and mutant flies were transheterozygous for the two deletion alleles, dMPC11 and dMPC12, as described (Bricker et al. 2012). Transgenic lines are as follows: UAS-dMPC1-RNAi (Bricker et al. 2012), UAS-PDH-E1-RNAi (NIG 7010R-3), UAS-PDH-E2-RNAi (NIG 5261R-3), UAS-LDH-RNAi (Bloomington 33640), UAS-BskDN (Bloomington 6409), UAS-upd3-RNAi (Bloomington 28575), upd3.1-lacZ (Jiang et al. 2011), and 10XSTAT92E-DGFP (Bach et al. 2007). The UAS-LDH-RNAi transgenic line has been used previously for functional studies of this enzyme (Slaninova et al. 2016; Charlton-Perkins et al. 2017). Tub-GAL80ts was used to restrict RNAi to the adult stage in all experiments. The 10XSTAT92E-DGFP and w; myo1A-GAL4/CyO; Tub-GAL80ts, UAS-GFP/TM6B stocks were a gift from B. Edgar.
Histology and immunostaining
Intestines were dissected in 1xPBS and fixed with 4% formaldehyde (Polysciences Inc, EM grade) overnight at 4°. Tissues were washed four times with 0.1% Triton, 1xPBS (PBST) and incubated with appropriate primary antibodies at 4° overnight. Antibodies used: mouse anti-beta galactosidase (DSHB 40-1a) diluted 1:100 in PBST, chicken anti-GFP (Abcam ab13970) diluted 1:10,000, and rabbit anti-phospho-histone H3 (pHH3) diluted 1:1000 (Millipore 06-570). The tissues were then washed and incubated for 3-4 hr with the following secondary antibodies: donkey anti-mouse Cy3-conjugated antibodies (Jackson 715-165-157), donkey anti-rabbit Cy3-conjugated antibodies (Jackson 715-165-152), or goat anti-chicken Alexa-488 antibodies (Abcam ab150169). Samples were mounted using Vectashield (Vector, USA) with DAPI. Images were acquired using an Olympus FV1000 confocal microscope and assembled into Z stack projections for the figures.
RNA-seq analysis
Adult myo1A > mCherry-RNAi animals (controls) and myo1A > dMPC1-RNAi animals, both with Tub-GAL80ts to restrict RNAi to the adult stage, were aged for 7 days. Four biological replicates of 15 intestines per genotype were dissected in 1x cold PBS and immediately transferred to Trizol on ice. RNA was extracted as described (Zymo Research RNA extraction kit R2051) and RNA quality was analyzed using a Agilent RNA ScreenTape assay. Total RNA from each biological replicate was subjected to Illumina HighSeq2000 50-cycle single-read sequencing. Standard replicate RNA-seq analysis was performed using USeq and DESeq analysis packages with alignment to the D. melanogaster dm3 genome assembly. Transcripts displaying a log2-fold change ≥0.6 in mRNA abundance and FDR ≤1% were considered as differentially expressed genes. RNA quality control, library preparation, sequencing, and data analysis were performed at the University of Utah High Throughput Genomics and Bioinformatics Core Facilities.
GC-MS analysis
Control and dMPC1 mutant animals were raised until 7 days of adulthood. Twenty females per sample were snap-frozen in liquid nitrogen and prepared for analysis by gas chromatography/mass spectrometry (GC/MS) as described (Tennessen et al. 2014). Data are presented from four independent experiments, each consisting 20 animals per condition. Sample preparation and GC/MS analysis were performed by the Metabolomics Core Research Facility at the University of Utah School of Medicine.
N-acetyl cysteine feeding
Five day old adults were transferred to vials supplemented with 0.1% NAC (Sigma A7250) and shifted to 29°. Animals were maintained on this NAC supplemented diet for 7 days after which the intestines were dissected and subjected to antibody stains to detect pHH3.
