-
PDF
- Split View
-
Views
-
Cite
Cite
Giulia Oliva, Tobias Sahr, Carmen Buchrieser, Small RNAs, 5′ UTR elements and RNA-binding proteins in intracellular bacteria: impact on metabolism and virulence, FEMS Microbiology Reviews, Volume 39, Issue 3, May 2015, Pages 331–349, https://doi.org/10.1093/femsre/fuv022
- Share Icon Share
Sequencing-based studies have illuminated increased transcriptional complexity within the genome structure of bacteria and have resulted in the identification of many small regulatory RNAs (sRNA) and a large amount of antisense transcription. It remains an open question whether these sRNAs all indeed play regulatory roles, but their identification led to an exponential increase in studies searching for their function. This allowed to show that sRNAs may modulate virulence gene expression, cellular differentiation, metabolic functions, adaptation to environmental conditions and pathogenesis. In this review we will provide mechanistic insights into how sRNAs bind mRNAs and/or proteins. Furthermore, the important roles of the RNA chaperone Hfq, the CsrA system and the CRISPR RNA will be discussed. We will then focus on sRNAs and 5′ untranslated region (UTR) elements of intracellular bacteria like Chlamydia, Listeria, Legionella, or Salmonella, and place emphasis on those that are expressed during replication in host cells and are implicated in virulence and metabolism. In addition, sRNAs that regulate motility, iron homeostasis, and differentiation or stress responses will be highlighted. Taken together sRNAs constitute key elements in many major regulatory networks governing the intracellular life and virulence of pathogenic bacteria.
INTRODUCTION
Pathogenic bacteria have evolved a variety of strategies to replicate in many different niches in their hosts. They have learned how to counteract the host defences and to replicate in specific, normally sterile regions. There are bacteria that remain extracellular and those that are internalized via active or passive pathways (Cossart and Sansonetti 2004). Intracellular bacterial pathogens co-exist with the infected cell in an obligate intracellular state or transit between the extracellular and intracellular environment. However, all have evolved the ability to survive and grow within eukaryotic cells such as professional phagocytes or other cell types by employing diverse mechanisms to manipulate the host cell for their own benefit. After entering the host cell, the bacteria are surrounded by a membrane-bound vacuole that is targeted to the lysosomal compartments to undergo proteolytic degradation. There, the bacteria are facing a changing and very hostile environment characterized by decreasing pH, elevated concentration of reactive oxygen and nitrogen species and poor nutrient content to which either they have to adapt or they have to avoid in order for infection to succeed. Two main strategies are observed: (i) survival in this niche either by preventing vacuole–lysosome fusion or by modifying the environment within the phagolysosome (vacuolar pathogens) like Legionella, Coxiella, Brucella, Mycobacterium and Salmonella; or (ii) escape from the vacuole to gain access to and proliferate within the host cell cytosol (cytosolic pathogens) like Listeria, Shigella, Burkholderia, Francisella and Rickettsia (Haas 2007; Ray, Marteyn, Sansonetti, et al.2009; Fredlund and Enninga 2014).
Adaptation to these intracellular lifestyles is regulated in both space and time. Those bacteria that adapt most rapidly to the hostile conditions encountered in the host are the most successful. The adaptation process is accompanied by major changes in gene transcripts, post-transcriptional regulatory molecules and the protein levels of the bacteria. For intracellular bacteria this includes a temporal and spatial highly co-ordinated regulation of the production of specific effector proteins. These proteins are transferred to the host by dedicated secretory systems and are able to modify the immune response and the metabolism of the infected cell, bringing advantage for the pathogens (Hubber and Roy 2010; Agbor and McCormick 2011; Steiner, Furuya and Metzger 2014). The bacteria sense specific stimuli and respond with changes in gene expression, a process that is tightly controlled by various and complex regulatory networks. Involved therein are factors that are highly conserved in the microbial world including the stringent response alarmone (p)ppGpp (Dalebroux and Swanson 2012), two-component systems (Salazar and Laub 2015), the global regulator CsrA (Romeo, Vakulskas and Babitzke 2013), the RNA chaperone Hfq (Sobrero and Valverde 2012) and the alternative sigma factor RpoS (Lange and Hengge-Aronis 1991; Schellhorn 2014). Key players in these important regulatory networks are small, non-coding RNAs (sRNA). In the last years, genome-wide expression studies using high-density tiling arrays or RNA deep sequencing (RNAseq) have uncovered the wide distribution and large number of non-coding RNAs present in bacterial genomes (Papenfort and Vogel 2010). Studies of their functionality attracted more and more attention, and meanwhile it is evident that they play a crucial role in many biological processes such as in environmental sensing and stress adaptation, virulence and infectivity of intracellular bacteria, as well as development and metabolism (Gottesman and Storz 2011). In this review we will focus on how intracellular pathogens regulate their adaptation to the invaded host, allowing their replication, by discussing the fascinating roles that sRNAs and the two major RNA-binding proteins Hfq and CsrA/RsmA play in these regulatory processes. After describing their mechanisms of function we will illustrate their impact by providing selected examples of their regulatory roles in intracellular bacteria.
MECHANISMS BY WHICH sRNAs FUNCTION IN BACTERIA
Regulatory RNAs in bacteria are usually not translated and comprise a size range between 50 and 400 nucleotides in length. They can modulate transcription, translation, mRNA stability, and DNA maintenance or silencing. These diverse functions are achieved through a variety of mechanisms, including changes in RNA conformation, protein binding, base pairing with other RNAs and interactions with DNA (Waters and Storz 2009; Gottesman and Storz 2011). Due to the recent development and improvement of genome-wide RNAseq methods, there has been an explosion in the amount of sRNAs identified, but the characterization of their function in the diverse regulatory networks is still in its infancy (Sorek and Cossart 2010). The major mechanisms of how sRNAs can act are as follows.
– Trans-encoded sRNA molecules are present on the chromosome in a location distinct from their targets and thus share only limited complementarity with their targets. The general mechanism of trans-acting sRNAs is to sequester the ribosome-binding site (RBS) of a target mRNA by base-pairing to the Shine–Dalgarno (SD) sequence or the start codon and may also interact with the coding sequence of the mRNAs (Storz, Vogel and Wassarman 2011). In order to exploit their regulatory functions, most of the already characterized trans sRNAs are tightly coupled with the activity of RNases, which are enzymes involved in RNA turnover through RNA cleavage (Viegas et al.2007; Viegas et al.2011; Saramago et al.2014). Furthermore, taking into account the limited complementarity between sRNAs and their mRNA targets, many trans-encoded RNA molecules engage the RNA chaperone protein Hfq (Vogel and Luisi 2011) (Fig. 1A).
– Cis-encoded antisense RNAs are complementary to their target RNA as they are transcribed from the DNA strand opposite to the genes they regulate, hence they can interact autonomously. They are often located in the untranslated regions (UTRs) of the corresponding gene where the RNA duplex formation can affect ribosome-binding/translation, termination events or overall stability of the mRNA by rearranging the secondary structures, such as hairpins in the target RNA (Caldelari et al.2013) (Fig. 1B).
– Alternatively to the RNA–RNA regulation, sRNA can also interact with regulatory proteins, influencing their activity directly. The best understood system in this context is the global carbon storage regulator of the CsrA/RsmA family. A common feature in this network present in numerous pathogenic and non-pathogenic bacteria is the transcription of at least one or more sRNAs named CsrBC or RsmYZ, depending on a two-component system (TCS). These sRNAs can interact with CsrA/RsmA and sequester it from its specific position on the target mRNA often in the immediate vicinity of the RBS (Babitzke and Romeo 2007). This leads to the translation of the previously blocked transcripts. Alternatively, it is discussed that CsrA might also have positive effects on the turnover of mRNAs due to stabilization of the target transcript and protection against RNase attacks.
– A more recently described class of sRNAs participates in the clustered regularly interspaced short palindromic repeats/CRISPR associated (CRISPR/Cas) system, which provides the bacteria with an RNA-mediated adaptive immune system against nucleic acids deriving, for example, from bacteriophages, plasmids or mobile genetic elements (Brouns et al.2008; Jinek et al.2014). The variable crRNAs originating from the foreign DNA are located in an array, each of which is flanked by an identical repeat sequence (Fig. 2). Together with the conserved Cas proteins, the crRNA can recognize the complementary DNA target and mediate its degradation.
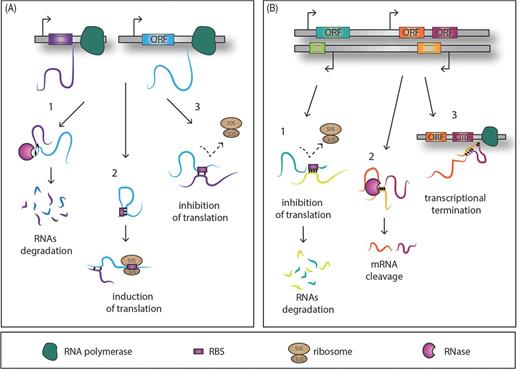
Simplified model of action of trans-encoded and cis-encoded RNAs. (A1) trans-encoded sRNAs interact with their target RNA through imperfect base-pairing and hence promote RNase degradation of the double-stranded RNA molecules. Alternatively the sRNAs might affect the target translation positively (A2) and negatively (A3) by releasing or masking the ribosome-binding site, respectively. Conversely the cis-encoded sRNAs bind through full sequence complementarity the mRNA target, affecting translation, and end in the degradation of the sRNA–target RNA complex (B1). A small cis-encoded RNA, antisense between two genes, can lead to mRNA cleavage (B2) or the transcriptional termination through a putative loop formation and consequently the cessation of the RNA polymerase activity (B3).
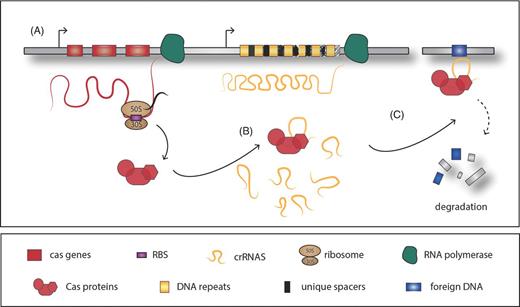
Model of CRISR/Cas system function. (A) Basic structure of the CRISPR/Cas system: several Cas proteins are encoded by the cas genes, located typically in the close proximity of the CRISPR arrays, composed of a variable number of DNA repeats interrupted by unique spacer regions which originate from foreign DNA acquisition. (B) The Cas proteins are in charge of the crRNAs processing and interference with the cDNA region, promoting DNA degradation or silencing (C).
By highlighting some of the recent studies about these different RNA-based regulations in intracellular bacteria we show the remarkable contribution of sRNAs and their broad diversity and functionality in regulatory networks (Table 1).
Examples for the functional diversity of sRNAs in selected intracellular bacteria.
