-
PDF
- Split View
-
Views
-
Cite
Cite
Miguel Ángel Salinas-García, Jonas Fernbach, Riikka Rinnan, Anders Priemé, Extreme smells—microbial production of volatile organic compounds at the limits of life, FEMS Microbiology Reviews, Volume 49, 2025, fuaf004, https://doi.org/10.1093/femsre/fuaf004
- Share Icon Share
Abstract
Microbial volatile organic compounds (MVOCs) are diverse molecules produced by microorganisms, ranging from mere waste byproducts to important signalling molecules. While the interest in MVOCs has been increasing steadily, there is a significant gap in our knowledge of MVOCs in extreme environments with e.g. extreme temperatures or acidity. Microorganisms in these conditions are subjected to additional stress compared to their counterparts in moderate environments and in many cases have evolved unique adaptations, including the production of specialized MVOCs. This review highlights the diversity of MVOCs identified in extreme environments or produced by isolated extremophiles. Furthermore, we explore potential applications already investigated and discuss broader implications for biotechnology, environmental biology, and astrobiology.
Introduction
Extremophiles are organisms that can sustain growth in extreme environments where at least one factor (e.g. temperature, pH, water activity, nutrient availability, heavy metals, radiation, or pressure) significantly deviates from what is considered normal. Before the 1960s, it was thought that life could not thrive in such environments. However, the discovery of microorganisms in the thermal pools of Yellowstone and the isolation of the thermophile bacterium Thermus aquaticus (Brock and Freeze 1969) caused a paradigm shift, opening a new field of study. Since then, many extremophiles have been isolated from a variety of extreme environments, greatly expanding the known limits of life (Table 1). For further reading, Harrison et al. (2013) provide an extensive review on the limits of life. Furthermore, advancements in metagenomics, metatranscriptomics, and other omics technologies have allowed us to characterize the broad diversity of life in extreme environments (Shu and Huang 2022). A distinction can be made between extremotolerant and extremophilic organisms—that is, facultative versus obligate extremophiles. Many extremophiles thrive better under milder conditions (Matthews et al. 2023). For example, the bacterium Planococcus halocryophilus, originally isolated from soil in northern Canada, has the lowest recorded growth temperature (−15°C), but this extremophilic bacterium grows optimally at 25°C and sustains growth even at 37°C (Mykytczuk et al. 2013). In many cases, isolating, growing, and assessing the impact of an extreme factor on an organism (extremophile or otherwise) requires special considerations, and many specific techniques have been developed. These techniques have been covered by other reviews, such as Atasoy et al. (2024) for acidic pH, Kevbrin (2020) for alkaline pH, Lach et al. (2021) for halophiles, and Schultz et al. (2023) for extremophiles in general.
Extremophilic microorganisms cope with environmental conditions not tolerated by other organisms. An organism is considered an extremophile if its optimum for a specific environmental condition falls beyond the boundary given in the table, although these boundaries may vary slightly depending on the authors. We note that an organism may be considered as “tolerant” (e.g. thermotolerant, halotolerant) if it can grow beyond these boundaries, though not optimally. Also shown are currently known limits for activity of microbial isolates in the laboratory under the different extreme conditions discussed in this review.
Stressor . | Definition . | Reference for definition . | Limit . | Species . | Reference for limit . |
---|---|---|---|---|---|
High temperature | >50°C (thermophile) | Canganella and Wiegel (2011) | 122°C | Methanopyrus kandleri (archaeon) | Takai et al. (2008) |
Low temperature | <15°C (psychrophile) | Canganella and Wiegel (2011) | −25°Ca | Planococcus halocryophilus (bacterium) | Mykytczuk et al. (2013) |
Low pH | < pH 5 (acidophile) | Canganella and Wiegel (2011) | pH −0.06 | Picrophilus oshimae (archaeon) | Schleper et al. (1995) |
High pH | > pH 9 (alkaliphile) | Canganella and Wiegel (2011) | pH 12.5b | Alkaliphilus transvaalensis (bacterium) | Takai et al. (2001) |
High pressure | > 10 MPa (piezophile) | Fang et al. (2010) | >100 MPa | Colwellia marinimaniae (bacterium)c | Yayanos et al. (1981) |
High salinity | > 3% w/v (halophile) | Canganella and Wiegel (2011) | 36% w/v NaCl (saturation) | Halobacterium salinarum (archaeon)c | Eichler (2019) |
Low water activity | aw < 0.7 (xerophile) | Lebre et al. (2017) | 0.585 aw | Aspergillus penicilloides (fungus) | Lebre et al. (2017) |
Stressor . | Definition . | Reference for definition . | Limit . | Species . | Reference for limit . |
---|---|---|---|---|---|
High temperature | >50°C (thermophile) | Canganella and Wiegel (2011) | 122°C | Methanopyrus kandleri (archaeon) | Takai et al. (2008) |
Low temperature | <15°C (psychrophile) | Canganella and Wiegel (2011) | −25°Ca | Planococcus halocryophilus (bacterium) | Mykytczuk et al. (2013) |
Low pH | < pH 5 (acidophile) | Canganella and Wiegel (2011) | pH −0.06 | Picrophilus oshimae (archaeon) | Schleper et al. (1995) |
High pH | > pH 9 (alkaliphile) | Canganella and Wiegel (2011) | pH 12.5b | Alkaliphilus transvaalensis (bacterium) | Takai et al. (2001) |
High pressure | > 10 MPa (piezophile) | Fang et al. (2010) | >100 MPa | Colwellia marinimaniae (bacterium)c | Yayanos et al. (1981) |
High salinity | > 3% w/v (halophile) | Canganella and Wiegel (2011) | 36% w/v NaCl (saturation) | Halobacterium salinarum (archaeon)c | Eichler (2019) |
Low water activity | aw < 0.7 (xerophile) | Lebre et al. (2017) | 0.585 aw | Aspergillus penicilloides (fungus) | Lebre et al. (2017) |
Microbial activity has been detected at −39°C in permafrost soil samples (Panikov et al. 2006).
A diverse bacterial community lives at pH up to 13.0 in heavily contaminated Lake Calumet, SE Chicago, IN, USA (Ohlsson et al. 2019).
Other microbial species are also known to be active at >100 MPa or 36% NaCl.
Extremophilic microorganisms cope with environmental conditions not tolerated by other organisms. An organism is considered an extremophile if its optimum for a specific environmental condition falls beyond the boundary given in the table, although these boundaries may vary slightly depending on the authors. We note that an organism may be considered as “tolerant” (e.g. thermotolerant, halotolerant) if it can grow beyond these boundaries, though not optimally. Also shown are currently known limits for activity of microbial isolates in the laboratory under the different extreme conditions discussed in this review.
Stressor . | Definition . | Reference for definition . | Limit . | Species . | Reference for limit . |
---|---|---|---|---|---|
High temperature | >50°C (thermophile) | Canganella and Wiegel (2011) | 122°C | Methanopyrus kandleri (archaeon) | Takai et al. (2008) |
Low temperature | <15°C (psychrophile) | Canganella and Wiegel (2011) | −25°Ca | Planococcus halocryophilus (bacterium) | Mykytczuk et al. (2013) |
Low pH | < pH 5 (acidophile) | Canganella and Wiegel (2011) | pH −0.06 | Picrophilus oshimae (archaeon) | Schleper et al. (1995) |
High pH | > pH 9 (alkaliphile) | Canganella and Wiegel (2011) | pH 12.5b | Alkaliphilus transvaalensis (bacterium) | Takai et al. (2001) |
High pressure | > 10 MPa (piezophile) | Fang et al. (2010) | >100 MPa | Colwellia marinimaniae (bacterium)c | Yayanos et al. (1981) |
High salinity | > 3% w/v (halophile) | Canganella and Wiegel (2011) | 36% w/v NaCl (saturation) | Halobacterium salinarum (archaeon)c | Eichler (2019) |
Low water activity | aw < 0.7 (xerophile) | Lebre et al. (2017) | 0.585 aw | Aspergillus penicilloides (fungus) | Lebre et al. (2017) |
Stressor . | Definition . | Reference for definition . | Limit . | Species . | Reference for limit . |
---|---|---|---|---|---|
High temperature | >50°C (thermophile) | Canganella and Wiegel (2011) | 122°C | Methanopyrus kandleri (archaeon) | Takai et al. (2008) |
Low temperature | <15°C (psychrophile) | Canganella and Wiegel (2011) | −25°Ca | Planococcus halocryophilus (bacterium) | Mykytczuk et al. (2013) |
Low pH | < pH 5 (acidophile) | Canganella and Wiegel (2011) | pH −0.06 | Picrophilus oshimae (archaeon) | Schleper et al. (1995) |
High pH | > pH 9 (alkaliphile) | Canganella and Wiegel (2011) | pH 12.5b | Alkaliphilus transvaalensis (bacterium) | Takai et al. (2001) |
High pressure | > 10 MPa (piezophile) | Fang et al. (2010) | >100 MPa | Colwellia marinimaniae (bacterium)c | Yayanos et al. (1981) |
High salinity | > 3% w/v (halophile) | Canganella and Wiegel (2011) | 36% w/v NaCl (saturation) | Halobacterium salinarum (archaeon)c | Eichler (2019) |
Low water activity | aw < 0.7 (xerophile) | Lebre et al. (2017) | 0.585 aw | Aspergillus penicilloides (fungus) | Lebre et al. (2017) |
Microbial activity has been detected at −39°C in permafrost soil samples (Panikov et al. 2006).