Statistical analysis
GraphPad PRISM 6 software was used to plot data and perform statistical analysis. Pairwise comparison p-values were calculated using a two-tailed Student’s t-test. Multiple comparison P values were calculated using one-way ANOVA with Sidak’s multiple test correction. Error bars depict the mean ± SEM.
Data availability
A full list of all RNAi lines screened can be found above with the corresponding stock numbers. All reagents are also cited with regard to catalog number and source. RNA-seq data from this study can be accessed at NCBI GEO (accession number: GSE136211). Supplemental material available at FigShare: https://doi.org/10.25387/g3.9621263.
Results and Discussion
Mitochondrial pyruvate metabolism in enterocytes regulates stem cell proliferation
Previously we demonstrated that loss of MPC function in ISCs enhances their proliferation in a cell autonomous manner (Schell et al. 2017). During these studies we noticed that GFP-marked dMPC1 mutant MARCM clones included mutant stem cells that differentiated into mature ECs or EEs. This observation raised the possibility that mutant differentiated cells might signal to adjacent wild-type ISCs, inducing their proliferation in a non-cell autonomous manner. To test this hypothesis, we targeted dMPC1 RNAi specifically to ECs using the myo1A-GAL4 driver and measured ISC proliferation by staining dissected intestines for phosphorylated histone H3 (pHH3) (Figure 1A, Supplementary Figure 1A). RNAi was temporally restricted to the adult stage by using Tub-GAL80ts (McGuire et al. 2004). Interestingly, this experiment revealed a major increase in ISC proliferation, more than twofold higher than we had seen with ISC-specific Dl > dMPC1 RNAi under similar conditions (Figure 1A, Supplementary Figure 1A) (Schell et al. 2017). This observation supports the conclusion that a loss of mitochondrial pyruvate metabolism in differentiated ECs can impact ISC proliferation rates.
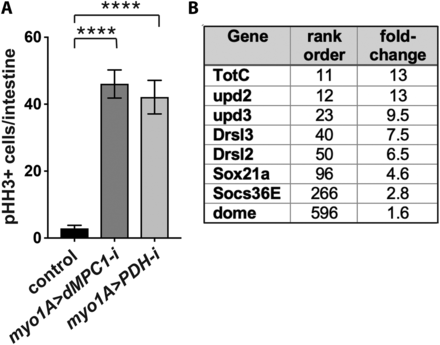
Reduced mitochondrial pyruvate metabolism in enterocytes increases stem cell proliferation in a non-autonomous manner. (A) The EC-specific myo1A-GAL4 driver was used to target RNAi for mCherry (control), dMPC1, or PDH-E1 in the presence of Tub-GAL80ts. The number of cells staining positive for phosphorylated histone H3 (pHH3+ cells) was quantified per intestine after shifting 4-5 day old animals to 29°C for seven days. Data are plotted as the mean ± SEM, n ≥ 20 animals for each condition, ****P ≤ 0.0001. (B) Genes that encode key components in the JAK/STAT signaling pathway increase their expression in myo1A > dMPC1-RNAi intestines. Data from Supplementary Table 1 is depicted for genes involved in JAK/STAT signaling in the intestine, showing their rank order in the RNA-seq dataset and fold-change increase in expression relative to the control.
To confirm that this response is due to changes in EC pyruvate metabolism, we conducted a similar experiment using myo1A-GAL4 to target RNAi for a gene encoding part of the pyruvate dehydrogenase (PDH) complex. PDH acts downstream from the MPC in the mitochondrial matrix where it catalyzes the conversion of pyruvate into acetyl-CoA, which is the initial metabolite in the tricarboxylic acid (TCA) cycle. Quantification of pHH3-staining cells in myo1A > PDH RNAi intestines revealed a major increase in ISC proliferation, similar to that seen with dMPC1 RNAi (Figure 1A). Taken together, these results indicate that disruption of pyruvate metabolism in ECs is sufficient to induce ISC proliferation in a non-cell autonomous manner.