Species . | sRNA . | Property . | Function . | References . |
---|---|---|---|---|
Legionella pneumophila | 6S, 6S2 | RNA polymerase-binding RNA | Required for intracellular replication in macrophages; implicated in stress response and nutrient acquisition | Faucher et al.2010; Weissenmayer et al.2011 |
RsmY, Z, X | CsrA-binding RNA | Implicated in replication in macrophages; regulation of motility | Sahr et al.2009; Rasis and Segal 2009 | |
Cas2-dependent crRNA | CRISPR/Cas system | Implicated in stress response and replication in amoeba | Sahr et al.2009; Gunderson and Cianciotto 2013 | |
Listeria monocytogenes | Anti0677 | RNA excludon | Control of flagellum biosynthesis and motility | Toledo-Arana et al.2009 |
5′ UTR-prfA | RNA thermometer | Control of the major virulence regulator PrfA | Johansson et al.2002 | |
AspoC | RNA B12 riboswitch | Regulation of propanediol utilization | Mellin et al.2013 | |
Rli55 | RNA B12 riboswitch | Regulation of ethanolamine utilization | Mellin et al.2014 | |
LhrA | Hfq-binding RNA | Unknown | Nielsen et al.2010 | |
RliB-CrispR | CRISPR/Cas system | Implicated in virulence | Toledo-Arana et al.2009; Sesto et al.2014 | |
Rli27 | Trans-acting RNA | Implicated in virulence; regulation of the cell wall- associated protein (Lmo0514) | Quereda et al.2014 | |
SreA, SreB | Trans-acting riboswitches | Control of the major virulence regulator PrfA | Loh et al.2009; Toledo-Arana et al.2009 | |
Salmonella enterica serovar Typhimurium | 6S | RNA polymerase-binding RNA | Related to intracellular replication | Ortega, Gonzalo-Asensio and Garcia-del Portillo, et al.2012 |
CsrB,C | CsrA-binding RNA | Control of pathogenesis; required for flagella expression | Jonas et al.2010 | |
IsrM | Trans-acting RNA | Implicated in virulence; required for intracellular replication in macrophages | Gong et al.2011 | |
RybB-1, RybB-2 | Trans-acting RNA | Regulation of oxidative stress response | Calderon et al.2014 | |
lesR-1 | Cis-encoded RNA | Control of the intracellular replication in eukaryotic cell lines; implicated in virulence | Gonzalo-Asensio et al.2013 | |
5′ UTR-agsA | FourU thermometer sensor | Regulation of the small heat shock gene agsA | Waldminghaus, Gaubig and Narberhaus 2007 | |
AmgR | Cis-encoded RNA | Implicated in virulence in mice | Lee and Groisman 2010 | |
Salmonella enterica serovar Typhi | AsdA | Cis-encoded RNA | Regulation of intracellular replication | Dadzie et al.2013 |
RfrA, RfrB | Trans-acting RNA | Regulation of iron homeostasis | Leclerc, Dozois and Daigle 2013 | |
Chlamydia trachomatis | IhtA | Trans-acting RNA | Inhibitor of the histone-like protein Hc1; required for the RB to EB differentiation programmre | Tattersall et al.2012 |
Neisseria meningitidis | NrrF | Trans-acting RNA | Iron homeostasis regulation | Mellin et al.2007, Mellin et al.2010 |
Francisella novicida | Cas9-dependent crRNA, tracrRNA, scaRNA | CRISPR/Cas system | Regulation of endogenous virulence factors | Sampson et al.2013 |
Brucella abortus | AbcR-1, AbcR-2 | Trans-acting RNA | Implicated in virulence in mice; important for survival in macrophages | Caswell et al.2012 |
Coxiella burnetii | 6S | RNA polymerase-binding RNA | Potential implication in regulating intracellular stress response | Warrier et al.2014 |
Mycobacterium tubercolosis | Mcr7 | Trans-acting RNA | Regulation of the TAT secretion system | Solans et al.2014 |
Species . | sRNA . | Property . | Function . | References . |
---|---|---|---|---|
Legionella pneumophila | 6S, 6S2 | RNA polymerase-binding RNA | Required for intracellular replication in macrophages; implicated in stress response and nutrient acquisition | Faucher et al.2010; Weissenmayer et al.2011 |
RsmY, Z, X | CsrA-binding RNA | Implicated in replication in macrophages; regulation of motility | Sahr et al.2009; Rasis and Segal 2009 | |
Cas2-dependent crRNA | CRISPR/Cas system | Implicated in stress response and replication in amoeba | Sahr et al.2009; Gunderson and Cianciotto 2013 | |
Listeria monocytogenes | Anti0677 | RNA excludon | Control of flagellum biosynthesis and motility | Toledo-Arana et al.2009 |
5′ UTR-prfA | RNA thermometer | Control of the major virulence regulator PrfA | Johansson et al.2002 | |
AspoC | RNA B12 riboswitch | Regulation of propanediol utilization | Mellin et al.2013 | |
Rli55 | RNA B12 riboswitch | Regulation of ethanolamine utilization | Mellin et al.2014 | |
LhrA | Hfq-binding RNA | Unknown | Nielsen et al.2010 | |
RliB-CrispR | CRISPR/Cas system | Implicated in virulence | Toledo-Arana et al.2009; Sesto et al.2014 | |
Rli27 | Trans-acting RNA | Implicated in virulence; regulation of the cell wall- associated protein (Lmo0514) | Quereda et al.2014 | |
SreA, SreB | Trans-acting riboswitches | Control of the major virulence regulator PrfA | Loh et al.2009; Toledo-Arana et al.2009 | |
Salmonella enterica serovar Typhimurium | 6S | RNA polymerase-binding RNA | Related to intracellular replication | Ortega, Gonzalo-Asensio and Garcia-del Portillo, et al.2012 |
CsrB,C | CsrA-binding RNA | Control of pathogenesis; required for flagella expression | Jonas et al.2010 | |
IsrM | Trans-acting RNA | Implicated in virulence; required for intracellular replication in macrophages | Gong et al.2011 | |
RybB-1, RybB-2 | Trans-acting RNA | Regulation of oxidative stress response | Calderon et al.2014 | |
lesR-1 | Cis-encoded RNA | Control of the intracellular replication in eukaryotic cell lines; implicated in virulence | Gonzalo-Asensio et al.2013 | |
5′ UTR-agsA | FourU thermometer sensor | Regulation of the small heat shock gene agsA | Waldminghaus, Gaubig and Narberhaus 2007 | |
AmgR | Cis-encoded RNA | Implicated in virulence in mice | Lee and Groisman 2010 | |
Salmonella enterica serovar Typhi | AsdA | Cis-encoded RNA | Regulation of intracellular replication | Dadzie et al.2013 |
RfrA, RfrB | Trans-acting RNA | Regulation of iron homeostasis | Leclerc, Dozois and Daigle 2013 | |
Chlamydia trachomatis | IhtA | Trans-acting RNA | Inhibitor of the histone-like protein Hc1; required for the RB to EB differentiation programmre | Tattersall et al.2012 |
Neisseria meningitidis | NrrF | Trans-acting RNA | Iron homeostasis regulation | Mellin et al.2007, Mellin et al.2010 |
Francisella novicida | Cas9-dependent crRNA, tracrRNA, scaRNA | CRISPR/Cas system | Regulation of endogenous virulence factors | Sampson et al.2013 |
Brucella abortus | AbcR-1, AbcR-2 | Trans-acting RNA | Implicated in virulence in mice; important for survival in macrophages | Caswell et al.2012 |
Coxiella burnetii | 6S | RNA polymerase-binding RNA | Potential implication in regulating intracellular stress response | Warrier et al.2014 |
Mycobacterium tubercolosis | Mcr7 | Trans-acting RNA | Regulation of the TAT secretion system | Solans et al.2014 |
Examples for the functional diversity of sRNAs in selected intracellular bacteria.
Species . | sRNA . | Property . | Function . | References . |
---|---|---|---|---|
Legionella pneumophila | 6S, 6S2 | RNA polymerase-binding RNA | Required for intracellular replication in macrophages; implicated in stress response and nutrient acquisition | Faucher et al.2010; Weissenmayer et al.2011 |
RsmY, Z, X | CsrA-binding RNA | Implicated in replication in macrophages; regulation of motility | Sahr et al.2009; Rasis and Segal 2009 | |
Cas2-dependent crRNA | CRISPR/Cas system | Implicated in stress response and replication in amoeba | Sahr et al.2009; Gunderson and Cianciotto 2013 | |
Listeria monocytogenes | Anti0677 | RNA excludon | Control of flagellum biosynthesis and motility | Toledo-Arana et al.2009 |
5′ UTR-prfA | RNA thermometer | Control of the major virulence regulator PrfA | Johansson et al.2002 | |
AspoC | RNA B12 riboswitch | Regulation of propanediol utilization | Mellin et al.2013 | |
Rli55 | RNA B12 riboswitch | Regulation of ethanolamine utilization | Mellin et al.2014 | |
LhrA | Hfq-binding RNA | Unknown | Nielsen et al.2010 | |
RliB-CrispR | CRISPR/Cas system | Implicated in virulence | Toledo-Arana et al.2009; Sesto et al.2014 | |
Rli27 | Trans-acting RNA | Implicated in virulence; regulation of the cell wall- associated protein (Lmo0514) | Quereda et al.2014 | |
SreA, SreB | Trans-acting riboswitches | Control of the major virulence regulator PrfA | Loh et al.2009; Toledo-Arana et al.2009 | |
Salmonella enterica serovar Typhimurium | 6S | RNA polymerase-binding RNA | Related to intracellular replication | Ortega, Gonzalo-Asensio and Garcia-del Portillo, et al.2012 |
CsrB,C | CsrA-binding RNA | Control of pathogenesis; required for flagella expression | Jonas et al.2010 | |
IsrM | Trans-acting RNA | Implicated in virulence; required for intracellular replication in macrophages | Gong et al.2011 | |
RybB-1, RybB-2 | Trans-acting RNA | Regulation of oxidative stress response | Calderon et al.2014 | |
lesR-1 | Cis-encoded RNA | Control of the intracellular replication in eukaryotic cell lines; implicated in virulence | Gonzalo-Asensio et al.2013 | |
5′ UTR-agsA | FourU thermometer sensor | Regulation of the small heat shock gene agsA | Waldminghaus, Gaubig and Narberhaus 2007 | |
AmgR | Cis-encoded RNA | Implicated in virulence in mice | Lee and Groisman 2010 | |
Salmonella enterica serovar Typhi | AsdA | Cis-encoded RNA | Regulation of intracellular replication | Dadzie et al.2013 |
RfrA, RfrB | Trans-acting RNA | Regulation of iron homeostasis | Leclerc, Dozois and Daigle 2013 | |
Chlamydia trachomatis | IhtA | Trans-acting RNA | Inhibitor of the histone-like protein Hc1; required for the RB to EB differentiation programmre | Tattersall et al.2012 |
Neisseria meningitidis | NrrF | Trans-acting RNA | Iron homeostasis regulation | Mellin et al.2007, Mellin et al.2010 |
Francisella novicida | Cas9-dependent crRNA, tracrRNA, scaRNA | CRISPR/Cas system | Regulation of endogenous virulence factors | Sampson et al.2013 |
Brucella abortus | AbcR-1, AbcR-2 | Trans-acting RNA | Implicated in virulence in mice; important for survival in macrophages | Caswell et al.2012 |
Coxiella burnetii | 6S | RNA polymerase-binding RNA | Potential implication in regulating intracellular stress response | Warrier et al.2014 |
Mycobacterium tubercolosis | Mcr7 | Trans-acting RNA | Regulation of the TAT secretion system | Solans et al.2014 |
Species . | sRNA . | Property . | Function . | References . |
---|---|---|---|---|
Legionella pneumophila | 6S, 6S2 | RNA polymerase-binding RNA | Required for intracellular replication in macrophages; implicated in stress response and nutrient acquisition | Faucher et al.2010; Weissenmayer et al.2011 |