A diverse bacterial community lives at pH up to 13.0 in heavily contaminated Lake Calumet, SE Chicago, IN, USA (Ohlsson et al. 2019).
Other microbial species are also known to be active at >100 MPa or 36% NaCl.
Extremophiles have been a focus in the search for novel genes, enzymes, and compounds with potential applications in pharmaceuticals (Patel et al. 2022), industrial processes (Zhu et al. 2020, Wang et al. 2024), and environmental biotechnology (Giovanella et al. 2020). They are also used as models in astrobiology (Hallsworth et al. 2021). Extremophilic microorganisms, like their less extreme relatives, produce microbial volatile organic compounds (MVOCs) (Fig. 1). These compounds have been extensively studied, with applications ranging from pathogen inhibition and plant growth promotion to influencing microbial community interactions. MVOCs are produced through various pathways, which have been described in other reviews, such as Weisskopf et al. (2021) and Lemfack et al. (2020). While the production of volatile organic compounds (VOCs) by plants growing in extreme environments has been discussed previously (Rinnan et al. 2014), this review is the first to focus on MVOCs and their roles in extreme environments.
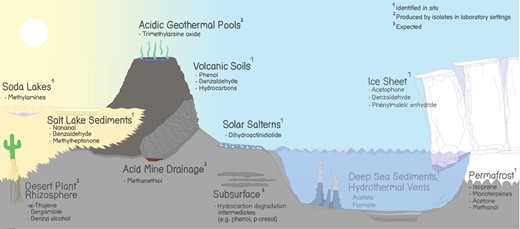
Some MVOCs associated with extreme environments. The references are: desert plant rhizosphere (Camarena-Pozos et al. 2021), salt lake sediments (Lu et al. 2021a), soda lakes (Sorokin et al. 2015), acid mine drainage (Baumler et al. 2007), acidic geothermal pools (Qin et al. 2009), volcanic soils (Randazzo et al. 2024), subsurface (Wartell et al. 2021), solar salterns (Donadio et al. 2011), deep sea sediments (Glombitza et al. 2019), deep sea hydrothermal vents (Zeng et al. 2021), ice sheets and caps (Doting et al. 2022), and permafrost (Jiao et al. 2023).
Extreme environments pose additional challenges when studying MVOCs compared to nonextreme environments. While some extreme environments support substantial biomass, such as the acidic Tinto River (López-Archilla et al. 2001) or hot deep-sea hydrothermal vents (Takai and Nakamura 2011), others, such as the deep subsurface, have minimal biomass or microbial activity (Hoehler and Jørgensen 2013). For instance, Bodaker et al. (2010) filtered 800 l of highly saline Dead Sea water to obtain enough biomass for constructing fosmid libraries. Metabolic activity, which is directly or indirectly linked to MVOC production, can be very low in specific environments, particularly in cold and/or low nutrient settings like deep subsurface soils or sediments (Sokol et al. 2022). Additionally, the specific characteristics of extreme environments often hinder measurement of MVOCs; for example, low temperatures drastically reduce the evaporation rates of volatile compounds and ice block the release of MVOCs from e.g. permafrost soil. Accessing certain environments, such as the deep sea or Earth’s crust, also poses logistical challenges, making it difficult to distinguish biogenic volatiles from abiogenic ones. In some environments, such as soil, microorganisms reside in small, discrete microhabitats (e.g. pores or cracks in rocks), with distinct physico-chemical conditions (Effmert et al. 2012). This heterogeneity may result in the production of different MVOCs, further complicating the distinction between microenvironments.
In this review, we explore the challenges microorganisms face in extreme environments, their production of MVOCs, and the functions and applications of MVOCs in these settings. To provide context, we begin with a brief introduction to MVOCs and the methodologies used to study them. We then discuss various extreme environments, characterized by their most prevalent extreme factor, such as high temperatures or extreme pH. However, it is important to note that many extreme environments have more than one extreme variable. For example, brine inclusions in permafrost soil exhibit both low temperatures and high salt concentrations, while solar salterns combine high salt concentrations with extreme UV exposure. Thus, microorganisms in seemingly different environments may share common adaptation strategies. Finally, we highlight the broader significance of MVOCs in areas such as climate change, agriculture, biotechnology, and astrobiology.
VOCs
VOCs are organic molecules with high vapour pressure (the pressure of the gas phase above the liquid or solid phase) and low water solubility. These compounds exhibit diverse structures and may contain various functional groups, including alkanes, ketones, amines, and thiols. All organisms produce biogenic VOCs (BVOCs), and global biogenic emissions far surpass those from human activities (Sindelarova et al. 2022). BVOCs are byproducts of primary and secondary metabolism of plants, fungi, prokaryotes, and animals (Korpi et al. 2009). Among these, plant emissions have been the most extensively studied, as they account for the majority of BVOC emissions in terrestrial ecosystems (Sindelarova et al. 2014). The environmental relevance of BVOCs stems from their atmospheric chemistry, where they influence air quality (Calfapietra et al. 2013, Eisenman et al. 2019) and contribute to the formation of secondary organic aerosols, which serve as cloud condensation nuclei (Shrivastava et al. 2017). In addition, BVOCs play roles in defence, stress responses, and communication (Loreto and Velikova 2001, Dudareva et al. 2013, Brosset and Blande 2022, Kessler et al. 2023).
Microorganisms are prolific producers of volatile compounds. The functions of MVOCs, much like their structures, are diverse (Fig. 2). To date, many MVOCs have been identified, with the mVOC 3.0 database cataloguing ~2000 unique compounds produced by microorganisms (Lemfack et al. 2018). Notably, up to 27% of the metabolites in the Kyoto Encyclopedia of Genes and Genomes (KEGG) are potentially volatile; of which 37% are uniquely associated with prokaryotes (Meredith et al. 2023). Moreover, a substantial number of yet unidentified MVOCs still remain to be described (Seager et al. 2016). MVOCs are critical in both intra- and interspecies communication (Weisskopf et al. 2021) and aid organisms in adapting to environmental conditions. While some MVOCs, such as ethanol, are merely byproducts of fermentation or other metabolic processes aimed at expelling excess metabolites (Shirk et al. 2002), others serve specialized roles in microbial competition and cooperation, as these compounds can diffuse across both liquid and gaseous phases (Weisskopf et al. 2021). Studies have demonstrated that MVOCs can stimulate or inhibit the growth of plants, fungi, protozoa, and bacteria, as well as influence bacterial quorum sensing, biofilm formation, and antibiotic resistance (Chernin et al. 2011, Bitas et al. 2013, Schulz-Bohm et al. 2017, Hou et al. 2021). Additionally, microorganisms may emit MVOCs to attract mutualistic animals (Becher et al. 2020) or as a defence mechanism against predation (Nguyen et al. 2022).
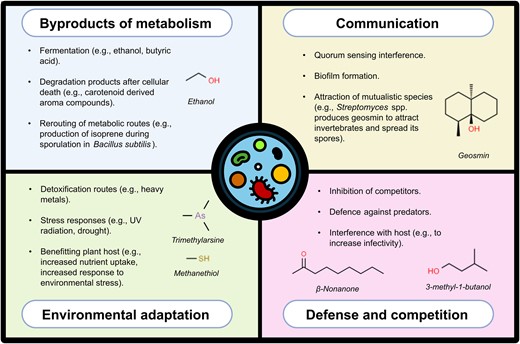
Over recent decades, the field of volatolomics (also referred to as volatilomics) has emerged as a frontier in biology, chemistry, and biochemistry, focusing on the emission profiles of volatile compounds in various biological systems. This discipline, a member of the “omics sciences” alongside genomics, transcriptomics, and metabolomics, employs high-throughput techniques to analyse hundreds of volatile compounds, encompassing VOCs, nitrogen- and sulphur-containing volatiles, and other inorganic compounds (Box 1). Volatolomics boasts a broad range of applications, including community activity and biodiversity (McNeal and Herbert 2009, Insam and Seewald 2010, Wang et al. 2016, Steinke et al. 2018, Usai et al. 2020, 2021), exposure to pollutants (Bouhlel et al. 2017, Ratel et al. 2017, Hidalgo et al. 2019), medical diagnostics (Kai Zhang 2014, Leja et al. 2016), fermentation and composting monitoring (Aboudi et al. 2021), food quality control (Starowicz 2021), climate change monitoring (Yuan et al. 2009), and human health assessments (Saalberg and Wolff 2016, Sun et al. 2016, Tiele et al. 2020) Additionally, MVOCs have potential as biosignatures in astrobiology because they can be detected at low concentrations and exhibit a wide diversity, with distinctly biogenic fingerprints produced by living organisms (Seager et al. 2012, Batty et al. 2025).