To gain insights into the molecular mechanisms by which ECs might be signaling to ISCs, we conducted genome-wide RNA sequencing analysis using dissected intestines from dMPC1 mutants and myo1A > dMPC1-RNAi animals (Supplementary Table 1). Interestingly, the myo1A > dMPC1-RNAi dataset included increased expression of multiple canonical markers of the JAK/STAT pathway (Figure 1B). This included the inflammatory cytokine genes upd2 and upd3 among the top most affected genes, as well as the inflammatory-responsive Drosomycin genes Drsl2 and Drsl3, the gene encoding the Domeless cytokine receptor, and the canonical JAK/STAT target genes Sox21a and Socs36E (Figure 1B). This response is consistent with the well-established signaling pathway by which stressed or damaged ECs signal to ISCs to promote their proliferation and differentiation (Buchon et al. 2009a; Buchon et al. 2009b; Jiang et al. 2009; Beebe et al. 2010; Lin et al. 2010).
Enterocytes require Upd3 and JNK signaling to indirectly control stem cell proliferation
If ECs initiate cytokine signaling in response to a loss of the MPC then we would expect to see increased upd3 expression in these cells accompanied by elevated JAK/STAT signaling and proliferation in ISCs. To test this possibility, we used myo1A-GAL4 to target dMPC1 RNAi in ECs in the presence of the upd3.1-lacZ and 10XSTAT92E-DGFP reporters (Bach et al. 2007; Jiang et al. 2011). As expected, myo1A > dMPC1 RNAi resulted in increased ISC proliferation relative to controls as detected by pHH3 antibody staining (Figure 2A-D, red nuclei). In addition, ECs displayed varying levels of elevated upd3.1-lacZ expression. Moreover, we could detect cells adjacent to these ECs that display high levels of 10XSTAT92E-DGFP expression. Although we did not determine the identity of these GFP-expressing cells, their small size and triangular shape suggest that they represent ISCs, pre-ECs, or EBs (Figure 2D). Taken together, these observations are consistent with canonical cytokine signaling from ECs to ISCs, activating the JAK/STAT pathway and promoting ISC proliferation.
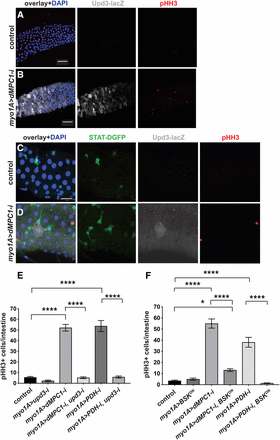
MPC loss of function in enterocytes requires UPD3 and JNK to indirectly control stem cell proliferation. The myo1A-GAL4 driver was used to target RNAi for mCherry (control) (A,C) or dMPC1 (B,D) in the presence of Tub-GAL80ts. The upd3.1-lacZ reporter (gray, A-D) and 10xSTAT92E-DGFP reporter (green, C,D) were used to detect Upd3 expression and JAK/STAT activation, respectively, by immunohistochemistry. Proliferating ISCs are marked by staining for pHH3+ cells (red) and nuclei are stained with DAPI (blue). Panels 2A,B and 2C,D are from independent experiments and depict the R4 region of the intestine. Scale bars represent 50 µm (A,B) and 20 µm (C,D). (E) The myo1A-GAL4 driver was used to target RNAi for mCherry (control), dMPC1, PDH-E1, or upd3, either alone or in combination as shown, in the presence of Tub-GAL80ts. The number of cells staining positive for phosphorylated histone H3 (pHH3+ cells) was quantified per intestine after shifting 4-5 day old animals to 29°C for seven days. Data are plotted as the mean ± SEM, n ≥ 20 animals for each condition. (F) The myo1A-GAL4 driver was used to target mCherry RNAi (control), dMPC1 RNAi, PDH-E2 RNAi, or BskDN expression, either alone or in combination as shown, in the presence of Tub-GAL80ts. The number of cells staining positive for phosphorylated histone H3 (pHH3+ cells) was quantified per intestine after shifting 4-5 day old animals to 29°C for seven days. Data are plotted as the mean ± SEM, n ≥ 20 animals for each condition. ****P ≤ 0.001, *P ≤ 0.05.