RsmY, Z, X | CsrA-binding RNA | Implicated in replication in macrophages; regulation of motility | Sahr et al.2009; Rasis and Segal 2009 | |
Cas2-dependent crRNA | CRISPR/Cas system | Implicated in stress response and replication in amoeba | Sahr et al.2009; Gunderson and Cianciotto 2013 | |
Listeria monocytogenes | Anti0677 | RNA excludon | Control of flagellum biosynthesis and motility | Toledo-Arana et al.2009 |
5′ UTR-prfA | RNA thermometer | Control of the major virulence regulator PrfA | Johansson et al.2002 | |
AspoC | RNA B12 riboswitch | Regulation of propanediol utilization | Mellin et al.2013 | |
Rli55 | RNA B12 riboswitch | Regulation of ethanolamine utilization | Mellin et al.2014 | |
LhrA | Hfq-binding RNA | Unknown | Nielsen et al.2010 | |
RliB-CrispR | CRISPR/Cas system | Implicated in virulence | Toledo-Arana et al.2009; Sesto et al.2014 | |
Rli27 | Trans-acting RNA | Implicated in virulence; regulation of the cell wall- associated protein (Lmo0514) | Quereda et al.2014 | |
SreA, SreB | Trans-acting riboswitches | Control of the major virulence regulator PrfA | Loh et al.2009; Toledo-Arana et al.2009 | |
Salmonella enterica serovar Typhimurium | 6S | RNA polymerase-binding RNA | Related to intracellular replication | Ortega, Gonzalo-Asensio and Garcia-del Portillo, et al.2012 |
CsrB,C | CsrA-binding RNA | Control of pathogenesis; required for flagella expression | Jonas et al.2010 | |
IsrM | Trans-acting RNA | Implicated in virulence; required for intracellular replication in macrophages | Gong et al.2011 | |
RybB-1, RybB-2 | Trans-acting RNA | Regulation of oxidative stress response | Calderon et al.2014 | |
lesR-1 | Cis-encoded RNA | Control of the intracellular replication in eukaryotic cell lines; implicated in virulence | Gonzalo-Asensio et al.2013 | |
5′ UTR-agsA | FourU thermometer sensor | Regulation of the small heat shock gene agsA | Waldminghaus, Gaubig and Narberhaus 2007 | |
AmgR | Cis-encoded RNA | Implicated in virulence in mice | Lee and Groisman 2010 | |
Salmonella enterica serovar Typhi | AsdA | Cis-encoded RNA | Regulation of intracellular replication | Dadzie et al.2013 |
RfrA, RfrB | Trans-acting RNA | Regulation of iron homeostasis | Leclerc, Dozois and Daigle 2013 | |
Chlamydia trachomatis | IhtA | Trans-acting RNA | Inhibitor of the histone-like protein Hc1; required for the RB to EB differentiation programmre | Tattersall et al.2012 |
Neisseria meningitidis | NrrF | Trans-acting RNA | Iron homeostasis regulation | Mellin et al.2007, Mellin et al.2010 |
Francisella novicida | Cas9-dependent crRNA, tracrRNA, scaRNA | CRISPR/Cas system | Regulation of endogenous virulence factors | Sampson et al.2013 |
Brucella abortus | AbcR-1, AbcR-2 | Trans-acting RNA | Implicated in virulence in mice; important for survival in macrophages | Caswell et al.2012 |
Coxiella burnetii | 6S | RNA polymerase-binding RNA | Potential implication in regulating intracellular stress response | Warrier et al.2014 |
Mycobacterium tubercolosis | Mcr7 | Trans-acting RNA | Regulation of the TAT secretion system | Solans et al.2014 |
THE RNA CHAPERONE HFQ AND ITS INTERACTION WITH TRANS-ENCODED SMALL RNAs
Hfq was identified in Escherichia coli as a host factor required for replication of the RNA phage Qβ > 40 years ago (Franze de Fernandez, Eoyang and August 1968). Hfq is now known as a central mediator of sRNA-based gene regulation in bacteria (Aiba 2007; Waters and Storz 2009; Gottesman and Storz 2011; Storz, Vogel and Wassarman 2011; Vogel and Luisi 2011; Wagner 2013). It is a member of the Sm protein family that is ubiquitous in prokaryotes and in eukaryotes where it is implicated in RNA splicing and decay, suggesting an ancient origin (Moller et al.2002; Wilusz and Wilusz 2005). However, in some cases there is no known Hfq homologue, as in the bacterial clades: Chlamydia– Spirochaetes, Actinomycetes-Deinococcus-Cyanobacteria and Green sulfur bacteria-Cytophagales and in those that have experienced a massive genome reduction due to their parasitic lifestyle, e.g. Buchnera sp., Rickettsia prowazekii and Brucella melitensis (Sun, Zhulin and Wartell 2002; Chao and Vogel 2010).
Briefly, Hfq in association with an sRNA might block the binding of 30S and 50S ribosomal subunits to the RBS of a target mRNA, leading to repression of protein synthesis (Fig. 3A). Conversely, Hfq can bolster protein translation by accompanying the sRNA to the 5′ end of its mRNA target and disrupting a secondary structure which otherwise could mask the RBS and prevent mRNA translation (Fig. 3B). Moreover Hfq can act alone, protecting sRNAs from ribonuclease cleavage by RNase E by binding to many RNase E cleavage sites and prohibiting its degradation in a post-target recognition manner (Vogel and Luisi 2011). On the other hand, Hfq can also stimulate the cleavage of sRNAs and their target mRNAs by directly interacting with RNase E, forming a degradasome-like complex that digests the bound RNA (Hajnsdorf and Regnier 2000; Morita, Maki and Aiba 2005) (Fig. 3C and D). Furthermore, Hfq may induce 3′ end polyadenylation of an mRNA by the enzyme poly(A)polymerase (PAP) and the subsequent 3′ to 5′ degradation by the exoribonucleases, governing the RNA turnover (Fig. 3E). However, it should be mentioned that not all trans-encoded RNAs require Hfq (e.g. RprA or RNAII). These sRNAs free of Hfq binding are preferably degraded by polynucleotide phosphorylase (PNPase) (Andrade et al.2012).
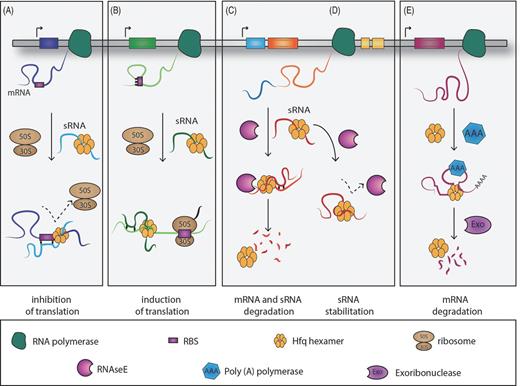
Schematic representation of the mechanisms of action employed by the RNA-binding protein, Hfq. (A) Inhibition at the translational level of the target mRNA by interaction of the sRNA–Hfq complex with the ribosome-binding site (RBS). (B) The complex sRNA–Hfq–mRNA favours the target translation by setting free the RBS, which alternatively would be locked by the formation of a secondary structure. (C) The RNA-binding protein Hfq modulates the mRNA and sRNA turnover rate by recruiting RNase E. (D) Hfq protects the sRNA cleavage site against RNase E attack. (E) Hfq facilitates the mRNA target polyadenylation by the poly(A)polymerase and degradation by exoribonucleases.
Taken together, Hfq is establishing dynamic interactions with a plethora of diverse RNA molecules and affects physiological functions and virulence of bacterial pathogens. Indeed, deletion of hfq led to growth defects and dramatic virulence phenotypes in several intracellular bacteria, such as Francisella tularensis (Meibom et al.2009), Legionella pneumophila (McNealy et al.2005), Neisseria gonorrhoeae (Dietrich et al.2009), S. enterica serovar Typhimurium (Sittka et al.2007) and Listeria monocytogenes (Christiansen et al.2004). Intriguingly, in a growing number of bacteria such as Burkholderia cenocepacia (Ramos et al.2013), multiple Hfq proteins indicating potential functional diversification have been identified. Studies of Hfq in these different organisms will help to uncover its full role in post-transcriptional regulation.
Genome-wide identification of Hfq targets in intracellular bacteria
With the advent of next-generation sequencing (NGS) and comprehensive transcriptome analysis techniques, increasing numbers of new sRNAs have been detected in bacteria (Albrecht et al.2011; Wurtzel et al.2012; Bilusic et al.2014; Boudry et al.2014). Using custom-made microarrays and transcriptome analyses of the intracellular pathogens N. gonorrhoeae (Dietrich et al.2009) or F. tularensis (Meibom et al.2009), it was reported that 6–15% of their genes were affected by the deletion of hfq (Chao and Vogel 2010). For S. enterica serovar Typhimurium, the intracellular pathogen, where Hfq has been studied probably the most using several different techniques, it has been shown that Hfq might directly or indirectly regulate 20% of all Salmonella genes. These genes include those required for host cell invasion, motility, central metabolism, lipopolysaccharide biosynthesis, two-component regulatory systems and fatty acid metabolism (Sittka et al.2008; Ansong et al.2009). Thus Hfq causes diminished fitness and pleiotropic phenotypes and has been shown to be required for virulence of an increasing number of bacterial pathogens.
For many intracellular bacteria, it was reported that Hfq deficiency dramatically impacts on virulence and fitness. For example Hfq is required for the expression of the VirB Type IV secretion system that is indispensable for full virulence of Brucella abortus (Caswell, Gaines and Roop 2012). Hfq is also necessary for the stabilization of two small regulatory RNAs, called AbcR1 and AbcR2 (Table 1). Although single mutants are not defective in intracellular growth in macrophages, double mutants are significantly attenuated. Thus AbcR1 and AbcR2 together are important for survival of B. abortus in macrophages and for virulence in a mouse model of infection. Although less is know about their mechanism of action, AbcR1 and AbcR2 directly regulate gene expression by degradation of the mRNA targets, which may involve the recruitment of a ribonuclease (Caswell et al.2012). These two sRNAs seem to perform redundant and compensatory regulatory functions probably as a consequence of an evolutionary adaption. This is an example where functional redundancy of RNA molecules may ensure the proper expression of genes required for the pathogenic lifestyle and is reminiscent of the CsrA system of, for example, L. pneumophila where the two sRNAs RsmY and RsmZ have a very strong virulence phenotype only when both are missing (Sahr et al.2009).
Furthermore, the Hfq RNA chaperone of L. monocytogenes was shown to contribute to stress tolerance and virulence. Co-immunoprecipitation with L. monocytogenes Hfq followed by sequencing of the Hfq-binding RNA molecules identified initially three novel sRNAs (Christiansen et al.2006). Interestingly, Hfq is dispensable for sRNA-mediated ribo-regulation in most Gram-positive bacteria. However, in the Gram-positive, intracellular pathogen L. monocytogenes there is evidence for Hfq-dependent translational repression. Hfq regulates the expression of the gene lmo0850 in two ways. First, Hfq stabilizes the sRNA LhrA (Table 1), encoded antisense to its target gene, and consequently down-regulates the lm0850 mRNA in an LhrA-dependent manner. Secondly, Hfq facilitates the association of LhrA with its mRNA target, defining a structure amendable for RNA duplex formation. However, the exact biological role of the LhrA sRNAs in L. monocytogenes remains to be characterized (Nielsen et al.2010).