When analysing VOCs, they are typically collected from the gas phase above the sample, known as the headspace. Over the years, numerous VOC sampling techniques have been developed. These methods are generally categorized as either passive or active sampling (Fig. 3). In both approaches, adsorptive matrices are often employed to preconcentrate the VOCs (Wang et al. 2016). Matrices are typically composed of activated carbon or polymers such as polydimethylsiloxane, sometimes combined with divinylbenzene to broaden the range of volatiles captured. Various matrices, each with distinct properties, are available to better target specific compounds, such as nitrogen- or sulphur-containing volatiles or low-molecular-weight compounds. Several commercial solutions have also been developed, including solid-phase microextraction (SPME), stir bar sorptive extraction (SBSE), and sorbent cartridges. For a comprehensive overview of headspace sampling techniques, see Soria et al. (2015).
Following sampling, VOCs are either extracted or thermally desorbed and subsequently analysed using gas chromatography–mass spectrometry (GC–MS) and similar techniques (Soria et al. 2015). The separation of the volatiles in the GC column prior to analysis improves the precision of compound identification, even enabling the distinction between different enantiomers. An alternative technique, proton transfer reaction mass spectrometry (PTR-MS), enables real-time analysis of the samples (Lindinger et al. 1998). Due to the absence of a prior separation step, identifying larger compounds is challenging. Nevertheless, its high sensitivity, real-time capabilities, and ability to detect low-molecular-weight VOCs that may be missed by sorbent matrices make PTR-MS a powerful tool for VOC analysis.
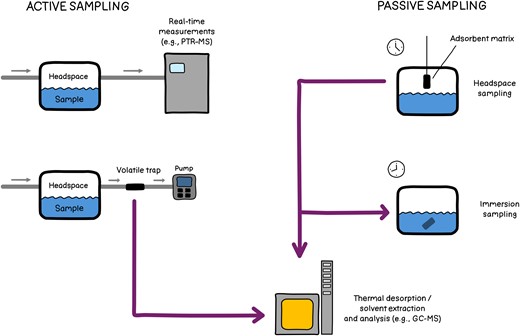
Different methods to sample MVOCs. During active sampling, air is flushed at constant rate over the sample for online analysis of MVOCs using, e.g. PTR-MS, or for trapping of MVOCs on an absorbent material before analysis using, e.g. GC–MS. During passive sampling, an absorbent material in the headspace or submerged in the liquid phase of the sample collects MVOCs during a defined time span.
Extremely cold environments
Microorganisms with an optimal growth temperature below 15°C are classified as psychrophiles. At such low temperatures, metabolic reaction rates and diffusion rates decline significantly. Below 0°C, ice crystal formation can occur, potentially obstructing the transfer of nutrients, waste products, and gases. Cellular membranes lose fluidity, eventually undergoing gel-phase transition, which leads to functional impairment. Additionally, the formation of intracellular ice crystals poses a severe risk, necessitating specific adaptations. One common adaptation is the accumulation of cryoprotectants–compounds that lower the melting point of water and prevent intracellular ice crystal formation, which would otherwise cause cell death. Examples of such cryoprotectants include VOCs or their precursors, such as dimethylsulphoniopropionate, a precursor to the volatile dimethyl sulphide, found in sea-ice algae (Thomas and Dieckmann 2002). In other cases, microorganisms employ Ice Binding Proteins to modulate ice crystal growth (Dolev et al. 2016). For further reading on the adaptations of psychrophiles, see Collins and Margesin (2019).
Measuring in situ production of MVOCs in cold environments is challenging, as low temperatures not only inhibit microbial activity but also reduce the vapour pressure of MVOCs, resulting in lower concentrations of volatiles in the headspace and diminished measurement sensitivity. This problem can be mitigated in specific environments, such as cold brines, where the salting-out effect (Copolovici and Niinemets 2007) partially offsets the reduction in vapour pressure cause by low temperatures. Where feasible, sample heating may enhance the evaporation of pre-existing MVOCs, improving detection.
Ice sheets and glaciers are home to diverse microbial communities, including photosynthetic microorganisms that change the colour of the ice, resulting in “red” or “green” snow. These microorganisms emit a wide range of MVOCs, some of which exhibit antifungal properties. Doting et al. (2022) identified acetophenone, benzaldehyde, and phenylmaleic anhydride as the predominant MVOCs among the 92 detected compounds from surface microbial communities on the southern Greenlandic Ice Sheet. These compounds’ antifungal properties suggest complex interactions among the ice-dwelling microorganisms. Additionally, MVOCs and primary aerosols deposited from other sources, like the ocean, may provide nutrients to glacial microbial communities when biogenic aerosols precipitate on the ice surface (Antony et al. 2014). Furthermore, sea-ice microorganisms are recognized as significant contributors of atmospheric nitrogen-containing MVOCs (Dall’Osto et al. 2017) and emit sulphur-containing MVOCs, particularly dimethyl sulphide (Thomas and Dieckmann 2002).
Permafrost soils—soils that remain frozen for at least two consecutive years—have been a focal point of research due to their inevitable thawing under the influence of climate change. This thawing has significant implications for the microbial degradation and release of its vast reserves of organic matter, estimated at ~800 petagrams (Pg) (Hugelius et al. 2014), into the atmosphere as greenhouse gases (Schuur et al. 2008). The microbiome of permafrost soils has been extensively studied for its role in decomposing organic matter upon thawing (Graham et al. 2012). The seasonally thawed layer above the permafrost, known as the active layer, maintains temperatures above freezing during the summer, supporting higher microbial metabolic activity. This affects both the production of MVOCs by active layer microorganisms and the consumption of volatiles released from microbial activity in the recently thawed permafrost soil. Net MVOC emissions from permafrost are influenced by microbial communities and physical factors, such as meltwater drainage (Kramshøj et al. 2019). Some of the most abundant MVOCs in permafrost soils are low-molecular-weight compounds like ethanol, methanol, or acetaldehyde, but other MVOCs, such as aromatic compounds and terpenes, can also be emitted (Li et al. 2020). Kramshøj et al. (2018) showed that these compounds, released by the frozen layer, can be consumed in the active layer. Despite the potential of microorganisms in this active layer to degrade these compounds and prevent them from being released into the atmosphere, thawing permafrost soil remains an important source of MVOCs (Jiao et al. 2023). Psychrophilic and psychrotolerant MVOC-producing microorganisms within frozen permafrost may slowly produce MVOCs over millennia, leading to their accumulation in the frozen soil. These MVOCs are then released into the atmosphere upon thawing under a warmer climate.
Brine inclusions in ice or permafrost soil (cryopegs) and brine lakes provide refuge for polyextremophiles—microorganisms thriving under two or more extreme environmental conditions. The presence of salt lowers the freezing point of water, preventing ice crystal formation. This allows microorganisms to remain metabolically active, resulting in significantly higher cell counts compared to the surrounding frozen material (Colangelo-Lillis et al. 2016). Rapp et al. (2021) found diverse communities both in sea ice brine inclusions and cryopegs, dominated by Marinobacter in cryopegs and by Polaribacter, Paraglaciecola, and Colwellia in sea ice brine inclusions. Marinobacter has been found to produce ketones, esters, and sulphur-containing and halogenated MVOCs, among other compounds (Lawson et al. 2020).
Polar oceans are notable sources of MVOCs with potential applications as antimicrobial agents and plant growth-promoters. For instance, Papaleo et al. (2013) identified 30 different MVOCs in the volatolome of Pseudoalteromonas and Psychrobacter isolated from Antarctic sea sponges. These include compounds such as dimethyl sulphide, butanal, (S-methyl) 3-methylbutanethioate, and acetophenone. These strains showed MVOC-mediated antimicrobial activity against the pathogenic Burkholderia cepacia complex, although the specific MVOCs involved in this inhibition were not identified. In a different study on bacteria from Antarctic seawater and sediments, Maida et al. (2015) found Pseudoalteromonas strains with MVOC-mediated antimicrobial activity against B. cepacia. Antarctic bacteria of different genera constitutively produce a diverse blend of MVOCs, suggesting that volatile compounds may have an unknown ecological purpose (Lo Giudice and Fani 2015).
Several Pseudomonas strains isolated from Antarctic soil have been shown to promote wheat germination at low temperatures, partly by inhibiting pathogenic fungi through the emission of volatiles (Yarzábal et al. 2018). While Yarzábal et al. (2018) did not identify the specific MVOCs produced, they suggested that hydrogen cyanide, which was detected, played a role in the antifungal activity. Additionally, MVOCs with known growth-promoting effects on Arabidopsis, such as 1-hexanol and tetradecane, have been identified in yeasts isolated from Arctic flowers (Niu et al. 2022). This ability of psychrophiles to promote plant growth and suppress pathogens reveals potential MVOC-mediated interactions in their natural habitats and suggests possible applications for agriculture in cold regions, such as boreal zones and mountainous areas.