Genetic studies have demonstrated that upd3 is the main cytokine that controls JAK/STAT signaling in the intestine (Osman et al. 2012). We therefore set out to determine if upd3 is required in ECs for the effect on ISC proliferation. As expected, myo1A > dMPC1 RNAi resulted in a dramatic increase in proliferating cells in the intestine (Figure 2E) and this response was unaffected by including nonspecific mCherry RNAi in the genetic background (Supplementary Figure 1B). EC-specific myo1A > upd3 RNAi alone had no effect on proliferation rates relative to the control (Figure 2E). However, the elevated proliferation seen with myo1A > dMPC1 RNAi was eliminated by including upd3 RNAi in the genetic background (Figure 2E). A similar effect was seen when myo1A-GAL4 was used to drive PDH RNAi and upd3 RNAi in ECs (Figure 2E). Taken together these observations support the model that a loss of mitochondrial pyruvate metabolism in the ECs acts through Upd3 cytokine signaling to activate stem cell proliferation.
The JNK pathway is activated by cellular damage and stress in ECs and required for Upd3 cytokine expression (Buchon et al. 2009a; Jiang et al. 2009). We therefore asked if the non-cell autonomous control of stem cell proliferation caused by changes in EC pyruvate metabolism is dependent on JNK signaling. For this purpose, we used myo1A-GAL4 to drive the expression of a dominant-negative form of JNK using the UAS-BskDN construct in either the presence or absence of UAS-dMPC1-RNAi or UAS-PDH-RNAi. EC-specific expression of BskDN had no effect on the basal rate of ISC proliferation (Figure 2F). In contrast, combining this expression with either dMPC1 RNAi or PDH RNAi resulted in a major reduction in ISC proliferation (Figure 2F). These observations indicate that JNK signaling is required in ECs for the non-cell autonomous effect of EC mitochondrial pyruvate metabolism on ISC proliferation.
dMPC1 mutants have elevated levels of lactate
Although a number of studies have addressed how stress signaling pathways in ECs can promote ISC proliferation, much less is known about how metabolic changes might impact this response. One possibility is that the mitochondrial dysfunction resulting from reduced levels of pyruvate oxidation might lead to an increase in oxidative stress. However, feeding the dietary antioxidant N-acetylcysteine (NAC) to animals with EC-specific dMPC1 RNAi had no effect on the rate of ISC proliferation (Supplementary Figure 1C). A similar treatment of dSdhaf3 mutants in our lab was sufficient to rescue the oxidative stress caused by a loss of Succinate Dehydrogenase activity (Na et al. 2014). Although further studies are needed to definitively conclude that oxidative stress is not contributing to the non-autonomous effect of EC metabolism on ISC proliferation, this result provides support for this possibility.
An alternative model is that changes in the level of one or more metabolite in MPC mutant ECs might provide a signal that is sensed by the stress response pathway. Although we attempted to perform untargeted GC/MS metabolomic analysis on dissected Drosophila intestines we were unable to generate reproducible data using this approach. Accordingly, we used GC/MS to profile basic metabolites in extracts from whole control animals and dMPC1 mutants. We used mature adult females maintained on a diet containing 8% yeast 3% sucrose and 6% glucose for seven days in order to reproduce the experimental conditions used for the functional studies in this paper. As expected, the loss of MPC function leads to a backup in glycolytic intermediates, including elevated levels of glucose, sorbitol, pyruvate, and lactate (Figure 3). This is accompanied by reduced levels of TCA cycle intermediates along with reduced proline, which is a major amino acid for anaplerotic input into the TCA cycle in insects (Teulier et al. 2016). These results are consistent with our previous observation that dMPC1 mutants are hyperglycemic and display shifts in the levels of metabolic intermediates that are consistent with reduced mitochondrial pyruvate import (Bricker et al. 2012; McCommis et al. 2016).