In the last years several approaches have been developed to identify those sRNAs that are interacting with Hfq genome-wide. NGS of RNA enriched by co-immunoprecipitation with chromosomally encoded, epitope-tagged Hfq proteins has been used successfully for several bacteria (Sharma and Voge 2009). The first time it was applied to study sRNAs and mRNAs bound to Hfq of S. enterica serovar Typhimurium it allowed differentiation between transcriptional and post-transcriptional effects of Hfq and definition of the exact sequence of these RNA molecules (Sittka et al.2008). This approach confirmed the previously described Hfq-associated RNAs identified by other techniques but revealed also that a fifth of all Salmonella genes are governed by the action of Hfq, including: the mRNAs of hilD, the master regulator of Salmonella invasion genes; flhDC, the flagellar master regulator; and two sigma factor regulons (Sittka et al.2008). Recently this study was expanded by deep sequencing of Hfq-bound transcripts from multiple stages of growth of S. enterica serovar Typhimurium (Chao et al.2012). This revealed a plethora of new small RNA species from within mRNA loci, and showed that the synthesis of the sRNA DapZ is controlled by HilD. Additionally, this study discovered that an mRNA locus might have a double functional output by producing both a protein and an Hfq-dependent trans-acting RNA. Furthermore, the genome-wide map established for Hfq targets suggested that the 3′ regions of mRNA genes constitute a reservoir of regulatory small RNAs (Chao et al.2012).
Despite the fact that not all bacteria encode an Hfq protein, the high percentage of sRNAs regulated by this RNA-binding protein in many bacteria and several bacterial pathogens led to the explosion of studies aiming not only at identifying Hfq-dependent sRNAs and their targets but also at characterizing their roles to understand the diverse and often species-specific cellular functions and their implications in pathogenesis. However, not all trans-encoded sRNAs need Hfq. As examples of the versatility of trans-encoded sRNAs, Hfq-independent sRNAs and their role in the iron level and life cycle regulation in different intracellular pathogens will be discussed in greater detail in the following paragraphs.
TRANS-ENCODED BACTERIAL sRNAs IMPLICATED IN THE INTRACELLULAR LIFE
Trans-encoded sRNAs respond to iron levels in the host cell
The impact of sRNAs on many aspects of bacterial physiology is known. Here we will concentrate on one important aspect, the regulation of iron levels in the cell. Iron participates in many biological functions and it is essential for bacterial growth but it is toxic at higher concentrations. Thus, iron homeostasis is tightly regulated. A bioinformatics screen identified the small RNA NrrF [for neisserial regulatory RNA responsive to iron (Fe)] in Neisseria meningitidis, which was demonstrated to be both responsive to iron in the environment as encountered in host tissues of fluids and Fur regulated. NrrF has a well-conserved orthologue in Neisseria gonorrhoeae (Mellin et al.2007) (Table 1). In order to monitor the level of iron within the cells, bacteria engage a tight iron-responsive regulation, mediated by the ferric uptake repressor Fur, an iron-dependent transcriptional repressor. In N. meningitidis Fur can indirectly activate gene expression by repressing the regulatory sRNA NrrF. NrrF is able to bind to limited complementarity regions of the sdh transcripts and down-regulate the expression of the sdhA and sdhC genes, encoding subunits of the succinate dehydrogenase complex. Thus, the sRNA NrrF regulates Fur-dependent, iron-activated gene transcription in N. meningitidis. Interestingly, the Fe-regulated sRNA RyhB, first characterized in E. coli (Masse and Gottesman 2002; Masse, Escorcia and Gottesman 2003; Masse, Vanderpool and Gottesman 2005), needs the binding of Hfq to exploit its function. In contrast, the NrrF-mediated iron regulation of the sdhA and sdhC genes in Neisseria does not require the chaperone Hfq (Mellin et al.2010).
Two RybB homologues, named RfrA and RfrB, predicted in Salmonella enterica serovar Typhi are important for optimal intracellular replication in macrophages (Leclerc, Dozois and Daigle 2013) (Table 1). Furthermore, Fur, a repressor of both of these sRNAs, was shown to play a role in phagocytosis and intracellular survival of S. Typhi in human macrophages. Interestingly, loss of either RfrA or RfrB resulted in distinct phenotypes, with respect to siderophore production, salmochelin production, or fur expression in low iron conditions, suggesting a non-redundant role for these regulatory RNAs (Leclerc, Dozois and Daigle 2013). Similarly, deletion of either rfrA or rfrB did not affect bacterial uptake, survival or replication; however, deletion of both sRNAs resulted in a significantly reduced intracellular replication ability, suggesting a complementary role for these two sRNAs for intracellular replication (Leclerc, Dozois and Daigle 2013). Indeed, RfrA and RfrB were induced inside THP-1 macrophages (Leclerc, Dozois and Daigle 2013), inside murine macrophages (Padalon-Brauch et al.2008) and inside fibroblasts (Ortega, Gonzalo-Asensio and Garcia-del Portillo 2012). In addition to their central role in iron homeostasis regulation, these two sRNA molecules and their homologues have additional functions such as protecting against oxidative stress, bactericidal antibiotics and acid resistance (Kim and Kwon 2013). Indeed, it was shown that the homologous sRNAs in S. enterica, named RyhB-1 and RyhB-2, are controlled by OxyR and are important for the oxidative stress response of this pathogen (Calderon et al.2014) (Table 1). This suggests that multiple bacterial pathogens that share these conserved sRNAs may employ a similar mechanism to regulate iron and/or oxidative stress within the host cells.
Trans-encoded sRNAs regulate intracellular differentiation of Chlamydia trachomatis
Another example of regulation of important functions of intracellular life by trans-encoded sRNAs is found in the obligate intracellular bacterium Chlamydia trachomatis. This pathogen employs the sRNA IhtA (inhibitor of hctA translation) to regulate its differentiation processes (Grieshaber et al.2006) (Table 1). IhtA is a trans-encoded RNA, which is implicated in controlling the differentiation from the replicative reticulate bodies (RBs) to the infectious elementary bodies (EBs). IhtA is highly expressed in the replicative phase where it represses the translation of the hctA gene encoding the histone-like protein Hc1. Hc1 binds to and compacts the bacterial chromosome, making the EBs transcriptionally and translationally inactive. In contrast, as soon as IhtA transcription decreases during the late infectious phase, the hctA transcript is highly expressed, allowing the pathogen to transit and differentiate to EBs, where it endures in a metabolically inert stage (Grieshaber et al.2006; Tattersall et al.2012; Ortega et al.2014). Thus, the sRNA IhtA is part of a global regulatory circuit that controls differentiation of RBs to EBs during the chlamydial life cycle. Recently it was shown that regulation of HctA by IhtA is a conserved mechanism found across pathogenic chlamydial species (Tattersall et al.2012).
Trans-encoded sRNAs are implicated in pathogen entry into and replication within host cells
The advances in RNA sequencing and the possibility to undertake these analyses in infection-relevant conditions led to the discovery of several sRNAs implicated in virulence in cellular and animal infection models. An excellent example is the Rli27 sRNA that was identified by whole-genome tiling array analyses as a sRNA induced in L. monocytogenes in vivo (Toledo-Arana et al.2009) (Table 1). Further functional analysis showed that Rli27 mediates the regulation of the cell wall-associated protein, Lmo0514 during the intracellular infection cycle (Quereda et al.2014). Interestingly, in response to environmental cues sensed in the eukaryotic intracellular niche, distinct promoters located upstream of the lmo0514 gene generate two alternative lmo0514 transcript isoforms containing 5′ UTRs of different length. These two transcript isoforms, which display two distinct target sites within the 5′ UTR for sRNAs, are differentially expressed in intracellular and extracellular bacteria. During the intracellular infection cycle, the long 5′ UTR lmo0514 transcript isoform, bearing the Rli27-binding site, is targeted by the Rli27 sRNA, leading to the release of the occluded SD sequence and allows therewith Lmo0514 protein production. Thus, L. monocytogenes promotes Rli27-mediated post-transcriptional regulation of its specific target, a cell wall-associated protein Lmo0154, only in response to its entry into eukaryotic cells (Quereda et al.2014).
Another sRNA-dependent temporal and tissue-specific regulation of virulence has been reported in the S. enterica serovar Typhimurium pathogenicity islands (SPIs) (Gong et al.2011). This sRNA, called IsrM, is expressed in vitro under conditions comparable with those during infection in the gastrointestinal tract (Table 1). Moreover, in vivo assays revealed a higher expression of IsrM in the ileum, suggesting an involvement of this sRNA in Salmonella pathogenicity. Indeed, IsrM targets the SPI-effector SopA and the global regulator of the SPI-1 genes, HilE. HilE is the major regulator of Salmonella virulence crucial for bacterial evasion. Specifically IsrM targets the 5′ UTRs and masks the nearby SD sequence of both hilE and sopA mRNAs, hindering the production of the two proteins. Thus, Salmonella probably uses the fine-tuned expression of IsrM as a mechanism to regulate specifically SPI-1 protein expression in vivo temporally and spatially.
REGULATORY 5′ UTR ELEMENTS
Two diverse classes of 5′ UTRs of mRNAs have gained recent attention: RNA thermometers and RNA riboswitches. To some extent, these two types of regulatory molecules share common features but, most importantly, both are complex RNA molecules that sense a particular chemical or physical signal and accordingly alter their conformation to control the expression of downstream genes. 5′ UTR elements are another striking example of a refined RNA-dependent mechanism, allowing bacteria to respond rapidly and cost-effectively to physical alterations. Riboswitches are regulating the use of different nutrient sources that seem to be important for growth of bacterial pathogens inside host cells. Thermosensors may allow a pathogen to recognize the host environment by sensing the temperature shift encountered when extracellular bacteria invade host cells to replicate intracellularly. An example is L. monocytogenes (discussed below) that regulates virulence gene expression indispensable for intracellular growth in response to temperature by a thermosensor.
RNA thermometers
RNA thermometers control gene expression by temperature-induced conformational changes. All cellular processes are temperature dependent, but virulence genes, cold shock genes and heat shock genes are particularly disposed to thermoregulation. Specifically, the response to an increase in temperature is often used by pathogenic bacteria when they enter their mammalian host. The temperature of 37°C causes induction of virulence genes whose products are only needed in the host environment. The advantage of regulation via RNA thermometers lies in the fact that they respond to temperature changes in a more immediate manner because they control the translation of already existing or nascent mRNAs (Kortmann and Narberhaus 2012). Despite the fact that cis-acting regulatory 5′ UTR elements modulate gene expression either at the transcriptional or translational level (Mandal and Breaker 2004), all RNA thermometers described so far act at the level of translational initiation. In most of the cases, the SD region and/or the initiation codon are involved in base-pairing interactions forming a secondary structure at low temperature. An increase in temperature facilitates the mRNA–ribosome interaction after the full liberation of the SD sequence and the AUG start codon, leading to the formation of the translation initiation complex (Narberhaus, Waldminghaus and Chowdhury 2006). The first RNA thermometer described controls the expression of the cIII protein and also governs the development of phage λ. Strikingly, it represents the only thermometer which turns on the translation of the cIII protein when the temperature is decreased and, furthermore, it does not operate by gradual melting but switches between two exclusive conformations (Altuvia et al.1989). However, RNA thermometers have little or no sequence conservation and are thus difficult to predict from genome sequences. Therefore, the bioinformatics prediction has remained a major challenge (Waldminghaus, Gaubig and Narberhaus 2007). The mechanisms of function of the different RNA thermometers known to date have recently been reviewed in great detail (Kortmann and Narberhaus 2012), and thus here we will give a brief overview of those that are implicated in regulatory processes in intracellular bacteria.