Extremely hot environments
Thermophilic microorganisms grow optimally at or above 50°C with those thriving above 80°C classified as hyperthermophiles. Elevated temperatures increase the fluidity of the cytoplasmic membrane and destabilize proteins, DNA, and metabolites. To adapt to these challenges, thermophilic and hyperthermophilic bacteria have evolved specialized membranes containing a higher proportion of saturated fatty acids and hyperthermophilic archaea possess monolayer structured membranes, along with specific protein and DNA adaptations that enhance stability at high temperatures. For further reading, Pati et al. (2023) provide an excellent chapter on thermophilic adaptations.
High temperatures can be advantageous for VOC analysis, as they increase evaporation rates. In laboratory settings, samples can be heated to improve sensitivity. However, caution is needed when sampling MVOCs in very hot environments, as excessive temperatures can reduce the adsorption efficiency of sorbent materials (Kroupa et al. 2004). Some semivolatile compounds that are practically nonvolatile at room temperature, such as indole, become detectable in the gas phase at elevated temperatures. In hot deserts, soil temperatures can exceed 70°C, as shown by satellite data (Mildrexler et al. 2011). In such environments, water scarcity poses an additional challenge to microorganisms. This topic is covered in the following section on extremely dry environments.
High temperatures are generated during the composting of organic waste, with microbial metabolism driving temperatures up to nearly 80°C (Jäckel et al. 2005). However, microbial activity generally declines when the temperature exceeds 60°C; for instance, thermophilic fungi do not grow above 62°C (Patel and Rawat 2021). During aerobic and anaerobic composting, many volatiles are produced and emitted into the atmosphere at high temperatures. Dozens of compounds, including MVOCs, such as alcohols, ketones, terpenoids, and xenobiotic volatiles, are emitted during the composting of the organic fraction of municipal solid waste (Komilis et al. 2004). Lin et al. (2023) observed a succession of VOCs during the cocomposting of diesel and food waste, Alcohols and esters were dominant during the early, mesophilic conditions, while branched alcohols, aldehydes, ketones, sulphur-containing VOCs, amines, and terpenes were released during the thermophilic phase. However, the terpenes likely originated from plant matter decomposition rather than microbial synthesis. Volatile compounds and other trace gases emitted from landfills are an environmental and health concern (Duan et al. 2021).
Even higher temperatures are observed in hydrothermal areas, where complex interactions between the hydrothermal fluid and surrounding geological features can result in the formation of abiogenic VOCs. These compounds may subsequently be consumed or transformed by microbial activity. In hydrothermal regions of central Italy, Randazzo et al. (2024) found evidence of microbial degradation of geogenic hydrocarbons, along with the production of oxygenated MVOCs, such as alcohols, phenols, and ketones, which are intermediaries of hydrocarbon degradation pathways. This highlights the importance of microbial activity in the reduction or modification of abiotic VOCs, a conclusion which is also supported by previous studies (Tassi et al. 2015). In deep-sea hydrothermal vents, where high pressure prevents water from boiling, temperatures can reach up to 400°C, while the surrounding sea water remains at 2°C–3°C. The temperatures and chemical compositions of these hydrothermal environments can vary depending on local geology and the intensity of hydrothermal activity. Several heterotrophic and lithoautotrophic bacteria and archaea, including methanogens, sulphur reducers and oxidizers, and hydrogen oxidizers, have been isolated from deep-sea hydrothermal vents (Zeng et al. 2021). Although little is known about MVOC production and consumption in these remote environments, some hydrothermal vent bacteria have been shown to volatilize mercury as an adaptation to high concentrations of heavy metals in hydrothermal fluids (Vetriani et al. 2005) Additionally, low molecular weight volatile fatty acids (VFAs) like acetate and formate, produced by bacteria and archaea, can be utilized by other deep-sea hydrothermal microorganisms (Zeng et al. 2021).
Thermophiles have significant potential as producers of valuable products, including MVOCs. The high temperatures at which they thrive facilitate the recovery of volatile products via vapour condensation, especially when the organism grows above the boiling point of the product. Furthermore, the high incubation temperatures reduce the risk of contamination. For example, Kato et al. (2021) genetically engineered the thermophilic bacterium Moorella thermoacetica, which has an optimal growth temperature of 55°C, to produce acetone. Similarly, Keller et al. (2015) genetically engineered the archaeon Pyrococcus furiosus to produce 1-butanol at 70°C. This approach has been applied to the production of VFAs and alcohols from waste during thermophilic decomposition in several studies (Yu and Fang 2001, Yao et al. 2019, Amanidaz et al. 2023). Thermophiles have also garnered interest as potential producers of biofuels such as ethanol (Zuliani et al. 2021). Additionally, thermophiles can be used to modify VOC blends; for instance, Kong et al. (2001) studied thermophiles in biofilters as potential degraders of methanol and α-pinene, common VOCs emitted by the pulp and forestry industry.
Environments with extreme pH
Organisms can be categorized based on their optimum pH into acidophiles (optimal growth below pH 5), neutrophiles, and alkaliphiles (optimal growth above pH 9). Extreme acidophiles are typically defined as having a pH optimum below 3, while extreme alkaliphiles show optimal growth above 10 (Dhakar and Pandey 2016). Despite the potentially extreme pH values outside the cell and the resulting significant proton gradient across the cellular membrane, both acidophiles and alkaliphiles generally maintain a near-neutral cytosolic pH, which is crucial for protein and nucleic acid stability. Common adaptations include proton pumps and membranes with reduced proton permeability. For further information on microbial adaptations to environments with extreme pH, see Chen (2021) for acidophiles and Mamo (2020) for alkaliphiles.
Certain volatile compounds consisting solely of carbon and hydrogen, such as isoprene, may not exhibit significant differences in intermolecular interactions at varying pH values. However, volatiles containing oxygen- or nitrogen-based functional groups can behave differently across different pH ranges. For example, polychlorinated biphenyls, persistent organic pollutants, tend to adsorb more readily to soil particles at neutral or lower pH, as soil organic acids become protonated and more hydrophobic under such conditions (Adeyinka and Moodley 2019). Similarly, organic volatiles with carboxyl groups more easily diffuse into the gas phase at low pH because they are converted to their neutral carboxylic acid form, which reduces interactions with water and other polar molecules. In contrast, volatiles containing amine groups become protonated at low pH, forming cations with increased hydrophilicity and reduced vapour pressure. Additionally, volatiles may also undergo chemical reactions under extreme pH values depending on the physicochemical environment. For example, ketones and aldehydes may undergo aldol condensation reactions (Nielsen and Houlihan 2011), while alcohols and esters may undergo transesterification (Otera 1993).
Several studies have investigated the production of MVOCs in acid and alkaline environments. Ferroplasma acidarmanus, an acidophilic archaeon, produces methanethiol constitutively, and may play an important role in the sulphur cycle in its natural habitat (Baumler et al. 2007). The Tinto River in Southern Spain is the result of microbial oxidation of sulphide deposits and represents the largest acidic environment on Earth, with an average pH of 2.3 and high concentrations of arsenic, copper, and other toxic heavy metals (Daddaoua et al. 2023). Due to these harsh conditions, the extremophiles in the Tinto River have been extensively studied for their potential applications in bioremediation, biotechnology, and astrobiology. These heavy metals may be transformed into volatile organometallic compounds, as discussed in a later section of this review. MVOCs are common in the Tinto River’s sediments: Sánchez-Andrea et al. (2012) found that acetate was abundant in the brown, reducing layers, while formate was present throughout. Although MVOC production in many acidophiles has not yet been investigated, MVOC production by closely related species suggests that some MVOCs could serve as adaptation strategies to low pH. For example, the green alga Chlamydomonas acidophila, isolated from the Tinto river, is a model for studying heavy metal resistance (Puente-Sánchez et al. 2018). The type strain of this genus, C. reinhardtii, produces 3-methyl-2-pentanone, octanal, and other volatile compounds under acetic acid and salt stress (Zuo et al. 2012a, b). It is plausible that C. acidophila similarly emits specific MVOCs in response to stress.
Environments with a pH above 9 are rare on Earth due to the buffering effect of atmospheric CO2. Examples include insect stomachs, soda lakes, ikaite structures (Buchardt et al. 1997), and specific deep sea hydrothermal vents (Kelley et al. 2001). Many cyanobacteria are haloalkaliphilic or haloalkalitolerant, and are known to produce a variety of MVOCs (Zuo 2019). They serve as the main primary producers in moderately saline soda lakes (Jones et al. 1998), which, along with anoxygenic phototrophic bacteria, support rich ecosystems of archaea, bacteria, and viruses that thrive in these high pH, high salinity environments (Grant and Jones 2016). Spirulina is typically the dominant cyanobacterial genus in these environments and has been shown to produce alkanes, alkenes, 2-methylisoborneol, geosmin, and other odorous compounds (Milovanović et al. 2015). Additionally, nitrogen-containing MVOCs, such as methylamines and VFAs, are commonly found in soda lakes (Sorokin et al. 2015).