dMPC1 mutants have increased glycolytic intermediates and reduced TCA cycle intermediates. GC/MS metabolomic profiling of controls (blue bars) and dMPC1 mutants (red bars) on a diet containing 9% sugar and 8% yeast. Data are graphically represented as a box plot, with the box representing the lower and upper quartiles, the horizontal line representing the median, and the bars representing the minimum and maximum data points. Mutant values are normalized to the control and fold changes in metabolite levels are displayed. n = 4 independent experimental collections. ****P ≤ 0.0001, ***P ≤ 0.001, **P ≤ 0.01 *P ≤ 0.05.
LDH is required in enterocytes for non-cell autonomous effects on stem cell proliferation
We noted from our metabolomic analysis that lactate is increased ∼threefold in dMPC1 mutants compared to controls (Figure 3). Clearly, whole animal metabolomic analysis might not reflect metabolic changes that take place in specific intestinal cell types. Nonetheless, this provides a starting point to address how a shift in cellular metabolite levels due to a loss of the MPC might lead to a stress response in ECs. In addition, lactate production by LDH plays an important role in mediating the effects of hexosamine biosynthesis on ISC proliferation in the Drosophila intestine (Mattila et al. 2018). Moreover, changes in LDH activity could affect NAD levels and thus indirectly impact cellular redox state, which has been shown to regulate intestinal JNK signaling and ISC proliferation (Hochmuth et al. 2011; Wang et al. 2014). We thus set out to determine if LDH might be required for the increased expression of Upd3 in ECs lacking the MPC. We used myo1A-GAL4 to direct LDH RNAi in ECs in the presence of the upd3.1-lacZ reporter. Under these conditions we saw no detectable Upd3 expression, similar to controls (Figure 4A,D). Adding dMPC1 RNAi to this genetic background, however, revealed that the enhanced expression of Upd3 seen upon a loss of the MPC in ECs is suppressed by including LDH RNAi (Figure 4B,E). A similar effect was observed when myo1A-GAL4 was used to drive PDH RNAi in the presence or absence of LDH RNAi (Figure 4C,F). Importantly, we also saw a non-cell autonomous effect on ISC proliferation as measured by staining for pHH3 (Figure 4A-F). Quantitation of this effect revealed that the increased ISC proliferation seen upon dMPC1 or PDH RNAi in ECs is effectively suppressed by including LDH RNAi in these genetic backgrounds (Figure 4G). Taken together, these observations suggest that lactate production is required in ECs for the elevated expression of the Upd3 cytokine in response to reduced mitochondrial pyruvate metabolism.
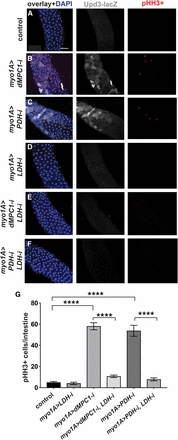
LDH is required in enterocytes for the non-autonomous effect of MPC loss on stem cell proliferation. (A-F) The myo1A-GAL4 driver was used to target RNAi for mCherry (A), dMPC1 (B,E), PDH-E1 (C,F), and LDH (D-F) in the presence of Tub-GAL80ts. The upd3.1-lacZ reporter (gray) was used to detect Upd3 expression, proliferating ISCs are marked by staining for pHH3+ cells (red), and nuclei are stained with DAPI (blue). Images depict the R4 region of the intestine. Scale bar represents 50 µm. (G) The myo1A-GAL4 driver was used to target RNAi for mCherry (control), LDH, dMPC1, or PDH-E1 in the presence of Tub-GAL80ts. The number of cells staining positive for phosphorylated histone H3 (pHH3+ cells) was quantified per intestine after shifting 4-5 day old animals to 29°C for seven days. Data are plotted as the mean ± SEM, n ≥ 20 animals for each condition. ****P ≤ 0.0001.