A specific family of RNA thermometers is characterized by the presence of a short sequence motif composed of four uridines, also known as fourU. The fourU element is located at the 5′ UTRs of several virulence and heat shock genes and pairs particularly with AGGA in the SD sequence. FourU elements have been identified and characterized in several pathogenic bacteria such as the lcrF thermometer in Yersinia pestis, the agsA fourU thermometer sensor of Salmonella Typhimurium and the prfA sensor of the intracellular pathogen L. monocytogenes (Waldminghaus et al.2007; Johansson et al.2002; Bohme et al.2012). PrfA of L. monocytogenes, the master regulator of virulence gene expression, is expressed at 37°C, the temperature of mammalian host cells, but not at lower temperatures that L. monocytogenes encounters mainly when growing extracellularly in the environment. Indeed the 5′ UTR of the prfA mRNA adopts a stable stem–loop structure at low temperature, thereby blocking the SD sequence and preventing binding of the ribosome (Table 1). At 37°C, the temperature encountered when infecting humans, the stem–loop melts into an alternative secondary structure, allowing the ribosome to access the SD sequence, leading to the translation of the prfA mRNA and to the subsequent induction of a number of virulence genes (Johansson et al.2002; Johansson and Cossart 2003). Another fourU RNA thermometer was identified in the 5′ UTR of the small heat shock agsA gene in S. enterica serovar Typhimurium. The 5′ UTR of the agsA gene, which codes for a small heat shock protein, consists of only two stem–loop structures, one of which contains the fourU motifs with imperfect complementarity to the SD sequence. As all RNA thermometers, the stability of this structure was calculated to decrease with increasing temperatures, thereby leading to a high level of agsA expression and subsequent availability of the small heat shock protein (Waldminghaus, Gaubig and Narberhaus 2007).
RNA riboswitches regulating metabolic function during intracellular growth
Riboswitches are widespread cis-acting RNA elements in mRNAs that sense metabolites and modulate transcription or translation of mRNAs in response to these metabolites by forming alternative metabolite-free and metabolite-bound conformations (Serganov and Nudler 2013; Peselis and Serganov 2014). In prokaryotes, most of the riboswitches are at the 5′ UTR of the main coding region of a particular mRNA and typically consist of two distinct functional domains. The evolutionarily conserved aptamer element serves as the ligand-binding domain (sensor) whereas a variable sequence, termed the expression platform, is responsible for exerting an influence on the downstream coding sequences, regulating their expression (Serganov and Nudler 2013). Riboswitches can control a broad range of genes including those involved in the biosynthesis and transport of prokaryotic metabolites such as amino acids, nucleotides, metal ions and cofactors (Henkin 2008). Therefore, to some extent the ligand represents not only the stimulus but also the final product of the biosynthetic pathway regulated by the downstream genes of the riboswitch, leading to a feedback control.
Riboswitches have been extensively studied in the last decade and many classical ones have been identified in prokaryotes. However, recently, the first ribsowitch controlling the transcription of non-coding RNAs was described in the intracellular pathogen L. monocytogenes (Mellin et al.2013; Mellin et al.2014). In these studies two vitamin B12-binding (B12) riboswitch-dependent mechanisms were identified that control the catabolism of propanediol and ethanolamine molecules (Mellin et al.2013; Mellin et al.2014). They showed that the B12 riboswitch was transcribed as part of a non-coding antisense RNA, named AspoC, and surprisingly was located downstream and in the antisense orientation of the adjacent gene, pocR (Table 1). PocR is a transcriptional factor that is activated by propanediol, a molecule produced in the intestine by commensal bacteria as a byproduct of the fermentation of rhamnose and fucose. Propanediol catabolism requires a B12-dependent diol dehydratase encoded by the pduCDE genes whose expression is induced by the transcriptional regulator PocR (Bobik et al.1997). In the absence of B12, the riboswitch induces the formation of an aspocR long transcript, which in turn inhibits in trans pocR expression. Furthermore it was suggested that this regulation relies on binding of AspocR to pocR mRNA. As such, the pdu genes are not expressed when vitamin B12 is not available. In contrast, low B12 conditions partially repress pocR expression and appear sufficient to induce the expression of the B12 biosynthesis genes; however, to induce the pdu gene expression maximally, accumulation of vitamin B12 is required. In response to sufficient accumulation of B12 and upon the ligand–aptamer interaction, the riboswitch leads to the production of a short aspocR truncated transcript that does not prevent the expression of the pocR mRNA, whose product enables propanediol catabolism. Thus, the B12 riboswitch does not operate in a black-or-white switch but acts more as a fine-tuning regulatory device. Markedly, regulation by the B12 riboswitch-dependent AspocR allows the propanediol catabolism pathway to be highly activated only when both propanediol and B12 are available (Mellin et al.2013).
Furthermore, in L. monocytogenes, vitamin B12 is also required for the utilization of ethanolamine (Mellin et al.2014). Ethanolamine, like the closely related propanediol molecule, is an important nutrient source for pathogens during infection (Buchrieser et al.2003). Recent studies suggested that propanediol and ethanolamine both play a role in L. monocytogenes pathogenesis as L. monocytogenes up-regulates the pdu and ethanolamine utilization (eut) genes during intracellular growth in intestinal epithelial cells (Joseph et al.2006), and in the intestine of germ-free mice pre-treated with lactobacilli (Archambaud et al.2012). Related to these findings, an in-depth whole-genome transcriptome analysis revealed the presence of a B12 riboswitch upstream of the first gene of the eut locus, coding for the ethanolamine utilization pathway (Toledo-Arana et al.2009). Indeed, according to the availability of the B12 cofactor, the riboswitch modulates eut gene expression through the production of a long or a short truncated sRNA transcript, called Rli55 (Table 1). Furthermore, four ANTAR (AmiR and NasR transcriptional antiterminator regulator) elements were found upstream of the eutA and eutV genes, and two in the Rli55 locus, which are transcribed as part of the 3′ end of the Rli55 transcript. Very elegant experiments showed that in the presence of ethanolamine but absence of the B12 cofactor, a long Rli55 together with the first ANTAR element is transcribed to sequester the two-component response regulator EutV, which consecutively leads to the down-regulation of the eut genes. Conversely, the accumulation of B12 and the successive interaction with the riboswitch triggers the production of a truncated Rli55 transcript lacking the first ANTAR element, which prevents binding to the EutV regulator. As such, the EutV protein co-ordinates the activation of the eut genes, leading to the utilization of ethanolamine (Mellin, Koutero, Dar, 2014). Thus, these studies highlighted a new, non-classical role for regulatory sRNAs, which is sequestering of a two-component system element dependent on a B12 riboswitch.
Recently trans-regulatory riboswitch elements that can function as sRNAs and link virulence and nutrient availability have been discovered in L. monocytogenes (Loh et al.2009). Listeria monocytogenes harbours seven putative S-adenosylmethionine (SAM) riboswitches that may, when SAM binds, form a termination structure that terminates transcription and inhibits synthesis of the downstream mRNA (Toledo-Arana et al.2009). In L. monocytogenes, two riboswitches, named SreA and SreB, have been shown to function in trans and to act as sRNAs controlling expression of PrfA, the major regulator of virulence gene expression (Table 1). Elegant in vitro and in vivo experiments suggest a non-coding RNA function for SreA and SreB. They bind to the 5′ UTR of prfA, and thereby reduce prfA transcript stability and/or prfA mRNA translation. Thus, both SreA and SreB act together to control expression of prfA and thus the virulence of this intracellular pathogen. The identification of many diverse riboswitches, facilitated by the rapid expansion of computational data analysis together with experimental analyses will reveal new mechanisms employed by bacteria to regulate their transcriptional response when growing inside host cells.
CIS-ENCODED SMALL RNAS
In contrast to the trans-encoded sRNAs, the cis-encoded sRNAs are located in the antisense strand of their target RNA regions and their mechanism of action leads to the post-transcriptional down-regulation of the target gene(s) through a high degree of sequence complementarity. In general, this class of sRNAs does not require the help of the RNA-binding protein Hfq (Caldelari et al.2013). However, some cis-encoded sRNAs may also stabilize their mRNA target, such as, for example, the AsdA sRNA discussed below. Although the first described cis-antisense RNAs were mobile elements such as phages, transposons and plasmids (Wagner and Simons 1994), few chromosomally encoded cis-antisense transcripts had been reported by 2007 (Brantl 2007). With the use of tiling arrays and RNAseq to analyse the transcriptional landscape of a bacterial genome widely and at a high level of depth, antisense transcription that was thought to be simply an irregularity turned into a rule. Antisense sRNAs (asRNAs) are present in Gram-positive and Gram-negative bacterial species, but a high variability in their prevalence and genomic density was reported (Georg and Hess 2011; Raghavan, Sloan and Ochman 2012; Lybecker et al.2014). Thus new attention to asRNAs within the bacterial kingdom emerged. AsRNAs can be classified according to their position as internally located, 5′-overlapping or 3′-overlapping asRNAs, or according to their size as short asRNAs (ranging from 100 to 300 nucleotides) or as long asRNAs reaching several kilobases in size (Georg and Hess 2011). However, the lack of functional characterization of many of the identified asRNAs hinders their annotation according to their role in cells. To date, asRNAs have been reported to act at the level of transcription, mRNA stability or translation. Their diverse regulatory mechanisms, asRNA regulation has been reviewed recently in several articles (Thomason and Storz 2010; Georg and Hess 2011). We will thus concentrate here on a particular asRNA mechanism newly described in Listeria, the excludon, and asRNAs important for Salmonella replication.
A new concept in antisense RNA-mediated regulation: the excludon
Recently a sophisticated asRNA-dependent mechanism that achieves a regulatory connection between neighbouring genes that often have opposite functions was reported in L. monocytogenes. The name ‘excludon’ has been coined to describe this new regulatory mechanism. A single transcript serves in parallel as an antisense repressor of one gene or group of genes and as an mRNA promoting the expression of the adjacent genes. This results in the regulation of adjacent genes of opposite functions (Sesto et al.2013).
The ‘excludon’ in L. monocytogenes takes part in the control of flagellum biosynthesis. The asRNA, Anti0677 has a proximal region that is antisense to the lmo0675–lmo0676–lmo0677 locus and a distal region that is part of the gene encoding the regulator MogR (Toledo-Arana et al.2009) (Table 1). The lmo0676–lmo0677 locus encodes FliP and FliQ that together with FliR (encoded by the adjacent gene lmo0678) constitute the flagellum export apparatus. The MogR regulator binds the fli operon promoter and down-regulates the flagellum and thus motility in L. monocytogenes. When Anti0677 is expressed, it inhibits the synthesis of lmo0675 (its function is not known yet) and the entire flagellum export apparatus and leads simultaneously to the expression of MogR. Thus the flagellum apparatus is concurrently turned off by the increasing expression of MogR and by the repression of the lmo0676–lmo0677 locus driven by the asRNA Anti0677. Furthermore, the inhibition of the flagellum export apparatus is likely to involve the formation of a double-stranded RNA when Anti0677 is transcribed and the subsequent cleavage by RNase III (Wurtzel et al.2012). The above-described asRNA Anti0677 was the first example for the excludon concept; however, at least 13 additional, putatively excludon-like regulated genomic loci were detected through comparative transcriptomic analysis of L. monocytogenes, suggesting that excludon-mediated regulation might represent a frequent mechanism. Furthermore, similar organizations have been found in other bacteria such as L. pneumophila (unpublished data), thus this mechanism of antisense regulation is probably not restricted to the genus Listeria but extends to other bacteria.