Extremely dry environments
Liquid water is essential for all known life on Earth, as it is crucial for many biomolecular processes, including protein folding and stability, enzyme–substrate interactions, and the maintenance of cellular structure (Lebre et al. 2017). Without water, cellular components such as lipid membranes, proteins, DNA, and RNA denature, leading to cell death unless resistance mechanisms are present. Some bacteria, such as Bacillus spp., form dormant endospores to protect their genetic material until conditions improve. Microorganisms capable of surviving with limited water are referred to as xerotolerant, while xerophiles can sustain activity in environments with low water activity (<0.7) compared to pure water, which has a water activity of 1. Low water activity can result from an actual lack of water (deserts), high osmotic pressure (as in brines), or freezing. These conditions are often associated with other stresses, such as low or high temperatures and high levels of UV radiation or osmolytes. As hypersaline and icy environments are discussed elsewhere in this review, this section focuses on hot deserts. While life may not be widespread in such environments, it has found refuge in protective niches, such as within the soil, in the rhizosphere of plants, and beneath (hypolithic) or within rocks (endolithic) (Wierzchos et al. 2012). Sajjad et al. (2022) provides an extensive review on endoliths.
Lithic communities, including hypo- and endolithic communities, vary depending on environmental factors and rock composition (Chan et al. 2012, Coleine et al. 2023). Typically, they contain photosynthetic primary producers, on which different fungi, bacteria, and archaea are dependent. Such primary producers often include the alga Trebouxia and cyanobacteria such as Chroococcidiopsis, Leptolyngbya, and Gloeocapsa (Chan et al. 2012, Meslier and DiRuggiero 2019). While there is no literature on the production of MVOCs by these communities themselves, their production is likely, as MVOC-mediated interactions have been observed in related species. For example, Gloeocapsa alpicola is inhibited by MVOCs produced by Bacillus (Wright and Thompson 1985) and cyanobacteria are known to produce a variety of volatile compounds (Achyuthan et al. 2017). Furthermore, some MVOCs, such as formic acid, can result in the dissolution of minerals, solubilizing nutrients and facilitating colonization by microorganisms (Puente et al. 2004).
A more familiar environment with low water activity, albeit less extreme, is found in the rhizosphere of xerophytes, plants adapted to survive with minimal water. Plant roots secrete exudates and organic molecules into the surrounding soil, which serve as nutrients, attracting various microorganisms, including bacteria and fungi (Marschner 2012). Several studies focus on these microbes, particularly due to their potential as plant growth enhancers in agriculture (Marasco et al. 2012, 2018, Araya et al. 2020, Zuo et al. 2021). Nevertheless, there are few studies reporting MVOC emissions from the roots of dryland plants. Camarena-Pozos et al. (2021) found 94 compounds of diverse chemical classes produced by 22 fungal isolates from agaves and cacti, important dryland plants. These MVOCs included diverse terpenes like α-thujene and (-)-β-pinene, alcohols such as phenol or esters such as bergamiol. The study also revealed that some of these volatiles, including camphene, benzyl benzoate, or benzyl alcohol, improved plant growth in Arabidopsis thaliana, Agave tequilana, and Agave salmiana. In a similar study, Camarena-Pozos et al. (2019) screened 40 bacterial strains from the microbiome of cacti and agave. They found novel compounds not listed in existing MVOC databases, such as ethyl benzoate. Furthermore, they found that some MVOCs such as ethyl isovalerate and 3-methyl-1-butanol promote the growth of A. thaliana, A. tequilana, and A. salmiana. Acidic MVOCs released by rhizosphere microorganisms can also improve root colonization and rock weathering in deserts (Puente et al. 2004). Microorganisms in less extreme environments also produce some of these compounds. However, for xerophiles, the MVOC production likely represents an adaptation to their niche in the rhizosphere of desert plants. Utilizing these microorganisms as biofertilizers could enhance agricultural productivity in arid and semiarid regions (Alsharif et al. 2020).
Hypersaline environments
Microorganisms can be classified based on their salt requirements for optimal growth, ranging from nonhalophiles to halophiles (optimal growth at >3% w/v NaCl) and extreme halophiles (optimal growth >15% w/v NaCl), some of which can thrive in saturated NaCl solutions (∼36% w/v). To sustain osmotic balance and cellular function, halophilic and halotolerant microorganisms employ two primary strategies: accumulating compatible solutes such as glycerol or ectoine (“salt-out”) or accumulating KCl ions (“salt-in”) (Saini et al. 2023). Volatile compound analysis in these environments benefits from the salting-out effect (Copolovici and Niinemets 2007). Additionally, certain MVOCs are specifically derived from compatible solutes. A notable example is dimethylsulfoniopropionate, which also serves as a cryoprotectant in marine phytoplankton and acts as a precursor to dimethyl sulphide and methanethiol (Bentley and Chasteen 2004). In phytoplankton, dimethyl sulphide production increases with rising salinity due to enhanced accumulation of dimethylsulfoniopropionate (Zhao et al. 2023).
Salterns are rich ecosystems, hosting a variety of halophilic microorganisms, including photosynthetic and heterotrophic organisms, both aerobic and anaerobic. Due to intense solar radiation in these environments, native microorganisms produce various pigments, such as carotenoids, which help mitigate UV-damage. These pigmented molecules can undergo degradation, either biotically or abiotically, to produce volatile “aroma” compounds (Waché et al. 2003). Notably, some of these aroma compounds, such as the terpenoid dihydroactinidiolide, have commercial value, as they contribute to the distinctive characteristics of fleur de sel, one of the most expensive sea salt varieties (Donadio et al. 2011). The antifungal activity of some MVOCs from halophilic bacteria has also been investigated. Toral et al. (2021) identified several MVOCs produced by halotolerant bacterial strains, such as acetoin, dimethyl disulphide, and 2,3-butanediol, and studied their antifungal properties. However, since the bacteria were not cultured in high-salinity conditions for this study, MVOCs specific to such environments may have been overlooked.
Salt lakes are significant sources of MVOCs and atmospheric aerosols, particularly when organic matter, such as dead leaves below the salt crust, is present (Kamilli et al. 2016). Salt lake sediments act as reservoirs of MVOCs, containing a complex mixture of volatiles such as terpenes, alcohols, and acids. Lu et al. (2021a) quantified 34 MVOCs in the sediments of East Taijinar Lake in China and reported that higher organic matter decomposition in the sediments correlated with increased MVOC production. The study highlights the importance of volatiles in environments, where they constitute a significant portion of the total organic matter. In a subsequent study, Lu et al. (2021b) observed changes in MVOC composition in different strata of the same lake, with the largest variation in phenol and aldehyde proportions. Phenols accumulated during warm, humid periods, whereas aldehydes—primarily produced by diatoms—dominated during cooler, drier periods. This analysis enabled the authors to infer the paleoclimate history of the lake, highlighting the potential of MVOCs as proxies in paleoclimatology. Additionally, volatile chlorinated hydrocarbons, such as trichloroethene, can be produced by archaeal Halobacteria in salt lake sediments (Weissflog et al. 2005), including the Dead Sea (Shechner et al. 2019). These compounds are potentially phytotoxic and may have ecological implications.
Halophiles have also been investigated as potential producers of low molecular weight oxygenated VOCs, such as ethanol and butanol, for biofuel applications (Amoozegar et al. 2019). Moreover, halophiles, including the green alga Chlorella, can methylate and volatilize selenium (Fan et al. 1997), a process observed in the Great Salt Lake in the western USA (Diaz et al. 2009).