Reduced EC pyruvate metabolism indirectly regulates ISC proliferation
In conclusion, our studies demonstrate that a disruption in mitochondrial pyruvate metabolism in ECs activates JAK/STAT signaling resulting in a non-cell autonomous effect on ISC proliferation. Our work supports the model that elevated lactate levels generated by LDH are an important stress signal in ECs that lack MPC function (Figure 5). We cannot, however, rule out other roles for LDH in these pathways, such as its contributions to NAD production or 2-hydroxyglutarate synthesis (Hochmuth et al. 2011; Wang et al. 2014; Li et al. 2017). This stress acts through the canonical JNK pathway to result in Upd3 cytokine expression that, in turn, can signal to ISCs. This signaling activates the JAK/STAT pathway which promotes stem cell proliferation, resulting in the production of new differentiated intestinal cells that can replace those cells that have mitochondrial dysfunction. These functional interactions parallel those of other studies that have demonstrated a critical role for JNK and JAK/STAT signaling in maintaining intestinal homeostasis under conditions of tissue stress, infection, or damage (Jiang and Edgar 2011; Miguel-Aliaga et al. 2018). Our results are also consistent with prior reports that mitochondrial function is important for intestinal homeostasis in response to stress and aging in Drosophila (Koehler et al. 2017; Deng et al. 2018). This work expands these studies to include a specific role for pyruvate metabolism in ECs and provides a foundation for future efforts aimed at understanding how changes in cellular physiology can modulate intestinal homeostasis and function.
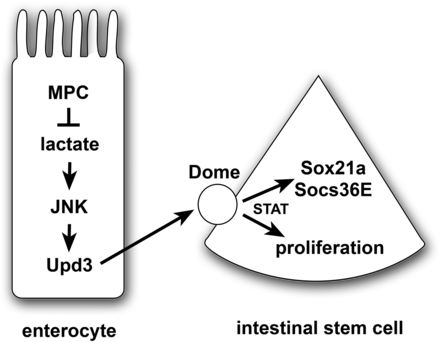
A model for the non-autonomous regulation of ISC proliferation by enterocyte pyruvate metabolism. A loss of the MPC in enterocytes results in increased lactate production by LDH, which activates the JNK stress signaling pathway and Upd3 expression. This secreted cytokine acts through the Dome receptor in ISCs to promote JAK/STAT signaling, resulting in up-regulation of Sox21a and Socs36E as well as stem cell proliferation. Note that this is a speculative model that is intended to summarize the conclusions from this study in the context of the well-established JNK/STAT stress response pathway in the intestine.
Acknowledgments
We thank B. Edgar for providing stocks, G. Lam for technical support, K. Beebe and J. Tennessen for helpful discussions, the Bloomington Stock Center for fly stocks (NIH P40OD018537), and FlyBase for informatic support. RNA-seq analysis was performed by the High-Throughput Genomics and Bioinformatic Analysis Shared Resource at the University of Utah, which is supported by the NCI (P30CA042014). Metabolomics analysis was performed by the Metabolomics Core Facility at the University of Utah which is supported by 1 S10 OD016232-01, 1 S10 OD021505-01 and 1 U54 DK110858-01. The University of Utah has filed a patent related to the Mitochondrial Pyruvate Carrier, of which C.S.T. is a co-inventor. This work was supported by the NIH (1R01CA228346).
Footnotes
Supplemental material available at FigShare: https://doi.org/10.25387/g3.9621263.
Communicating editor: E. Gavis
Literature Cited
Mattila, J., K. Kokki, V. Hietakangas and M. Boutros, 2018 Stem Cell Intrinsic Hexosamine Metabolism Regulates Intestinal Adaptation to Nutrient Content. Dev Cell 47: 112–121 e113.
Obata, F., K. Tsuda-Sakurai, T. Yamazaki, R. Nishio, K. Nishimura et al., 2018 Nutritional Control of Stem Cell Division through S-Adenosylmethionine in Drosophila Intestine. Dev Cell 44: 741–751 e743.
Author notes
Current address: ARUP Laboratories, 500 Chipeta Way, Salt Lake City, UT 84108