Cis-encoded sRNAs: regulation of replication
Although transcription of asRNAs occurs genome-wide in many bacteria, only a small fraction of asRNAs is shared across species. Moreover, the promoters associated with asRNAs show no evidence of sequence conservation between, or even within, species. Thus researchers are questioning whether these asRNAs are all functional or not (Raghavan, Sloan and Ochman 2012). Further functional analyses and in-depth characterization of these asRNAs will be necessary to answer this open question. For example, one of the cis-encoded RNAs, termed AsdA, was characterized in S. enterica serovar Typhi where it was shown to be involved in regulating the replication process (Table 1). The AsdA sRNA is located in the complementary strand to the dnaA gene that encodes a site-specific DNA-binding protein (Dadzie et al.2013). DnaA serves as a bacterial replication initiation factor and is capable of recognizing the origin of replication (oriC), mediating the open complex formation, and therefore it induces the assembly of the DNA replication machinery. Moreover DnaA acts as a transcriptional factor, promoting the transcription of target genes (Messer and Weigel 2003). Non-coding RNA elements are often transcribed in response to specific conditions. Indeed, the AsdA sRNA is highly expressed during the stationary growth phase after growth in rich medium but it is already expressed in very early exponential growth when iron limitation or osmotic stresses are exerted. In these conditions, the expression of the AsdA antisense transcript enhances the stability of the dnaA mRNA, which in turn increases its translational rate (Dadzie et al.2013). These observations indicated that the AsdA sRNA, through stabilization of the dnaA mRNA, is a crucial regulator of DNA replication in S. enterica serovar Typhi.
Cis-encoded sRNAs: regulation of virulence gene expression
Interestingly, a long asRNA of 1.2 kb, named AmgR, was identified in the antisense strand of the mgtCBR operon and complementary to the 5′ terminus of this polycistronic mRNA in S. enterica serovar Typhimurium (Table 1). AmgR is transcribed from a promoter that is located in the intergenic region between the mgtC and mgtB genes. The aforementioned operon encodes the MgtC protein implicated in Mg2+ homeostasis and virulence, the Mg2+ transporter MgtB and the 30 amino acid peptide MgtR that mediates degradation of MgtC by the FtsH protease. Intriguingly, the transcription of the polycistronic mgtCBR mRNA and the AmgR sRNA is under the positive control of the two-component regulatory system PhoP/PhoQ. In detail, when PhoQ senses low extracytoplasmatic Mg2+, it phosphorylates PhoP, which then binds to the mgtC and amgR promoters, mediating the transcription of the mgtCBR mRNA and the long sRNA. As such, the long RNA regulatory element limits the MgtC and MgtB protein levels, promoting the MgtR binding to the MgtC protein and the subsequent degradation by the FtsH protease. Markedly, AmgR serves as a timing device to attenuate virulence in mice mediated by the sense-encoded MgtC protein (Lee and Groisman 2010). The finding that the AmgR asRNA is located inside a polycistronic region revealed that the repertoire of regulatory sRNA elements might be larger than thought and raises the possibility that additional asRNAs exist which have been overlooked.
Another example of an asRNA, named lesR-1, encoded within the pSLT virulence plasmid has been reported recently in the bacterial pathogen S. enterica serovar Typhimurium (Gonzalo-Asensio et al.2013) (Table 1). RNA expression has been studied using oligonucleotide microarrays containing probes in each of the two strands of every intergenic region testing a variety of culture conditions. Remarkably, lesR-1 was preferentially expressed in non-growing dormant bacteria during colonization of fibroblasts. The deletion of this asRNA not only influenced the control of bacterial growth within the fibroblast cell lines but also impaired virulence in a mouse infection model. As an asRNA that is overlapping the PSLT047 transcript, the lesR-1 regulatory mechanism involves the direct interaction of the 3′ end of the RNA molecule leading to the subsequent remodelling of the PSLT047 protein levels. Given the virulence phenotype, this suggests that PSLT047 is important for the intracellular lifestyle of S. enterica serovar Typhimurium (Gonzalo-Asensio et al.2013).
DYNAMIC EXPRESSION CHANGES OF sRNAs DURING INTRA- AND EXTRACELLULAR GROWTH
The use of whole-genome tiling arrays and RNA deep sequencing has revealed that sRNAs, similar to protein-coding genes, show dynamically changing expression profiles during growth in laboratory media but also during infection. An example is L. pneumophila as this intracellular pathogen is known to have a pronounced biphasic life cycle. Schematically, pathogenic L. pneumophila encode one set of genes dedicated to transmission that includes all major virulence traits and another set that promotes replication in phagocyte vacuoles, a characteristic that is reflected in a major shift of gene expression during the switch from replicative to transmissive bacteria (Molofsky and Swanson 2004; Bruggemann et al.2006). Recently, RNAseq analyses showed that ∼700 sRNAs are expressed in the L. pneumophila genome. Similar to the protein-coding genes, >60% of the sRNAs are growth phase dependently regulated (Sahr et al.2012). Most interestingly, transcriptional start site mapping identified in addition a high number of sRNAs with tandem promoters, 30% of which are used growth phase dependently (Sahr et al.2012). These strong changes in sRNA expression profiles indicate an important role for sRNAs in regulating the biphasic life cycle of L. pneumophila and thus virulence.
Similarly, transcriptome data using a 70mer oligonucleotide array of the S. enterica Typhimurium genome revealed that ∼2% (98 genes) of all genes are differentially expressed in non-growing intracellular bacteria, when the fibroblast infection model was used (Nunez-Hernandez et al.2013). Later work also identified sRNAs that are differentially expressed. As an example, SraL shows higher expression levels in non-growing bacteria than in actively growing ones (Ortega, Gonzalo-Asensio and Garcia-del Portillo 2012).
A comprehensive picture of growth phase- and condition-dependent expression of sRNAs was reported for L. monocytogenes. The analyses of L. monocytogenes expression profiles using tiling arrays and bacteria grown in exponential phase, stationary phase, hypoxia and low temperature or isolated from the intestine of axenic mice or bacteria grown in the blood of human donors provided important information about their expression conditions and thus hints of their putative functions (Toledo-Arana et al.2009). Similarly, RNA sequencing of L. monocytogenes grown in macrophages showed that 29 of the 150 described sRNAs are specifically induced during intracellular growth, pointing to functional roles during infection. Indeed, knockout mutants of three of these RNAs, named rli31, rli33-1 and rli50, were defective in intracellular growth (Mraheil et al.2011). Recently the non-coding genome was characterized in multiple growth conditions that are relevant for the infection process using RNA deep sequencing. This led not only to the characterization of condition-dependent expression of sRNAs but also to the identification of new regulatory mechanisms such as the excludon described above (Wurtzel et al.2012).
Using a combined experimental and in silico approach, 34 sRNAs have been identified in Mycobacterium bovis BCG, many of which are responsive to changing environmental growth conditions. Homologues of several of these sRNAs are also present in Mycobacterium tuberculosis and Mycobacterium smegmatis (DiChiara et al.2010). Recently one of these, the sRNA homologous to Mcr7, has been functionally characterized in M. tuberculosis. To analyse the regulon of PhoPR, a two-component system essential for virulence of M. tuberculosis, a ChIPseq analyses using PhoP antibodies was undertaken. This allowed identification of all directly PhoP-regulated genes, but also a PhoP-regulated sRNA named Mcr7. In elegant experiments, it was shown that Mcr7 modulates the TAT secretion system of M. tuberculosis and impacts its activity. This leads to a diminished secretion of the immunodominant AG85 complex, implicated in successful infection of the host (Solans et al.2014). Taken together, sRNAs show dynamic changes of expression according to the conditions in which they are functioning and many are crucial contributors to the virulence process of intracellular bacteria.
PROTEINS REGULATING SMALL RNAs
RNA-binding proteins are major players in the regulation of gene expression. Certain of these proteins involve an association with sRNAs. One of the best known is the 6S RNA that interacts with σ70-containing RNA polymerase and regulates transcription at specific promoters and that recently has also been shown to be involved in virulence regulation of intracellular bacteria. Another well-known system present in many bacteria is the CsrA/RsmA protein, whose activity is regulated by sRNAs.
The RNA polymerase-interacting 6S RNA
First identified in E. coli in the late 1960s, the 6S RNA is a double-stranded sRNA transcribed by the ssrS gene and ubiquitously expressed, with a remarkable accumulation during the late stationary phase of the bacterial growth cycle (Hindley 1967; Wassarman and Storz 2000). The conserved secondary structure consisting of two irregular stem structures and a single-stranded central bulge has led to the prediction of the presence of this sRNA in >100 bacterial species so far (Barrick et al.2005). Although the characterization and the identification of physiological functions of 6S RNA remained elusive for three decades as no appreciable growth phenotype in cells depleted or overexpressing 6S RNA was observed, the conserved secondary structure suggested an important role for this sRNA. The first time, a detectable phenotype was observed when E. coli was grown under stringent stress and limiting nutrient conditions, suggesting that the bacteria, under these conditions, engage 6S RNA to allocate the nutrients (Trotochaud and Wassarman 2004). Strikingly, the 6S RNA is the first sRNA that was shown to alter gene expression by binding directly to the active site of the housekeeping holoenzyme form of the RNA polymerase, the σ70-RNA polymerase, to hinder the access to the promoter DNA. In E.coli it was shown that the 6S RNA contributes to the regulation of a large number of genes, by inhibiting their transcription during the late stationary phase (Wassarman and Storz 2000; Willkomm and Hartmann 2005). The specificity of the binding strictly resides in the conserved secondary structure and in the internal stem bulge region of the 6S RNA (Trotochaud and Wassarman 2004). Secondly, further investigations on the nature of the secondary structure of the 6S RNA revealed a remarkable resemblance to the conformation of DNA, suggesting a shared mechanism of binding. Additional structural requirements, related to the 6S-dependent inhibition, involve the presence of a promoter with an extended –10 element and/or a weak –35 element (Cavanagh, Sperger and Wassarman 2012). Furthermore, Wassarman and Saecker showed that 6S RNA can be used as a template for RNA synthesis during the stationary phase, generating a 14–20 nucleotide long RNA product, named pRNA (Wassarman and Saecker 2006). The synthesis of the pRNA seems to be required to induce the release of the 6S–RNA polymerase complex, which consequently would lead to the formation of the 6S RNA–pRNA duplex. Thus, during outgrowth, the inhibitory machinery is switched off when necessary and the 6S RNA is destabilized and alternatively degraded post-pRNA synthesis (Wassarman and Saecker 2006). Although a down-regulation of the pRNA depending on the NTP concentration is suggested, many questions concerning the potential cellular function of this pRNA still need to be answered.
The function of the 6S RNA has been studied in the intracellular pathogens L. pneumophila, S. typhimurium and Coxiella burnetii (Ortega, Gonzalo-Asensio and Garcia-del Portillo 2012; Ortega et al.2014; Warrier et al.2014). In L. pneumophila the exact role of the 6S RNA (ssrS) is not known but it has been shown that it is important for intracellular multiplication in human macrophages and in Acanthamoeba castellanii but not for growth in laboratory media (Table 1). In contrast to the above-discussed function of the 6S RNA as a repressor of the transcription of genes during stationary phase of growth through the binding to the σ70-containing RNA polymerase, the 6S RNA of L. pneumophila serves mainly as a positive regulator. Several genes including those involved in stress adaptation, amino acid metabolism and genes encoding Dot/Icm effectors, important for Legionella's ability to survive in the host, were identified (Faucher et al.2010). This different regulatory mechanism of the L. pneumophila 6S RNA could be due to an only weak binding to the RNA polymerase, adding another regulatory level to the current knowledge of the regulatory mechanism by which 6S RNAs function (Faucher et al.2010). Interestingly, a second 6S RNA copy, named 6S2 RNA, was reported to be present in L. pneumophila strain Philadelphia (Weissenmayer et al.2011). According to their results these two 6S RNAs are expressed at different stages during the biphasic life cycle of L. pneumophila (Weissenmayer et al.2011). However, this second copy does not seem to be present in L. pneumophila strain Paris (Cazalet et al.2004), indicating strain-specific differences (Cazalet et al.2004; Sahr, Rusniok, Dervins-Ravault et al.2012). Similarly, the 6S RNA homologues of S. enterica serovar Typhimurium and Coxiella burnetii were described as important for intracellular growth especially during stress conditions (Ortega, Gonzalo-Asensio and Garcia-del Portillo 2012; Ortega et al.2014; Warrier et al.2014). In C. burnettii the 6S RNA also interacts with the σ70-containing RNA polymerase, but it can serve as both a positive and a negative regulator during stationary phase growth (Table 1).