Other extreme environments
Although the presence of toxic heavy metals is not traditionally considered an extreme factor, these elements are frequently encountered in extreme environments, such as acidic environments and those with a volcanic or hydrothermal origin. Heavy metals, including arsenic, lead, and chromium, induce toxicity in cells either directly (e.g. substituting essential cations in enzymes) or indirectly (e.g. production of reactive oxygen species). Microorganisms have evolved several mechanisms to cope with these elements, ranging from intracellular compartmentalization to extracellular exclusion, extensively reviewed elsewhere, (Nanda et al. 2019, Pal et al. 2021). Of particular relevance to this review is the volatilization of heavy metals into organometallic volatile compounds. This process often involves methylation of the metal, though the formation of other volatile inorganic compounds, such as arsine (AsH3) (Mohammed et al. 2024) or elemental mercury (Hg0) (Mathema et al. 2011), is also common. Examples of volatile organometallic compounds include dimethyl mercury, methyl selenide, and dimethyl lead. Although methylation may increase the toxicity of heavy metals, it also facilitates their diffusion out of the cell and away from their immediate proximity. Microbial volatilization has been observed for lead, mercury, selenium, tin, arsenic, and antimony (Pal et al. 2021). While the mechanisms of microbial production of dimethyl mercury remain unclear, it has been detected in Desulfovibrio desulfuricans and may represent an important detoxification strategy in sulphate-reducing bacteria (Baldi et al. 1995). This process, along other forms of mobilization and immobilization, is important in bioremediation (Jing and Kjellerup 2018) and contributes significantly to the global geochemical heavy metals cycling (Gadd 2010). Unfortunately, certain methylated heavy metals can bioaccumulate and affect human health and ecosystems (Ali et al. 2019). A nonexhaustive list of extremophiles capable of volatilizing heavy metals includes the archaeon Halobacterium [mercury (Al-Mailem et al. 2011) and possibly arsenic (Wang et al. 2004)], the archaeon and bacterium Halococcus and Haloferax, respectively [mercury (Al-Mailem et al. 2011)], the bacterium Acidithiobacillus ferrooxidans [mercury (Takeuchi et al. 2001)], and the alga Cyanidioschyzon [arsenic (Qin et al. 2009)]. In addition, arsenic volatilization in Yellowstone National Park, USA, has been attributed to thermophiles (Planer-Friedrich et al. 2006). Microbial volatilization of heavy metals may be of particular importance in environments like acid mine drainages, which tend to be rich in heavy metals.
Pressure can also define an extreme environment. The upper troposphere and lower stratosphere are characterized by low pressure (around 8 kPa at 17 km, the altitude of the tropopause near the equator), high UV radiation, low water, and low nutrient availability. While aerial microbial transport is important on a global scale, determining whether the microbes found here are merely dormant transients or metabolically active residents remains challenging. Recent studies have provided evidence supporting the latter hypothesis (DeLeon-Rodriguez et al. 2013, Bryan et al. 2019), though it remains uncertain whether microbes permanently reside at such altitudes. It is currently unknown whether microorganisms in the atmosphere produce any specific MVOCs, although they have been shown to metabolize atmospheric formate, acetate, and phenol in cloud water (Hu et al. 2018, Khaled et al. 2021). Furthermore, these microbes may influence and transform local MVOC profiles upon deposition from the atmosphere.
On the other end of the scale, organisms inhabiting the deep sea or deep in the Earth’s crust are subjected to hydrostatic pressures of up to 150 MPa. Organisms with growth optima above 10 MPa are termed piezophiles (formerly barophiles) (Kanekar and Kanekar 2022). High pressure reduces membrane fluidity and can denature proteins, necessitating specialized adaptations, such as using smaller amino acids in proteins or incorporating more unsaturated fatty acids in membranes. For detailed discussion on piezophile adaptations, see Scheffer and Gieg (2023).
In the ocean, pressures exceeding 10 MPa occur below depths of 1000 m, corresponding to 88% of the total ocean area (Jebbar et al. 2015). Water temperatures in these regions average just above freezing (2°C–3°C), and hydrostatic pressure can reach 110 MPa in deep-sea trenches. Microorganisms in marine sediments produce MVOCs during the decomposition of organic matter, a process that persists even at significant depths (Oguri et al. 2022). VFAs, intermediates in the mineralization of organic matter (Glombitza et al. 2019), are commonly found in deep-sea marine sediments. In a recent study, Hu et al. (2024) found that the deep-sea bacterium Spongiibacter nanhainus inhibits human pathogens, including Pseudomonas aeruginosa, using a blend of 14 MVOCs, six of which were previously unreported as microbial products such as 2-hydroxy-iso-butyrophenone. Testing each MVOC separately for pathogen inhibition, the authors proposed an inhibition model involving disruptions in cell division, iron uptake and quorum sensing. The existence of these blends of MVOCs in remote environments raises questions about the interactions among the life that inhabits them and exemplifies the potential to discover novel compounds with diverse applications.
At greater depths within the subsurface, pressures increase further. Evidence supports the existence of a subsurface biosphere extending several kilometres below the surface (McMahon and Ivarsson 2019), though MVOC production in these environments remains poorly understood. Roumagnac et al. (2020) measured the metabolic activity of two Pseudothermotoga elfii bacterial strains, isolated from a deep African oil well, by measuring VFA production. Additionally, fungi such as the genus Exophiala are residents in the deep subsurface and degrade hydrocarbons (Hirayama et al. 2012, Isola et al. 2021). Hydrocarbon degradation, common in anaerobic environments like the deep subsurface and deep sea marine sediments, may lead to the formation of volatile intermediates like phenol and p-cresol (Wartell et al. 2021).
Certain environments are characterized by cycles of changing conditions, such as freeze–thaw cycles or dry–wet cycles, where pH, salinity, or others factors periodically reach extremes (Table 1). Examples include surface soils on deserts, the active layer of Arctic soils, which undergo seasonal thawing, intertidal areas, and fluctuating freshwater springs in the Dead Sea (Ionescu et al. 2024). Temperature fluctuations and periodic flooding affect microbial activity and influence MVOC production and uptake in subarctic regions (Baggesen et al. 2022). In tropical soils, drought increases emissions of carbonyl MVOCs, such as acetone and butanone, while rewetting triggers short-lived peaks of sulphur-containing VOC emissions, such as methanethiol and dimethyl sulphide (Pugliese et al. 2023). Similar dynamics may occur in more extreme environments. Intertidal areas, where evaporation creates highly saline tide pools (Mandal et al. 2021), are a source of novel MVOC-producing strains with biotechnological potential; for instance, Zou et al. (2021) found several yeast isolates which inhibit grey mould in strawberry plants through the release of MVOCs.
Significance of MVOCs produced by extremophiles
MVOC-producing extremophiles have been extensively studied for their potential biotechnological applications, including industrial use as antimicrobial agents, plant growth promoters, and bioactive compounds (Table 2). While many of the compounds produced by extremophiles are similar to those of mesophilic organisms, the ability of extremophiles to thrive in extreme environments gives them unique potential.
Biotechnological applications of extremophiles and their MVOCs reported in the literature. N/D: not determined. VFAs: volatile fatty acids.
Organism(s) . | Type of extremophiles . | Application . | MVOC . | References . |
---|---|---|---|---|
Pseudoalteromonas sp., Psychrobacter sp. | Psychrophiles | Inhibition of human pathogen | Bioactive MVOCs not determined | Papaleo et al. (2013), Maida et al. (2015) |
Pseudomonas sp. | Psychrophile | Promotion of wheat germination | N/D | Yarzábal et al. (2018) |
Thermophiles | Production of biofuels | Ethanol | Zuliani et al. (2021) | |
Inhabitants of bioactive filters | Thermophiles | Degradation of common VOCs in pulp and forestry industry | Methanol, α-pinene | Kong et al. (2001) |
Thermophiles | Production of valuable compounds from waste | VFAs, alcohols | Yu and Fang (2001), Yao et al. (2019), Amanidaz et al. (2023) | |
Thermophiles | Genetically engineered to produce compounds of interest | E.g. acetone, 1-butanol | Many examples in the literature. E.g. Keller et al. (2015), Kato et al. (2021) | |
Bacterial and fungal isolates from rhizosphere of xerophytes | Xerophiles | Improvement of plant growth | Camphene, benzyl benzoate, isovalerate, 3-methyl-1-butanol, and others | Camarena-Pozos et al. (2019, 2021) |
Inhabitants of solar salterns | Halophiles | Characteristics of special salt varieties | Carotenoid derivatives | Donadio et al. (2011) |
Halophiles | Production of biofules | Ethanol, butanol | Amoozegar et al. (2019) | |
S. nanhainus | Piezophile | Inhibition of P. aeruginosa and other human pathogens | 2-Hydroxy-iso-butyrophenone; 2, 4, 6-trimethyl-benzaldehyde, 2-ethyl-1-hexanol | Hu et al. (2024) |
Yeast isolates | Halotolerant | Inhibition of strawberry pathogen | N/D | Zou et al. (2021) |
Organism(s) . | Type of extremophiles . | Application . | MVOC . | References . |
---|---|---|---|---|
Pseudoalteromonas sp., Psychrobacter sp. | Psychrophiles | Inhibition of human pathogen | Bioactive MVOCs not determined | Papaleo et al. (2013), Maida et al. (2015) |
Pseudomonas sp. | Psychrophile | Promotion of wheat germination | N/D | Yarzábal et al. (2018) |
Thermophiles | Production of biofuels | Ethanol | Zuliani et al. (2021) | |
Inhabitants of bioactive filters | Thermophiles | Degradation of common VOCs in pulp and forestry industry | Methanol, α-pinene | Kong et al. (2001) |
Thermophiles | Production of valuable compounds from waste | VFAs, alcohols | Yu and Fang (2001), Yao et al. (2019), Amanidaz et al. (2023) | |
Thermophiles | Genetically engineered to produce compounds of interest | E.g. acetone, 1-butanol | Many examples in the literature. E.g. Keller et al. (2015), Kato et al. (2021) | |
Bacterial and fungal isolates from rhizosphere of xerophytes | Xerophiles | Improvement of plant growth | Camphene, benzyl benzoate, isovalerate, 3-methyl-1-butanol, and others | Camarena-Pozos et al. (2019, 2021) |
Inhabitants of solar salterns | Halophiles | Characteristics of special salt varieties | Carotenoid derivatives | Donadio et al. (2011) |
Halophiles | Production of biofules | Ethanol, butanol | Amoozegar et al. (2019) | |
S. nanhainus | Piezophile | Inhibition of P. aeruginosa and other human pathogens | 2-Hydroxy-iso-butyrophenone; 2, 4, 6-trimethyl-benzaldehyde, 2-ethyl-1-hexanol | Hu et al. (2024) |
Yeast isolates | Halotolerant | Inhibition of strawberry pathogen | N/D | Zou et al. (2021) |
Biotechnological applications of extremophiles and their MVOCs reported in the literature. N/D: not determined. VFAs: volatile fatty acids.
Organism(s) . | Type of extremophiles . | Application . | MVOC . | References . |
---|---|---|---|---|
Pseudoalteromonas sp., Psychrobacter sp. | Psychrophiles | Inhibition of human pathogen | Bioactive MVOCs not determined | Papaleo et al. (2013), Maida et al. (2015) |
Pseudomonas sp. | Psychrophile | Promotion of wheat germination | N/D | Yarzábal et al. (2018) |
Thermophiles | Production of biofuels | Ethanol | Zuliani et al. (2021) | |
Inhabitants of bioactive filters | Thermophiles | Degradation of common VOCs in pulp and forestry industry | Methanol, α-pinene | Kong et al. (2001) |
Thermophiles | Production of valuable compounds from waste | VFAs, alcohols | Yu and Fang (2001), Yao et al. (2019), Amanidaz et al. (2023) | |
Thermophiles | Genetically engineered to produce compounds of interest | E.g. acetone, 1-butanol | Many examples in the literature. E.g. Keller et al. (2015), Kato et al. (2021) | |
Bacterial and fungal isolates from rhizosphere of xerophytes | Xerophiles | Improvement of plant growth | Camphene, benzyl benzoate, isovalerate, 3-methyl-1-butanol, and others | Camarena-Pozos et al. (2019, 2021) |
Inhabitants of solar salterns | Halophiles | Characteristics of special salt varieties | Carotenoid derivatives | Donadio et al. (2011) |
Halophiles | Production of biofules | Ethanol, butanol | Amoozegar et al. (2019) | |
S. nanhainus | Piezophile | Inhibition of P. aeruginosa and other human pathogens | 2-Hydroxy-iso-butyrophenone; 2, 4, 6-trimethyl-benzaldehyde, 2-ethyl-1-hexanol | Hu et al. (2024) |
Yeast isolates | Halotolerant | Inhibition of strawberry pathogen | N/D | Zou et al. (2021) |
Organism(s) . | Type of extremophiles . | Application . | MVOC . | References . |
---|---|---|---|---|
Pseudoalteromonas sp., Psychrobacter sp. | Psychrophiles | Inhibition of human pathogen | Bioactive MVOCs not determined | Papaleo et al. (2013), Maida et al. (2015) |
Pseudomonas sp. | Psychrophile | Promotion of wheat germination | N/D | Yarzábal et al. (2018) |
Thermophiles | Production of biofuels | Ethanol | Zuliani et al. (2021) | |
Inhabitants of bioactive filters | Thermophiles | Degradation of common VOCs in pulp and forestry industry | Methanol, α-pinene | Kong et al. (2001) |
Thermophiles | Production of valuable compounds from waste | VFAs, alcohols | Yu and Fang (2001), Yao et al. (2019), Amanidaz et al. (2023) | |
Thermophiles | Genetically engineered to produce compounds of interest | E.g. acetone, 1-butanol | Many examples in the literature. E.g. Keller et al. (2015), Kato et al. (2021) | |
Bacterial and fungal isolates from rhizosphere of xerophytes | Xerophiles | Improvement of plant growth | Camphene, benzyl benzoate, isovalerate, 3-methyl-1-butanol, and others | Camarena-Pozos et al. (2019, 2021) |
Inhabitants of solar salterns | Halophiles | Characteristics of special salt varieties | Carotenoid derivatives | Donadio et al. (2011) |
Halophiles | Production of biofules | Ethanol, butanol | Amoozegar et al. (2019) | |
S. nanhainus | Piezophile | Inhibition of P. aeruginosa and other human pathogens | 2-Hydroxy-iso-butyrophenone; 2, 4, 6-trimethyl-benzaldehyde, 2-ethyl-1-hexanol | Hu et al. (2024) |
Yeast isolates | Halotolerant | Inhibition of strawberry pathogen | N/D | Zou et al. (2021) |
In addition, MVOCs may play a critical role as signalling molecules in extreme environments, akin to their function in nonextreme conditions. They may also contribute to biogeochemical cycles, affecting carbon, nitrogen, and sulphur fluxes. This could be particularly relevant in polar regions, where rising temperatures are expected to thaw vast areas of permafrost soil, reactivating dormant microbial communities, increasing greenhouse gas emissions along with MVOC release (Jiao et al. 2024). These emitted MVOCs could influence atmospheric chemistry and cloud nucleation in these regions (Rinnan 2024). Similarly, decreased precipitation and desertification could reduce microbial activity in the soil including the overall MVOC production, although certain MVOCs may increase under such conditions (Pugliese et al. 2023).
The interest in MVOCs in the field of astrobiology has increased steadily in recent years. The volatility, diversity and, in some cases, chirality of these compounds offers information that could be useful in the search for life on other planets. In particular, MVOCs in Earth’s extreme environments may provide important insights into potentially biogenic volatile compounds in extraterrestrial settings; as reviewed recently (Batty et al. 2025). Moreover, VOCs and their atmospheric effects—such as the formation of secondary organic aerosols—may be detected remotely in extraterrestrial atmospheres using advanced instrumentation (Seager et al. 2016, Arney et al. 2018). Notably, a transmission spectrum from the James Webb Space Telescope recently suggested the presence of dimethyl sulphide in the atmosphere of the planet K2-18 b (Madhusudhan et al. 2023), though this interpretation was later contested (Wogan et al. 2024).
MVOCs produced by extremophiles may also provide valuable insights into the biosphere of Early Earth, as life likely originated in what would now be classified as an extreme environment, such as deep-sea hydrothermal vents (Xiao and Zhang 2014). Indeed, life has likely produced volatile compounds since its earliest stages: a recent metabolic reconstruction of LUCA (the Last Universal Common Ancestor) suggests that it may have synthesized a diverse range of volatile compounds, potentially influencing the atmosphere of Precambrian Earth and serving as a medium for intercellular communication (Ledford and Meredith 2024).
Conclusions and future directions
Much remains unknown about MVOCs in extreme environments (Table 3). Filling these research gaps could have global significance as desertification continues, permafrost soil thaws, and glaciers and ice sheets melt. Many of the MVOCs produced by extremophiles and discussed throughout this review are also produced in nonextreme environments. However, extreme environments often harbour unique microbial communities with distinct metabolic pathways, potentially leading to the discovery of previously unknown MVOCs. Only a small fraction of species has been investigated for their MVOC production, and this production is highly dependent on environmental conditions. Consequently, a substantial number of potential MVOCs remain unidentified (Seager et al. 2016). Untargeted approaches will be a useful first step towards detecting and later identifying potentially novel MVOCs, ultimately expanding our understanding of their ecological roles and biotechnological applications.
Knowledge gaps identified in this review with suggested approaches and recommendations for future research.