In conclusion, the different homologues of 6S RNA have multiple regulatory functions in intracellular pathogens with respect to both the genes they regulate and the mode of action, as they may induce or repress gene expression. Further investigations are necessary to determine when it acts as a negative or positive regulator and how this different mechanism is achieved.
The Csr/Rsm network and its interacting sRNAs
One of the best described RNA-binding proteins is the CsrA/RsmA (Carbon storage regulator/Regulator of secondary metabolism) ribonucleoprotein complex which controls different metabolic pathways and the repression of a variety of stationary-phase genes, including virulence-linked traits in pathogenic bacteria. CsrA was identified first in E. coli and defined as a carbon storage regulator system as the mutant strain of the csrA gene exhibited increased levels of glycogen compared with the wild-type strain (Romeo et al.1993). CsrA is a global bacterial regulator, as many homologous highly conserved proteins have now been identified in numerous bacteria but not in eukaryotes or Achaea. CsrA is a homodimer, in which each subunit is composed of five β-strands, one α-helix and an unstructured C-terminal part (Gutierrez et al.2005; Rife et al.2005). The major RNA-binding site is predicted to be the GxxG motif between strands β3 and β4, but other structures such as the β4 strand, the region between β2 and β3 and several conserved surface-exposed residues are also most probably involved in the interaction. This RNA-binding site allows CsrA to recognize specific A(N)GGA motifs in the RNA sequence, apparently with much higher affinity if these motifs are represented in the loop of a hairpin structure (Duss et al.2014). Typically, the SD sequence in the RNA leader region is rich in GA motifs; therefore, it is assumed that CsrA is predominantly regulating translation initiation by inhibiting the ribosome binding and furthermore favouring the degradation of the transcript (Seyll and Van Melderen 2013) (Fig. 4). However, there is also evidence that CsrA interactions influence the fate of the RNA not only by blocking translation but also in various other ways, such as stabilization of the RNA by protecting it from RNase degradation, affecting transcription elongation/termination or also as a positive regulator of translation by forming alternative RNA structures which even support ribosome binding (Liu, Yang and Romeo 1995; Figueroa-Bossi et al.2014). Interestingly, the regulation of the intracellular level of CsrA itself is also controlled predominantly at the post-transcriptional level, in particular by two or more sRNAs that efficiently bind and sequester the CsrA protein (Romeo 1998; Babitzke and Romeo 2007). These specific sRNAs, named CsrB/C or RsmY/Z in different bacteria, are composed of several repetitive sequence elements containing numerous conserved A(N)GGA motifs, most of which are located in hairpin loops (Table 1). Hence, almost the entire sRNA becomes covered with protein, making them a highly efficient antagonist to the interaction between CsrA and its target RNAs. Thus, the transcript level of these specific, small, untranslated RNAs is the key determinant of CsrA activity in the cell and already their moderate increase in expression can dramatically influence the equilibrium between target RNA- or sRNA-bound CsrA (Fig. 4).
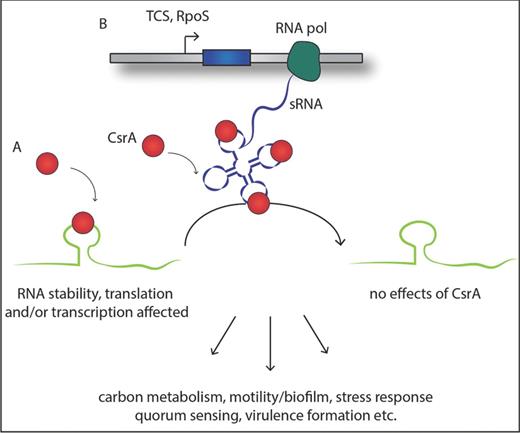
Simplified schematics of the general function of the Csr system. (A) The dimeric RNA-binding protein CsrA interacts with one or more A(N)GGA motifs of the target RNA, typically represented in a hairpin loop structure, leading to an alteration of the accessibility of the translation machinery, transcription (anti-)termination and/or the stability of the RNA. (B) Production of regulatory sRNA under the control of a two-component system and the alternative sigma-factor RpoS antagonizes CsrA function by binding CsrA with high affinity and hence abolishing the interaction with their target RNAs. This results in a global metabolic shift within the pathogen and amongst others to the activation of virulence-related proteins.
In all Csr/Rsm-regulatory networks known to date, the transcription of the small RNAs sequestering CsrA is regulated by a two-component system (TCS) that is homologous to the BarA/UvrY system in E. coli, and at least partially also by the alternative stress sigma factor RpoS (Romeo, Vakulskas and Babitzke 2013). This cascade of TCS/sRNA/CsrA is involved in the regulation of numerous cellular functions including carbon metabolism, motility, biofilm formation and adaptation to environmental stress responses, and production of virulence traits in the pathogen (Lucchetti-Miganeh et al.2008; Timmermans and Van Melderen 2010; Romeo, Vakulskas and Babitzke 2013) (Fig. 4). Even though the overall processes of CsrA regulation and its influence on regulation of the stationary phase and virulence-related pathways are better and better understood, not many direct target transcripts of CsrA, except its two regulatory sRNAs, have been identified to date in pathogenic bacteria. Most of the studies concentrated so far on indirect effects due to the loss of CsrA expression or overexpression of CsrA but rarely on physical interaction between the protein and the RNA.
The Csr/Rsm system is implicated in regulation of growth and motility
Among intracellular bacteria, the Csr-type regulatory system is well characterized in L. pneumophila and S. enterica serovar Typhimurium (Altier, Suyemoto and Lawhon 2000; Fettes et al.2001; Molofsky and Swanson 2003; Jonas et al.2008; Rasis and Segal 2009; Sahr et al.2009; Jonas et al.2010; Edwards et al.2011; Knudsen et al.2014; Nevo et al.2014). The Csr system is very versatile, as even though the regulatory impact observed for Legionella and Salmonella is similar to what is known for other pathogens such as Pseudomonas, Yersinia, Vibrio or E. coli, there are also striking differences. One commonly observed feature of the CsrA cascade is its strong effect on the cell morphology of the bacteria. Due to the loss of the csrA gene, the cells are converted during replication into smaller sized cells with coccoid shape instead of the typical rod-shaped structure of Salmonella and Legionella, while overexpression of CsrA resulted in highly elongated cells. Furthermore, mutation in csrA causes a drastic reduction in growth rate in particular at lower temperature (Forsbach-Birk et al.2004; Knudsen et al.2014). Also the transition from a sessile to a motile life form of S. enterica serovar Typhimurium is strongly affected by CsrA (Jonas et al.2010). In S. enterica serovar Typhimurium CsrA seems to act positively on swarming motility and it is required for accurate flagella expression by stabilizing the flhDC and the fliA mRNAs, which are the regulators of the flagella operon. This stabilizing effect leads to an increased production of flagellar proteins (Jonas et al.2010). In Legionella, CsrA also affects the flagella sigma factor FliA, but in contrast to S. enterica serovar Typhimurium, overexpression of CsrA in L. pneumophila resulted in lower fliA transcription and subsequently to reduced levels of FlaA, the major structural flagellar protein controlled by FliA (Forsbach-Birk et al.2004; Bruggemann et al.2006). Additionally, the knockout mutation of the TCS LetA/LetS, which is necessary for the transcription of the CsrA antagonists RsmY/RsmZ, completely abolishes motility in the post-exponential phase (Molofsky and Swanson 2003; Rasis and Segal 2009; Sahr et al.2009). Taken together, although CsrA is apparently a common regulator for flagella expression in different bacteria, the regulatory function differs significantly between them, as it can be either positive or negative.
The Csr/Rsm system is implicated in regulation of virulence and stress response
The control of stress responses and expression of virulence traits by the Csr system is a common feature among pathogens (Lucchetti-Miganeh et al.2008). The drastic changes in morphology and motility are closely related to the expression of genes which are important during stress response, such as heat/cold shock, acid resistance, osmotic stress and iron starvation. For example, in L. pneumophila, the inhibition of CsrA expression plays a major role during the transition from the replicative to transmissive phase, which is associated with adaptation to environmental stress and virulence formation (Molofsky and Swanson 2004; Sahr et al.2009). This finding was also supported by the recent identification of 26 effectors under the control of the LetA–RsmYZ–CsrA cascade (Nevo, Zusman, Rasis, et al.2014). These effectors were identified by comparing the expression levels of several effector-encoding genes in the wild type and a letA knockout mutant strain, and selecting those that showed a strong reduction of the expression level in the mutant strain. Then the presence of a putative CsrA-binding site was searched for by using the consensus sequence A(N)GGA. To confirm the regulation by CsrA, mutation of these putative CsrA-binding sites was performed. Indeed, during the exponential phase elevated expression levels of 22 of the 26 tested effector genes were observed. In contrast, in the wild-type strain, these effectors were more highly expressed in the stationary phase, suggesting that they might participate in the early steps of a new infection cycle most probably during the establishment of the Legionella-containing vacuole (LCV). Indeed, at least 19 of these 26 L. pneumophila effectors under the control of CsrA are involved in the modulation of endoplasmic reticulum (ER)-Golgi vesicular trafficking in S. cerevisiae (Nevo et al.2014). The effectors RalF, YlfA and VipA had already been shown to cause growth defects in S. cerevisiae and to affect vesicular trafficking in the host cell directly (Shohdy et al.2005; Heidtman et al.2009). Nevu and colleagues proposed that these effectors are probably the first to translocate into the host cell when L. pneumophila begins a new infection cycle and they then participate in the establishment of the LCV, by modulating different components of the ER–Golgi trafficking pathway. The finding that secreted effectors important for forming the LCV are under the control of CsrA further highlights the important role of this RNA-binding protein in survival and replication of intracellular bacteria.
In Salmonella, virulence gene expression is also under the control of CsrA as it binds near to the SD sequence of the hilD mRNA. HilD is the master regulator of the expression of virulence genes located on the SPIs. This negative regulation of HilD translation results in an overall down-regulation of the two pathogenicity islands SPI-1 and SPI-2, both required for intestinal infection in animal models (Martinez et al.2011). This effect is abolished by the activation of the SirA/BarA two-component system, resulting in a high level of transcription of the sRNAs CsrB/CsrC that sequester the CsrA binding to the hilD untranslated region (Table 1).
Given that CsrA is a very important post-transcriptional regulator, several attempts have been made to develop bioinformatics approaches to identify the RNAs that CsrA is targeting. A sequence-based approach to predict genes directly regulated by CsrA, CSRA_TARGET, was published recently (Kulkarni et al.2014). This computational algorithm predicted >100 mRNA targets in E. coli, S. enterica serovar Typhimurium, P. aeruginosa and L. pneumophila. In addition to new targets, already known and confirmed CsrA targets of these pathogens were identified, giving high confidence in its predictions. Furthermore, some predicted targets were tested and confirmed experimentally (Kulkarni et al.2014). The predicted CsrA targets indicated the important roles of CsrA in diverse processes such as stress response, quorum sensing and virulence factor regulation or metabolism. Also CsrA targets specific for intracellular pathogens, such as, for example, hilD, encoding the master regulator for the induction of invasion genes located on SPI-1 or sipA encoding a type III effector protein of S. enterica serovar Typhimurium necessary to induce a proinflammatory response in epithelial cells, were identified (Kulkarni et al.2014).