Area . | Knowledge gaps . | Possible approaches and improvements . |
---|---|---|
Extremophile adaptations | Uniqueness of specific MVOCs to specific extreme conditions. | Comparison of MVOC profiles across extreme environments with similar extreme conditions. |
Significance of MVOCs as stress indicators in extreme environments. | Characterization of MVOC production by extremophiles subjected to stress (e.g. halophiles in low salinity). | |
MVOC-mediated communication pathways in extreme environments. | Linking MVOC production profiles and species interactions by defined multispecies set-ups in extreme conditions. Modelling of communication networks in extremophilic communities. | |
Environmental biology | Significance of MVOCs in nutrient cycling in extreme environments. | Quantification of N- and S-containing MVOC fluxes. Modelling of MVOC-mediated nutrient and metal fluxes in extreme environments. |
Importance of microbial degradation of MVOCs in extreme environments. | Quantification of MVOC production and degradation in extreme environments. | |
Importance of MVOCs in detoxification of heavy metals in extreme environments. | Quantification of heavy metal-containing MVOCs. | |
Astrobiology | Incomplete inventory of MVOCs in extreme environments. | Use of targeted and untargeted MVOC analysis methods in situ in extreme environments. Characterization of MVOC production by extremophilic isolates. |
Insufficient knowledge on MVOC sampling in extraterrestrial environments (e.g. calibration, sensitivity). | Testing and development of techniques for quantification of MVOCs in extraterrestrial atmospheres and extraterrestrial-like conditions. | |
Biotechnology | Lack of knowledge on biochemical pathways behind production and transformation of MVOCs. | Link MVOC production profiles with (meta)genomics and (meta)transcriptomics analyses in extreme environments. Targeted experiments to identify metabolic pathways. |
Unknown mechanisms underlying the bioactivity of known and unknown MVOCs. Quantification of bioactivity of known and unknown MVOCs produced by extremophiles. | Quantification of MVOC bioactivity and identification of mechanisms of action in extreme environments. | |
Production of known and unknown MVOCs with potential commercial value. | Improvement of MVOC databases to document MVOC-mediated bioactivity, including interactions with target species. |
Area . | Knowledge gaps . | Possible approaches and improvements . |
---|---|---|
Extremophile adaptations | Uniqueness of specific MVOCs to specific extreme conditions. | Comparison of MVOC profiles across extreme environments with similar extreme conditions. |
Significance of MVOCs as stress indicators in extreme environments. | Characterization of MVOC production by extremophiles subjected to stress (e.g. halophiles in low salinity). | |
MVOC-mediated communication pathways in extreme environments. | Linking MVOC production profiles and species interactions by defined multispecies set-ups in extreme conditions. Modelling of communication networks in extremophilic communities. | |
Environmental biology | Significance of MVOCs in nutrient cycling in extreme environments. | Quantification of N- and S-containing MVOC fluxes. Modelling of MVOC-mediated nutrient and metal fluxes in extreme environments. |
Importance of microbial degradation of MVOCs in extreme environments. | Quantification of MVOC production and degradation in extreme environments. | |
Importance of MVOCs in detoxification of heavy metals in extreme environments. | Quantification of heavy metal-containing MVOCs. | |
Astrobiology | Incomplete inventory of MVOCs in extreme environments. | Use of targeted and untargeted MVOC analysis methods in situ in extreme environments. Characterization of MVOC production by extremophilic isolates. |
Insufficient knowledge on MVOC sampling in extraterrestrial environments (e.g. calibration, sensitivity). | Testing and development of techniques for quantification of MVOCs in extraterrestrial atmospheres and extraterrestrial-like conditions. | |
Biotechnology | Lack of knowledge on biochemical pathways behind production and transformation of MVOCs. | Link MVOC production profiles with (meta)genomics and (meta)transcriptomics analyses in extreme environments. Targeted experiments to identify metabolic pathways. |
Unknown mechanisms underlying the bioactivity of known and unknown MVOCs. Quantification of bioactivity of known and unknown MVOCs produced by extremophiles. | Quantification of MVOC bioactivity and identification of mechanisms of action in extreme environments. | |
Production of known and unknown MVOCs with potential commercial value. | Improvement of MVOC databases to document MVOC-mediated bioactivity, including interactions with target species. |
Knowledge gaps identified in this review with suggested approaches and recommendations for future research.
Area . | Knowledge gaps . | Possible approaches and improvements . |
---|---|---|
Extremophile adaptations | Uniqueness of specific MVOCs to specific extreme conditions. | Comparison of MVOC profiles across extreme environments with similar extreme conditions. |
Significance of MVOCs as stress indicators in extreme environments. | Characterization of MVOC production by extremophiles subjected to stress (e.g. halophiles in low salinity). | |
MVOC-mediated communication pathways in extreme environments. | Linking MVOC production profiles and species interactions by defined multispecies set-ups in extreme conditions. Modelling of communication networks in extremophilic communities. | |
Environmental biology | Significance of MVOCs in nutrient cycling in extreme environments. | Quantification of N- and S-containing MVOC fluxes. Modelling of MVOC-mediated nutrient and metal fluxes in extreme environments. |
Importance of microbial degradation of MVOCs in extreme environments. | Quantification of MVOC production and degradation in extreme environments. | |
Importance of MVOCs in detoxification of heavy metals in extreme environments. | Quantification of heavy metal-containing MVOCs. | |
Astrobiology | Incomplete inventory of MVOCs in extreme environments. | Use of targeted and untargeted MVOC analysis methods in situ in extreme environments. Characterization of MVOC production by extremophilic isolates. |
Insufficient knowledge on MVOC sampling in extraterrestrial environments (e.g. calibration, sensitivity). | Testing and development of techniques for quantification of MVOCs in extraterrestrial atmospheres and extraterrestrial-like conditions. | |
Biotechnology | Lack of knowledge on biochemical pathways behind production and transformation of MVOCs. | Link MVOC production profiles with (meta)genomics and (meta)transcriptomics analyses in extreme environments. Targeted experiments to identify metabolic pathways. |
Unknown mechanisms underlying the bioactivity of known and unknown MVOCs. Quantification of bioactivity of known and unknown MVOCs produced by extremophiles. | Quantification of MVOC bioactivity and identification of mechanisms of action in extreme environments. | |
Production of known and unknown MVOCs with potential commercial value. | Improvement of MVOC databases to document MVOC-mediated bioactivity, including interactions with target species. |
Area . | Knowledge gaps . | Possible approaches and improvements . |
---|---|---|
Extremophile adaptations | Uniqueness of specific MVOCs to specific extreme conditions. | Comparison of MVOC profiles across extreme environments with similar extreme conditions. |
Significance of MVOCs as stress indicators in extreme environments. | Characterization of MVOC production by extremophiles subjected to stress (e.g. halophiles in low salinity). | |
MVOC-mediated communication pathways in extreme environments. | Linking MVOC production profiles and species interactions by defined multispecies set-ups in extreme conditions. Modelling of communication networks in extremophilic communities. | |
Environmental biology | Significance of MVOCs in nutrient cycling in extreme environments. | Quantification of N- and S-containing MVOC fluxes. Modelling of MVOC-mediated nutrient and metal fluxes in extreme environments. |
Importance of microbial degradation of MVOCs in extreme environments. | Quantification of MVOC production and degradation in extreme environments. | |
Importance of MVOCs in detoxification of heavy metals in extreme environments. | Quantification of heavy metal-containing MVOCs. | |
Astrobiology | Incomplete inventory of MVOCs in extreme environments. | Use of targeted and untargeted MVOC analysis methods in situ in extreme environments. Characterization of MVOC production by extremophilic isolates. |
Insufficient knowledge on MVOC sampling in extraterrestrial environments (e.g. calibration, sensitivity). | Testing and development of techniques for quantification of MVOCs in extraterrestrial atmospheres and extraterrestrial-like conditions. | |
Biotechnology | Lack of knowledge on biochemical pathways behind production and transformation of MVOCs. | Link MVOC production profiles with (meta)genomics and (meta)transcriptomics analyses in extreme environments. Targeted experiments to identify metabolic pathways. |
Unknown mechanisms underlying the bioactivity of known and unknown MVOCs. Quantification of bioactivity of known and unknown MVOCs produced by extremophiles. | Quantification of MVOC bioactivity and identification of mechanisms of action in extreme environments. | |
Production of known and unknown MVOCs with potential commercial value. | Improvement of MVOC databases to document MVOC-mediated bioactivity, including interactions with target species. |
One of the main challenges in analysing the volatolome of environmental samples is to determine the specific sources of emitted MVOCs (Meredith and Tfaily 2022). Thus, many of the studies discussed in this review estimate in situ MVOC production without further analysis of the associated microbial communities, or they rely on simplified laboratory settings to study the MVOC production by isolated strains and their MVOC-mediated interactions. Such controlled settings may not represent real-world conditions. Additionally, the inherent complexity of natural environments with many interacting factors and fluctuating conditions poses significant challenges for accurately replicating and studying these systems in a laboratory setting. Many studies also rely on qualitative or semiquantitative methods, which provide only relative proportions of MVOCs rather than absolute concentrations. To gain a comprehensive understanding of MVOCs and their environmental interactions, more quantitative studies are needed. Even then, technical hurdles remain, such as detecting MVOCs at low concentrations and distinguishing them from abiogenic VOCs. In the future, comprehensive studies that integrate MVOC measurements with metagenomics, metatranscriptomics, and other high-throughput techniques will be necessary to elucidate the ecological importance of MVOCs for extremophiles. Such research will improve our understanding of the biogeochemical cycling of nutrients and heavy metals, atmospheric chemistry, and could drive biotechnological innovations and advances in astrobiology.
Conflict of interest
The authors have no conflict of interest to declare.
Funding
This project was funded by the Novo Nordisk Foundation under the Novo Nordisk Foundation Interdisciplinary Synergy Program (grant number NNF19OC0057374): effects of bacteria on atmospheres of Earth, Mars, and exoplanets—adapting and identifying life in extraterrestrial environments. This project was also supported by The Danish National Research Foundation within the Center for Volatile Interactions (VOLT, DNRF168).