Taken together, the Csr-type regulatory system is important for the expression of the virulence phenotype of intracellular bacteria by controlling a large variety of physiological and stress responses that are implicated directly or indirectly in host cell infection and the overall biological fitness during changing environmental conditions. However, although very similar cellular functions are regulated by this post-transcriptional control mechanism in many pathogens, the impact of the regulation, whether inducing or inhibiting, differs considerably between different bacterial species.
THE CRISPR/CAS SYSTEM AND THE IMPLICATION OF crRNAs IN VIRULENCE
Recently, an adaptive microbial immune system, named clustered regularly interspaced short palindromic repeats (CRISPR), has been identified which provides acquired immunity against viruses and plasmids in bacteria and Archaea (Barrangou et al.2007). Unlike the restriction-based defence mechanism, the CRISPR/Cas system is highly specific, adaptive and heritable. It consists of an array of conserved short DNA repeat sequences, which are interspaced by variable spacer regions, originating from foreign DNA. Additionally, several cas (CRISPR-associated) genes are located in close proximity to the repeat–spacer array. The composition of the CRISPR/Cas locus can vary greatly between different microbial species and accordingly can be divided into types I–III. However, the basic mechanism to mediate adaptive immunity is common to all types of CRISPR/Cas systems and is composed of three main steps: the acquisition of foreign DNA, the processing and crRNA generation and the interference or silencing (for a review, see Sorek, Lawrence and Wiedenheft 2013) (Fig. 2). Thus CRISPR/Cas is a DNA-encoded, RNA-mediated defence system that provides sequence-specific recognition, targeting and degradation of exogenous nucleic acids. Going into the details of functionality and mechanistic differences between species would exceed the scope of this review, thus we place our main focus here on a newly described role for the CRISPR/Cas systems during infection and virulence formation of pathogens.
In a model it was proposed that the pathogenic potential of bacteria is associated with or even controlled by bacteriophage infections as CRISPR activity might interfere with the uptake of foreign DNA potentially carrying virulence traits, such as genes coding for toxins or antibiotic resistance (Mojica et al.2005). Furthermore, it was found that the spacer regions contain sequence homologous not only to foreign DNA but also, even if in minor quantity, to endogenous chromosomal regions of the bacteria itself. This self-targeting was proposed to lead to a form of autoimmunity, suggesting a role in regulation of endogenous gene expression (Stern et al.2010). Furthermore, changes in cas gene expression seem to be correlated with stress response, e.g. observed in L. pneumophila where an induction of the cas operon is dependent on the growth phase and typically up-regulated in the transmissive phase (Sahr et al.2009). This phenomenon is abolished in a ΔletA and a ΔrpoS knockout mutant, suggesting a connection with the stringent response. Assuming an increased transcript level of RNAs in conditions relevant for their biological role, it suggests that CRISPR/Cas might have relevance for Legionella virulence formation. Indeed, similar results were observed in vivo during intracellular growth in macrophages and amoeba. Furthermore, cas2 mutants were significantly impaired during intracellular growth in amoebae, indicating a pivotal role for the CRISPR/Cas system during amoeba infection (Gunderson and Cianciotto 2013) (Table 1). Also in Listeria, the rliB locus composed of five small copies of expressed CRISPR repeats was shown to be involved in virulence (Toledo-Arana et al.2009). Interestingly, in L. monocytogenes, RliB–CRISPR is not related to an adjacent cas operon but is instead expressed and processed by the PNPase that is needed for RliB–CRISPR-mediated DNA interference (Sesto et al.2014) (Table 1).
In both examples, a strong correlation between CRISPR expression and pathogenicity is evident. However, the functionality and the role are not yet established. CRISPR/Cas can promote bacterial fitness through protection against bacteriophages and thus increase indirectly the survival rate and ability to infect host cells. Alternatively, it might have a direct influence by regulating endogenous virulence factors and effectors similar to a mechanism identified recently in Francisella novicida (Sampson et al.2013). Therein it was shown for the first time that the Cas9 complex of this intracellular pathogen directly represses the production of an immunogenic lipoprotein, BLP, by stimulating its mRNA degradation. The BLP protein is recognized by the Toll-like receptor 2, triggering the antibacterial proinflammatory immune response. Therefore, Cas9-dependent repression of Blp production is essential for innate immune evasion and intracellular survival of F. novicida during infection of eukaryotic host cells. This process involves additionally two other newly described small RNAs that mediate the sequence-specific recognition of the blp transcript (Sampson et al.2013). These two sRNAs, named trans-activating crRNA (tracrRNA) and CRISPR/Cas-associated RNA (scaRNA), are not part of the crRNA cluster but closely related to the CRISPR/Cas region (Table 1). The knockout mutants of either one of them were not capable of establishing infection in a mouse model.
This outstanding observation demonstrates that the CRISPR/Cas system can also have an endogenous function independent from classical bacterial defence via an antisense RNA silencing mechanism. As CRISPR/Cas is widespread in bacteria, a similar mechanism might exist in numerous other pathogens, suggesting that this system might have a more general involvement in gene regulation, particularly during stress response and virulence formation. Unravelling this phenomenon and a deeper knowledge of the functionality of the CRISPR/Cas system will lead to a better understanding of gene regulation and virulence formation in pathogens and possibly to a new chance to fight bacterial infection independently of preceding antibiotic multiresistance.
CONCLUDING REMARKS
Several decades of research revealed the high variability, wide distribution and diverse functionality of sRNAs and demonstrated their crucial role in many biological processes such as in environmental sensing and stress adaptation, virulence and infectivity of intracellular bacteria, as well as development and metabolism. Here we aimed to highlight some of the most striking aspects that make sRNAs such versatile regulators in bacteria in particular for intracellular bacteria during the interaction, infection and growth within their eukaryotic host cells. However, considering the outstanding plethora and variety of sRNA regulatory elements, one may speculate about their functional redundancy, mainly as a consequence of their nature, within pathogenic and non-pathogenic bacterial species. In this context we highlighted several and very diverse sRNAs that can act independently or simultaneously to ensure the bacteria a favourable adaptation to adverse conditions and a benefit during the infection of the host cells (Fig. 5). On the other hand, only little is known about the opposite scenario, that one single sRNA may combine multiple regulatory mechanisms. Excitingly, it was recently shown that the same sRNA might indeed have several functions. The sRNA Qrr is able to fulfil four distinct regulatory mechanisms to fine-tune the regulation of quorum sensing in Vibrio (Feng et al.2015). Qrr can act as a negative control element to repress its targets through sequestration, catalytic degradation or coupled degradation, and at the same time as a positive regulatory element by setting free the RBS, allowing the target expression to occur while promoting its own degradation. The four different mechanisms are the results of diverse base-pairing strategies, employed by the Qrr sRNA to target defined quorum-sensing mRNAs (Feng et al.2015). Thus, this new view of multifunctional sRNA may be employed by other bacterial pathogens, representing more than a mere peculiarity but a common role not yet thoroughly examined. Recently it has been shown that sRNAs of bacteria can have an interspecies role, as two sRNAs, OxyS and DsrA of E. coli, have been reported to have physiological effects on Caenorhabditis elegans via regulating its gene expression (Liu et al.2012), and that plant microRNAs in the food of animals could have interspecies roles in mammalian cells (Zhang et al.2012). It also has been shown that L. monocytogenes releases nucleic acids and that such secreted bacterial RNA/DNA is recognized by cytosolic sensors such as RIG-I, MDA5 and STING, leading to the triggering of interferon-β production (Abdullah et al.2012). However, until now the main virulence factors identified, which are secreted into the host cell by intracellular bacteria, are proteins. Thus another current challenge might be to discover whether specific sRNAs of intracellular bacteria might play a role in the host cells they infect. Taking into account the huge flexibility and evolutionary variety of sRNA within pathogenic bacteria one can imagine that bacterial sRNAs may also be secreted during infection to manipulate host cell function. In particular these bacterial sRNAs might mimic eukaryotic miRNAs to subvert their functions in the cells they infect. The identification of sRNAs secreted or released into the host cell to subvert its functions may emerge as a new, exciting topic of research in host–pathogen interactions. Thus, even though the analyses of bacterial RNAs is an ever-growing field of study, many questions still remain unanswered and new regulatory mechanisms might be discovered allowing us to appreciate more and more the diversified world of sRNA and its regulatory potential.
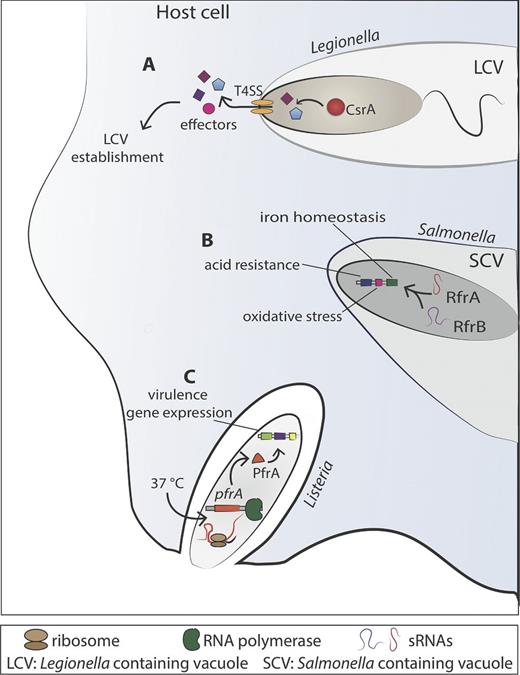
Examples of RNA-mediated regulation implicated in adaptation to the host environment in intracellular bacteria. (A) The regulatory cascade comprising CsrA and two sRNAs (RsmY and RsmZ) regulates the expression of secreted effectors of L. pneumophila that are released into the host cell cytosol. These effectors manipulate different processes of the secretory pathway to help in establishing the Legionella-containing vacuole (LVC). (B) The trans-encoded regulatory sRNAs RfrA and RfrB of Salmonella enterica serovar Typhi play a central role in iron homeostasis regulation, and are implicated in protecting the bacteria against oxidative stress, bactericidal antibiotics and acid resistance when replicating in the host cell. (C) Listeria monocytogenes virulence genes are under the control or PrfA, the master regulator of virulence gene expression. A thermosensor present in the 5′ UTR of prfA senses the variation of the temperature when L. monocytogenes changes from the extracellular environment (lower temperature) to invade a mammalian host cell (37°C). The increase in the temperature changes its conformation, freeing the ribosome-binding site, which is in turn allows the translation of PrfA and subsequent virulence gene expression necessary for invasion of eukaryotic cells and the rapid escape from the vacuole to the cytosol.
Work in the laboratory of CB is financed by the Institut Pasteur, the Institut Carnot-Pasteur MI, the French Region Ile de France (DIM Malinf), grant no. ANR-10-LABX-62-IBEID, the Fondation pour la Recherche Médicale (FRM), grant no. DEQ20120323697, and the Pasteur–Weizmann consortium ‘The roles of non-coding RNAs in regulation of microbial life styles and virulence’. GO was supported by a stipend from the Pasteur-Paris University (PPU) International PhD Program.
Conflict of interest. None declared.
REFERENCES